DOI:
10.1039/D1RA09139E
(Paper)
RSC Adv., 2022,
12, 4029-4041
Hexadecyl trimethyl ammonium bromide assisted growth of NiCo2O4@reduced graphene oxide/ nickel foam nanoneedle arrays with enhanced performance for supercapacitor electrodes†
Received
17th December 2021
, Accepted 19th January 2022
First published on 31st January 2022
Abstract
NiCo2O4@reduced graphene oxide (rGO)/nickel foam (NF) composites were prepared via a hydrothermal method followed by annealing assisted by hexadecyl trimethyl ammonium bromide (CTAB). NiCo2O4@rGO/NF nanoneedle arrays grew directly on Ni foam (NF) without using a binder. The effect of graphene oxide (GO) concentration on the electrochemical properties of the composite was studied. When the GO concentration was 5 mg L−1, the as-prepared NiCo2O4@rGO/NF reaches the highest specific capacitance of 1644 F g−1 at a current density of 1 A g−1. Even at 15 A g−1, the specific capacitance is still 1167 F g−1 and the capacitance retention rate is 89% after 10
000 cycles at 10 A g−1. Furthermore, a NiCo2O4@rGO/NF//graphene hydrogel (GH) asymmetric supercapacitor cell (ASC) device was assembled and exhibits a high specific capacitance of 84.13 F g−1 at 1 A g−1 and excellent cycle stability (113% capacitance retention) after 10
000 charge/discharge cycles at 10 A g−1. This provides potential for application in the field of supercapacitors due to the outstanding specific capacitance, rate performance and cycle stability of NiCo2O4@rGO/NF.
1. Introduction
With the aggravation of global warming and the exhaustion of fossil energy, the development and application of new energy materials are extremely urgent. On the one hand, new energy industries such as solar energy, wind energy and tidal power have promising prospects, but these energy sources have certain limitations in terms of time or space. In order to completely replace the original thermal power generation, matching energy storage systems are needed to achieve the continuous and stable output of electric energy. On the other hand, new energy vehicles represented by electric vehicles will replace traditional fuel vehicles to become the mainstream of transportation in the future, which will be a revolution in the world automobile industry and the inevitable result of low-carbon economic development. The key to overcome fuel vehicles is to develop efficient energy storage devices with high energy density and power density, long cycle life and good safety. In addition, with the continuous progress of technology and the acceleration of informatization, there is an increasingly urgent demand for high-performance energy storage components in the military, industry, communication and other fields.
As efficient, environmentally friendly and new-type energy storage elements, supercapacitors have many advantages of both traditional capacitors and batteries, such as large capacitance, high energy density and power density, wide operating temperature range, long cycle life,1,2 etc. They have attracted great attention in the world and become one of the important advanced technologies in the energy field. Electrode materials are the main component of supercapacitors and the key index to determine their performance. The research on the preparation and performance of electrode materials has always been the focus of the field of supercapacitors. Double-layer capacitor electrode materials represented by carbon materials3,4 and pseudocapacitance electrode materials represented by transition metal oxides/hydroxides5–7 and conductive polymers8,9 have their own advantages, and considerable progress has been made in related researches. Among numerous transition metal oxides, NiCo2O4 is a kind of mixed metal oxide with low price and abundant resource.10,11 NiCo2O4 is spinel structure, which can be considered to be formed by replacing Co with Ni in Co3O4. There are Co3+/Co4+, Ni2+/Ni3+ and Co2+/Co3+ redox reaction pairs in the charge–discharge process. Therefore, NiCo2O4 has higher electrochemical activity than pure nickel or cobalt oxides, and its electronic conductivity (10−1 to 102 S cm−1) is better than Co3O4 and NiO.12,13 Based on this, NiCo2O4 has become the research hotspot of electrode materials for supercapacitors in recent years. However, its poor cycle stability and rate performance limit its application in practice. In order to improve the electrochemical properties of NiCo2O4 materials, a lot of researches have been carried out.
The construction of special complex nanostructures is an effective strategy. Special nanostructures have significant effects on the properties of materials. Numerous NiCo2O4 materials with various morphologies have been designed and synthesized, including nanosheets,14,15 nanowires,16,17 nanorods,11,18 microspheres,19,20 hexahedrons,21 etc. These morphologies have their own advantages, but also have their limitations. One-dimensional nanostructures such as nanowires are beneficial to the assembly of nanodevices, but their own specific surface area is small and they cannot provide enough active sites in the reaction process. Two-dimensional nanostructures such as nanosheets have a large specific surface area, which can provide more electron transport channels and make full use of the active materials. However, nanosheets tend to form layered stacks, and their stability will decline after several charge–discharge cycles. Compared with simple structures, complex stereoscopic cross structures, such as core–shell structure22 and yolk–shell structure23 have more advantages in preventing internal particle agglomeration, providing more active sites for electrochemical reactions and effectively shortening ion diffusion paths. At the same time, the space between shells can buffer the residual pressure caused by volume expansion/contraction during the repeated Faraday reaction.
Besides, NiCo2O4 compounds with different materials can combine the advantages of various materials and produce synergistic effect, which is expected to obtain more outstanding electrochemical performance. Most of the researches were focused on loading transition metal oxides such as MnO2,24 Ni(OH)2 (ref. 13) on the surface of NiCo2O4, or loading NiCo2O4 on porous materials with large surface area and high conductivity, such as nickel foam (NF)25 and carbon materials,26 etc. Among them, graphene as an infinitely scalable two-dimensional crystal material, which has a large specific surface area (2630 m2 g−1), good electronic conductivity and chemical stability,27 has been widely used in the preparation of composite electrode materials for supercapacitors since it was successfully isolated from graphite by Geim group in 2004.28 In addition, one-dimensional carbon nanotubes (CNTs) are also commonly used in the preparation of composite materials.29
By integrating the above two approaches, the special composite microstructure constructed by integrating several materials with different structures is considered to be a more effective method to obtain excellent electrode materials for supercapacitors due to its good synergistic effect. Zhang et al.30 synthesized reduced graphene oxide (rGO)/NiCo2O4 composites, in which the rGO was coated on the NiCo2O4 nanowires with no aggregation. The unique heterostructured rGO/NiCo2O4 nanostructures exhibit high specific capacitance, excellent cycling stability and good rate capability in the application for supercapactiors, resulting from the interconnected porous frameworks and the strong interface polarization. Yang et al.31 reported mesopores NiCo2O4 nano-needles directly grown on nickel foam for high-performance supercapacitors by one-step hydrothermal method. The unique structure of mesopores increased the contact efficiency between the active materials and the electrolyte, which made the electrolyte easily penetrate the electrode and achieved a high capacitance response to have effective storage application. Wei et al.32 developed a green pre-adjusted pH value aq. phase coprecipitation strategy assisted by citric acid, followed proper annealing at different temperature for the first time to synthesize novel honeycombed-like composites NiCo2O4/rGO. NiCo2O4/rGO-250 (at 250 °C) shows considerable specific capacitance, high rate performance and good cycling stability for supercapacitor, which could be attributed to the highly-ordered 3D honeycombed-like nanosheet array leading to high specific surface area and numerous open macroporous network giving sufficient electroactive sites. In brief, extensive researches revolved around the synthesis technology, morphology control, structural design and electrochemical performance improvement of NiCo2O4 composites.
In this work, a simple strategy was used to prepare NiCo2O4@rGO/NF composites assisted by hexadecyl trimethyl ammonium bromide (CTAB) via hydrothermal method followed annealing. The prepared NiCo2O4@rGO/NF was applied to supercapacitors and the electrochemical performance was evaluated. In the process, the effect of graphene oxide (GO) concentration on the electrochemical properties of the composite was also investigated. It is believable that the synthetic strategy, the morphological control and the application of NiCo2O4@rGO/NF electrode materials with enhanced performance in the field of supercapacitor could provide reference for researchers.
2. Experimental
2.1. Preparation of NiCo2O4@rGO/NF and NiCo2O4/NF
1 mmol of Ni(NO3)2·6H2O, 2 mmol of Co(NO3)2·6H2O and 4.5 mmol of urea were added into 20 mL of deionized water and stirred. Then 0.5 mmol of CTAB was added into the above solution with continuous stirring, and dispersed under ultrasonication for 30 min. GO was prepared by the modified Hummers' method.33 A certain mass of GO was weighed and added into 10 mL of deionized water to form uniform dispersion under ultrasonication with a range of concentrations (3, 4, 5 and 6 mg mL−1) for later use. All the chemical reagents are of analytical grade.
The above two solutions were mixed and stirred for 40 min, then transferred into a Teflon lined stainless steel autoclave, in which a piece of nickel foam (NF) (1 cm × 1 cm) was immersed, then reacted at 120 °C for 10 h. After cooling to room temperature, the nickel foam was rinsed with deionized water and ethanol. The product was dried at 60 °C for 12 h. Finally, NiCo2O4@rGO/NF composites were obtained after annealing at 300 °C for 2 h. Simultaneously, NiCo2O4/NF was synthesized by the same way, but in absence of rGO for comparison. The schematic synthesis procedure of the NiCo2O4@rGO/NF composites is illustrated in Fig. 1. In brief, firstly, NiCo2(OH)6 was formed in the hydrothermal reaction,34 and CTAB played a role of controlling the morphology of the products during the reaction.35 Then, NiCo2O4 was acquired after further heat treatment.
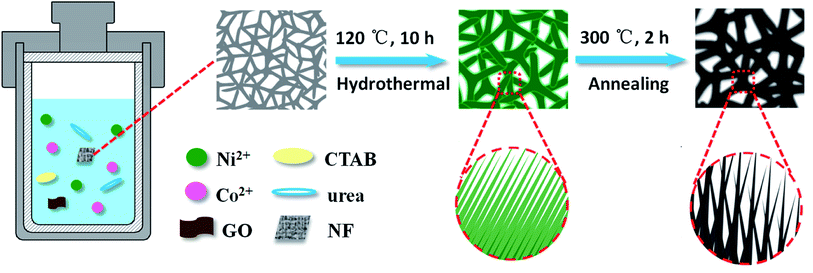 |
| Fig. 1 Schematic synthesis procedure of NiCo2O4@rGO/NF. | |
2.2. Characterization
The phase structures of the as-prepared materials were performed using the powder X-ray diffraction (XRD, D-max-2500/PC, Rigaku) with Cu Kα radiation (λ = 0.15406 nm) over a range 2θ = 10°–80°. The morphologies were characterized by scanning electron microscopy (SEM, KYKY-2800B) and transmission electron microscopy (TEM, Hitachi HT-7700). The elemental analysis was detected by X-ray photoelectron spectroscopy (XPS, Thermal ESCALAB 250).
2.3. Electrochemical measurements
The nickel foam loaded with active material was pressed under a pressure of 10 MPa, and then soaked in 6 M KOH solution for 24 h. The electrochemical performance was tested in a three-electrode system with 6 M KOH aqueous as the electrolyte. The as-prepared active material was used as the working electrode, platinum plate (1 cm × 1 cm) electrode as the counter electrode and Hg/HgO electrode as the reference electrode. Galvanostatic charge–discharge (GCD) tests were performed via a charge–discharge instrument (Neware CT-3008, Shenzhen, China) with a potential window of 0–0.45 V. Cyclic voltammetry (CV) and electrochemical impedance spectroscopy (EIS) were carried out on a CHI660E electrochemical workstation (Chenhua, Shanghai, China). The potential range of CV tests was from 0 V to 0.55 V, and the frequency range of EIS was from 10−2 Hz to 105 Hz with the amplitude of 5 mV.
3. Results and discussion
3.1. Structure and morphology
The XRD patterns of NiCo2O4@rGO/NF composites with different concentration of GO (3, 4, 5 and 6 mg mL−1) are shown in Fig. 2. The diffraction peaks of the composites obtained at different concentrations of GO are basically the same. It indicates that the amount of GO in the preparation of the composites had no effect on the phase of NiCo2O4. Three strong and sharp diffraction peaks are corresponded to nickel deriving from the foam nickel substrate. The diffraction peaks of 2θ at 36.7°, 44.6°, 59.1° and 64.9° can be indexed to NiCo2O4 (JCPDS 20-0781), corresponding to (311), (400), (511) and (440) crystal planes, respectively. There is no obvious diffraction peak of rGO observed, which probably because the characteristic peak of rGO was covered by three strong peaks of nickel.36 The chemical states of NiCo2O4@rGO/NF (GO 5 mg L−1) are characterized by XPS, as shown in Fig. 3, the region spectra of Ni 2p, Co 2p, O 1s and C 1s show characteristic peaks. The Ni 2p shows the peaks of Ni 2p3/2 and Ni 2p1/2 (Fig. 3(a)), both consisting of doublet peaks at 853.8, 855.4, 871.4 and 873.1 eV, corresponding to Ni2+ 2p3/2, Ni3+ 2p3/2, Ni2+ 2p1/2, and Ni3+ 2p1/2, respectively,37 which could imply the presence of both Ni2+ and Ni3+. Two fitting peaks corresponding to Co 2p3/2 and Co 2p1/2 were observed in the Co 2p spectrum (Fig. 3(b)), which are characteristic of Co2+ and Co3+. The doublet peaks at 779.65 and 781.1 eV correspond to Co3+ 2p3/2 and Co3+ 2p1/2 as well as the other twos at 794.85 and 796.65 eV correspond to Co2+ 2p3/2 and Co2+ 2p1/2.38 The spectrum of O 1s region (Fig. 3(c)) can be deconvolved into four peaks at 529.15, 529.75, 531.25 and 532.3 eV, represented as O1, O2, O3 and O4, which are assigned to metal–oxygen bonds in the lattice of NiCo2O4, the surface hydroxyl, defect sites and physical/chemical adsorbed water, respectively.39,40 The C 1s spectrum (Fig. 3(d)) displays three types carbon bonds, including sp2 C–C (284.8 eV), C–O (286.2 eV) and C
O (288.0 eV).41 It also can be seen from the peak intensity that the amount of oxygen-containing functional groups is less than that of C–C groups, indicating the successful reduction of GO to rGO.40
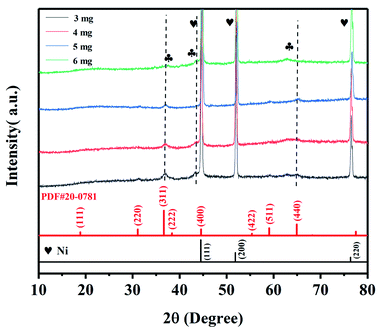 |
| Fig. 2 XRD patterns of NiCo2O4@rGO/NF with different concentration of GO. | |
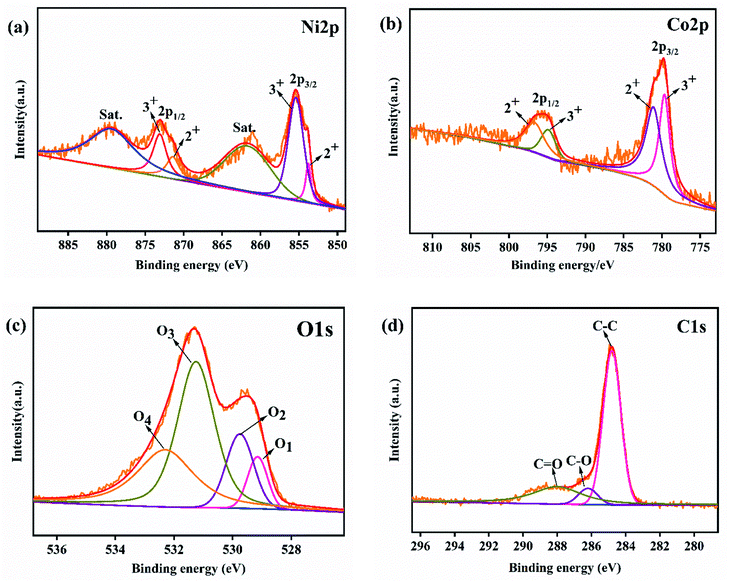 |
| Fig. 3 XPS spectra of (a) Ni 2p, (b) Co 2p, (c) O 1s and (d) C 1s of the NiCo2O4@rGO/NF (GO 5 mg L−1). | |
The morphologies and nanostructures of bare Ni foam, NiCo2O4/NF and NiCo2O4@rGO/NF with different concentrations of GO (3, 4, 5 and 6 mg L−1) are charactered by SEM and the images are shown in Fig. 4. As shown in Fig. 4(a and b), the surface of bare Ni foam is smooth. After hydrothermal reaction, double hydroxides precursor of NiCo2O4 was formed, which presents nanowire morphology (Fig. S1, ESI†). After heat treatment, when GO was in absence, the NF is completely covered by NiCo2O4 nanoneedle arrays, which are neatly oriented (Fig. 4(c and d)). When GO was added, the product of NiCo2O4@rGO still maintains nanoneedle morphology. However, it's important to point out that the nanoneedles crossed in different directions, as shown in Fig. 4(e–h). The difference in morphology is mainly due to the role of rGO. NF provided growth sites for NiCo2O4, and then nanoneedles' growth was prolonged and strongly isotropic. In the presence of rGO, rGO adsorbed to NF and then coated and separated NiCo2O4, which changed its growth direction and led to the anisotropy. As the concentration of GO increased, rGO cladding layers in the composites would be thickened. Fig. 5(a and b) show different magnified TEM images of NiCo2O4@rGO composites (GO 5 mg L−1), from which it could be clearly observed that NiCo2O4 nanoneedles are interlaced. From HRTEM images (Fig. 5(c and d)), it can be seen that a lattice spacing of 0.458 nm corresponding to the (111) crystal plane of NiCo2O4. The SEM energy dispersive spectroscopy (EDS) in Fig. 6(a) verifies the existence of C, O, Ni and Co elements. As can be seen from the SEM (Fig. 6(b)) mapping images in Fig. 6(c), Co, Ni, C, and O distribute homogeneously over the NiCo2O4@rGO architecture. It also proves the existence and distribution of rGO in the composites.
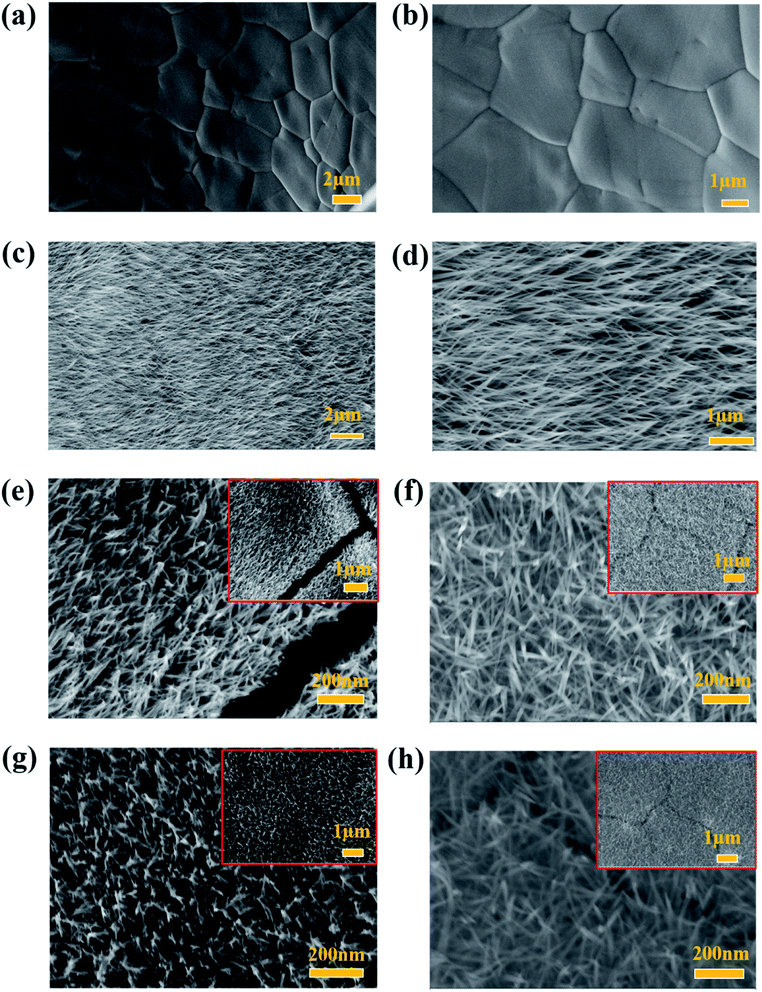 |
| Fig. 4 SEM images of (a and b) bare Ni foam (c and d) NiCo2O4/NF, (e) NiCo2O4@rGO/NF (GO 3 mg L−1), (f) NiCo2O4@rGO/NF (GO 4 mg L−1), (g) NiCo2O4@rGO/NF (GO 5 mg L−1) and (h) NiCo2O4@rGO/NF (GO 6 mg L−1). | |
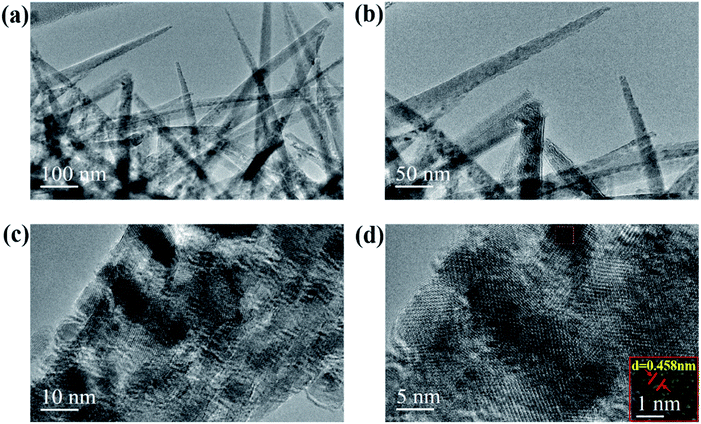 |
| Fig. 5 (a, b) TEM and (c, d) HRTEM images of NiCo2O4@rGO/NF (GO 5 mg L−1). | |
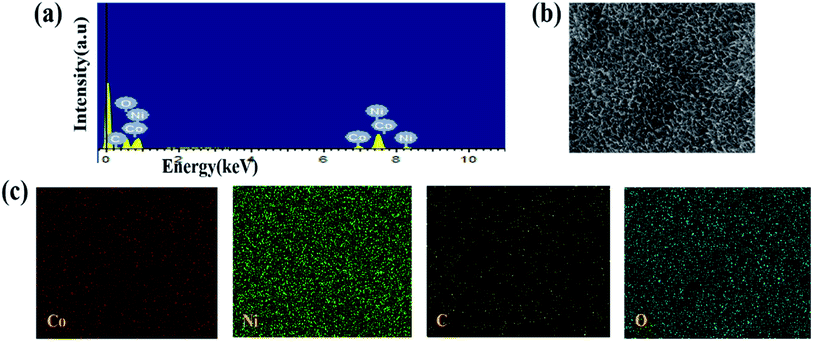 |
| Fig. 6 (a) SEM energy dispersive spectroscopy, (b) SEM and (c) SEM element mapping of NiCo2O4@rGO/NF (GO 5 mg L−1). | |
3.2. Electrochemical performance
Fig. 7 shows CV curves of NiCo2O4@rGO/NF with different concentration of GO (3, 4, 5, 6 mg L−1), which were carried out at the potential window from 0 to 0.55 V with the scan rate of 5, 10, 20, 30, 40 and 50 mV s−1. There is a pair of redox peaks can be observed on all CV curves (Fig. 7(a–d)), which resulted from redox reactions of Co3+/Co4+, Ni2+/Ni3+ and Co2+/Co3+, demonstrating the pseudocapacitive characteristic of NiCo2O4@rGO/NF composite.42 The reaction equations are as follows:43 |
NiCo2O4 + OH− + H2O ⇌ NiOOH + 2CoOOH + e−
| (1) |
|
CoOOH + OH− ⇌ CoO2 + H2O + e−
| (2) |
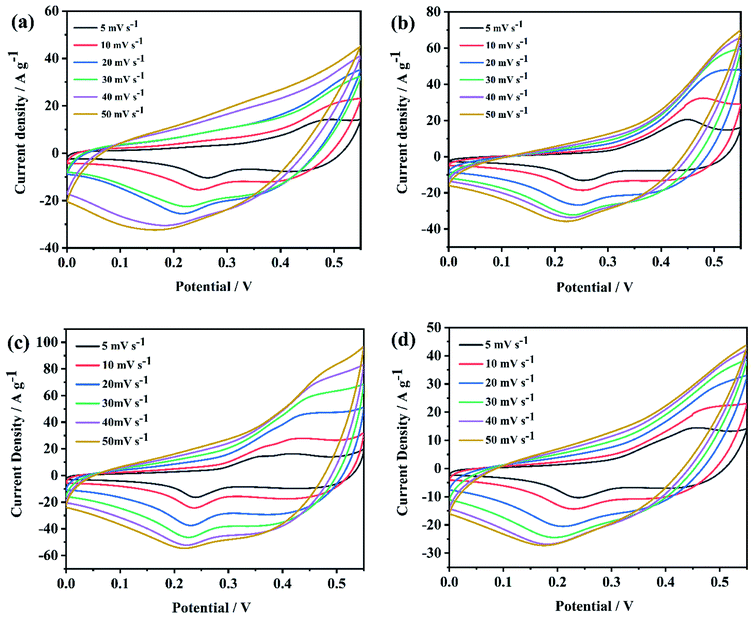 |
| Fig. 7 CV curves of NiCo2O4@rGO/NF with different concentration of GO at various scan rates: (a) NiCo2O4@rGO/NF (GO 3 mg L−1), (b) NiCo2O4@rGO/NF (GO 4 mg L−1), (c) NiCo2O4@rGO/NF (GO 5 mg L−1) and (d) NiCo2O4@rGO/NF (GO 6 mg L−1). | |
When the scan rate increased from 5 to 50 mV s−1, the shape of CV curves had little change, suggesting good electrochemical reversibility as supercapacitor electrodes. GCD curves of NiCo2O4@rGO/NF with different concentration of GO (3, 4, 5, 6 mg L−1) at the various current density of 1, 2, 3, 5, 10 and 15 A g−1 are displayed in Fig. 8. From (Fig. 8(a)–(d)) can we see, the charge–discharge curves are all nonlinear with evident voltage plateau, indicating typical pseudocapacitive behavior, which is consistent with the CV results. For a comparison, the electrochemical performance of NiCo2O4@rGO/NF composites with different concentration of GO were contrasted and depicted in Fig. 9. When the concentration of GO was 5 mg L−1, the closed area of CV curve is the largest (Fig. 9(a)) and the GCD curve has the longest discharge time (Fig. 9(b)). The specific capacitance calculated by the GCD curve is 1044, 1504, 1644 and 1322 F g−1, corresponding to GO concentration of 3, 4, 5 and 6 mg L−1, respectively. At all the current densities, NiCo2O4@rGO/NF (GO 5 mg L−1) has the largest specific capacitance compared with the samples of the other three concentrations. Moreover, its specific capacitance varies most gently with the current density, suggesting good rate performance (Fig. 9(c)). It is probably because that as the concentration of GO increased, the proportion of rGO in NiCo2O4@rGO/NF composite increased, and rGO plays a role in connecting NiCo2O4 nanoneedles to improve the electrical conductivity of the composite. However, due to the low specific capacitance of rGO itself, too much rGO will affect the overall specific capacitance of the composite. Nyquist plots of EIS for NiCo2O4@rGO/NF composites with various concentrations (3, 4, 5 and 6 mg L−1) are shown in Fig. 9(d). The line at low frequency region of NiCo2O4@rGO/NF (GO 5 mg L−1) is more vertical to the real axis (Z′), indicating more ideal capacitance behavior.44
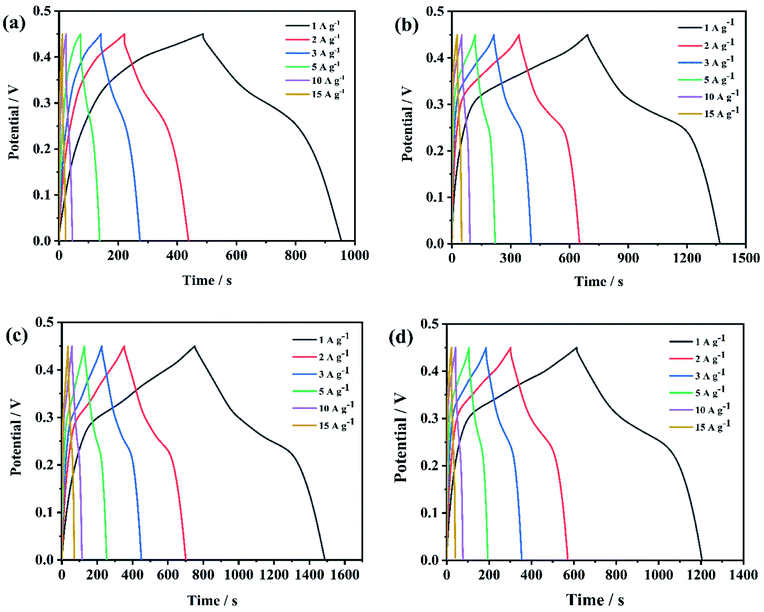 |
| Fig. 8 GCD curves of NiCo2O4@rGO/NF with different concentration of GO at various current densities: (a) NiCo2O4@rGO/NF (GO 3 mg L−1), (b) NiCo2O4@rGO/NF (GO 4 mg L−1), (c) NiCo2O4@rGO/NF (GO 5 mg L−1) and (d) NiCo2O4@rGO/NF (GO 6 mg L−1). | |
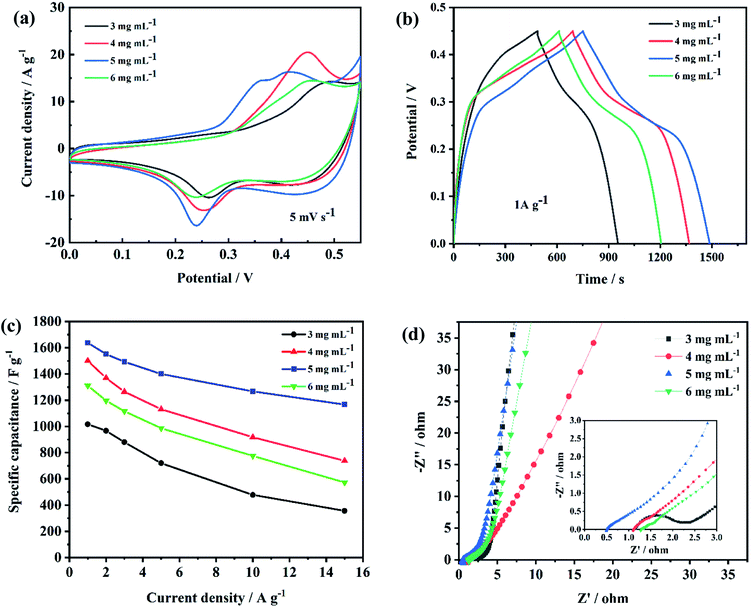 |
| Fig. 9 Comparison of electrochemical performance of NiCo2O4@rGO/NF with different concentration of GO: (a) CV curves (5 mV s−1), (b) GCD curves (1 A g−1), (c) specific capacitances at various current densities and (d) Nyquist plots of EIS. | |
Fig. 10 describes the comparison of electrochemical properties of NiCo2O4@rGO/NF and NiCo2O4/NF. As shown in Fig. 10(a), the CV curves of NiCo2O4@rGO/NF and NiCo2O4/NF at the scan rate of 50 mV s−1, NiCo2O4@rGO/NF has larger closed area than NiCo2O4/NF, demonstrating higher specific capacitance. It is confirmed by GCD tests, displayed in Fig. 10(b). At the current density of 1 A g−1, the specific capacitance of NiCo2O4/NF and NiCo2O4@rGO/NF is 582.2 and 1644 F g−1, respectively. Obviously, it is nearly twice higher the composite with rGO than that without rGO. Fig. 10(c) shows the specific capacitance of these two composites changing with the current density. The calculated specific capacitance of NiCo2O4@rGO/NF is 1644, 1551, 1493, 1400, 1267 and 1167 F g−1 at the current density of 1, 2, 3, 5, 10 and 15 A g−1. Even at 15 A g−1, 71% of the specific capacitance (1 A g−1) has still been retained, while the specific capacitance retention of NiCo2O4/NF is 61%. It can be seen that NiCo2O4@rGO/NF has better rate performance. Nyquist plots of EIS for NiCo2O4@rGO/NF and NiCo2O4/NF are shown in Fig. 10(d). The intercept at the real axis represents the equivalent series resistance (Rs), containing the electrolyte resistance, the intrinsic resistance and the contact resistance. The diameter of the semicircle at the high frequency is associated with the charge-transfer resistance (Rct). For NiCo2O4@rGO/NF and NiCo2O4/NF electrodes, the Rs values are 0.679 and 0.501 Ω, respectively, indicating that a lower solution resistance of NiCo2O4@rGO/NF than that of NiCo2O4/NF. Likewise, NiCo2O4@rGO/NF has a smaller semicircle, which could provide a fast pathway for ion transfer and electron transport. The cycle stability of the two electrodes after 10
000 cycles was tested at a current density of 10 A g−1. As shown in Fig. 10(e), it is obvious that the specific capacitance of NiCo2O4@rGO/NF composite is much higher than that of NiCo2O4/NF. Additionally, NiCo2O4@rGO/NF can still maintain 89% of the initial specific capacitance after 10
000 charge–discharge cycles, exhibiting more excellent cycle stability than NiCo2O4/NF. The electrochemical properties of NiCo2O4 and its composite materials reported in some literatures are listed in Table 1. It can be seen from the literature comparison, the NiCo2O4@rGO/NF composite prepared in this work is comparable. In brief, the superiority of electrochemical performance of NiCo2O4@rGO/NF composite is mainly analyzed for the following reasons: (i) the anisotropic nanoneedles formed a cross network, which could provide an effective channel for ion/electron diffusion. (ii) NiCo2O4 grows directly on NF matrix, and rGO plays a bridge among the NiCo2O4 nanoneedles, together with no binder is used, which have effectively improve the conductivity of the composite, due to the excellent electrical conductivity of NF and rGO. Furthermore, it also plays a positive role in the full use of active materials. (iii) The outstanding mechanical property inhibits the deformation caused by swelling/shrinking of the electrode material during the repeated charge–discharge process.
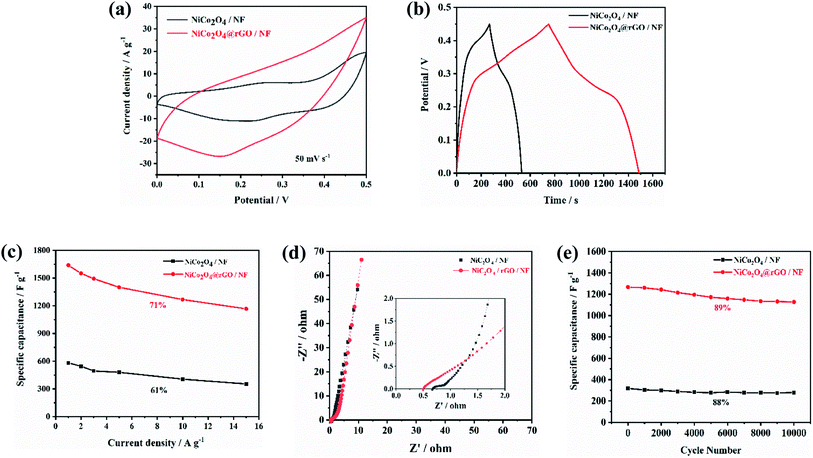 |
| Fig. 10 Comparison of electrochemical performance of NiCo2O4@rGO/NF with NiCo2O4/NF: (a) CV curves (50 mV s−1), (b) GCD curves (1 A g−1), (c) specific capacitances at different current densities, (d) Nyquist plots of EIS and (e) cycling stability (10 A g−1). | |
Table 1 Comparison of electrochemical performance of NiCo2O4@rGO/NF in this work with previous literatures
Materials |
Specific capacitance |
Cycle stability |
Rate performance |
References |
rGO/NiCo2O4 |
1248 F g−1 (2 mA cm−2) |
90% (5 mA cm−2) |
59% (2–10 mA cm−2) |
30 |
2000 cycles |
NiCo2O4 nano-needles/NF |
1410 F g−1 (1 A g−1) |
94.7% (20 A g−1) |
73.8% (1–20 A g−1) |
31 |
3000 cycles |
NiCo2O4/rGO |
1380 F g−1 (1 A g−1) |
90% (5 A g−1) |
70% (1–10 A g−1) |
32 |
1000 cycles |
rGO@NiCo2O4 NFs |
1040 F g−1 (1 A g−1) |
88% (30 A g−1) |
88.8% (1–10 A g−1) |
45 |
5000 cycles |
NiCo2O4@NF |
646.6 F g−1 (1 A g−1) |
96.5% (7 A g−1) |
32% (1–9 A g−1) |
46 |
3000 cycles (ASC) |
NiCo2O4@rGO/NF |
1644 F g−1 (1 A g−1) |
89% (10 A g−1) |
71% (1–15 A g−1) |
This work |
10 000 cycles |
In addition, the asymmetric supercapacitor cell (ASC) was fabricated from two-electrode devices by using the prepared NiCo2O4@rGO/NF as the positive electrode and graphene hydrogel (GH) as the negative electrode in 6 M KOH electrolyte, illustrated in Fig. 11(a). As shown in Fig. 11(b), the CV curve of NiCo2O4@rGO/NF positive electrode exhibits a potential range of 0 V to 0.55 V, whereas that of GH negative electrode shows a range of −1.0 V to 0 V. Therefore, CV measurements of NiCo2O4@rGO/NF//GH ASC were carried out at an operating potential window of 0 V to 1.55 V with the scan rate of 5, 10, 20, 50 and 100 mV s−1 (Fig. 11(c)), which indicate that the CV curves at all the scan rates retain both the pseudocapacitive characteristic and electrochemical double layer capacitance (EDLC). Besides, the redox peak has a slight movement with the increase of the scan rate, perhaps owing to the electrode polarization.47 Fig. 11(d) depicts GCD curves of NiCo2O4@rGO/NF//GH ASC at various current densities on the potential window of 0 V to 1.55 V. With the increase of the current density, the curves could maintain an approximate symmetrical triangle, showing good reversibility. The calculated specific capacitance of the ASC device is 84.13, 74.58, 68.32, 60.32 and 45.81 F g−1 at the current density of 1, 2, 3, 5 and 10 A g−1, respectively. The rate performance of NiCo2O4@rGO/NF//GH ASC is displayed in Fig. 11(e). When the current density increased to 10 A g−1, it could still maintain 54.5% of the specific capacitance at 1 A g−1. Fig. 11(f) describes the cycle performance of NiCo2O4@rGO/NF//GH ASC at a current density of 10 A g−1. After 10
000 cycles, the specific capacitance increased to 51.76 F g−1, which is 113% of the initial value (45.81 F g−1), which is probably because of the electrolyte has fully penetrated into the material, making the active material be more fully utilized with the continuous charge–discharge cycles, demonstrating excellent cycle stability of NiCo2O4@rGO/NF//GH ASC device.
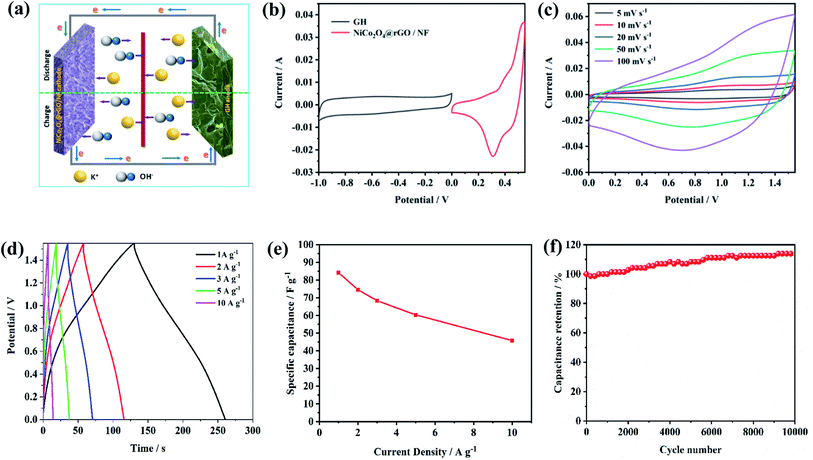 |
| Fig. 11 (a) Schematic of the fabricated NiCo2O4@rGO/NF//GH asymmetric supercapacitor device, (b) CV curves of GH anode and NiCo2O4@rGO/NF cathode electrodes (5 mV s−1), (c) CV curves of NiCo2O4@rGO/NF//GH at various scan rates, (d) GCD curves of NiCo2O4@rGO/NF//GH at various current densities, (e) specific capacitances of NiCo2O4@rGO/NF//GH at various current densities and (f) cycling performance of NiCo2O4@rGO/NF//GH device (10 A g−1). | |
4. Conclusion
In conclusion, NiCo2O4@rGO/NF composite electrode materials with enhanced supercapacitive performance were synthesized by a simple hydrothermal method assisted by CTAB combined with subsequent heat treatment. The aeolotropic NiCo2O4@rGO nanoneedles grew directly on Ni foam with rGO connecting to each other and without any binder, which has greatly improved the conductivity of electrode materials. Moreover, the crossed network also provides a pathway for ion diffusion. For supercapacitor application, the highest specific capacitance of NiCo2O4@rGO/NF could reach 1644 F g−1 at a current density of 1 A g−1, and even at 15 A g−1, the value still maintain 1167 F g−1, additionally, there is 89% capacitance retention after 10
000 cycles at 10 A g−1. Furthermore, NiCo2O4@rGO/NF//GH ASC device was assembled and exhibits a high specific capacitance of 84.13 F g−1 (1 A g−1) and excellent cycle stability (113% capacitance retention after 10
000 charge/discharge cycles at 10 A g−1). Higher specific capacitance, better rate performance and cycle stability highlight the advantages of NiCo2O4@rGO/NF composites as electrode materials for supercapacitors, associating with the regulated morphology, suitable GO concentration synergy of components. As one of the most promising materials, it is believed that NiCo2O4@rGO/NF will have a broader application prospect in the future.
Conflicts of interest
There are no conflicts to declare.
Acknowledgements
We are grateful for the support from the National Natural Science Foundation of China (51904077) and the Natural Science Foundation of Hebei Province (E2019203469).
References
- R. Vellacheri, A. Al-Haddad, H. Zhao, W. Wang, C. Wang and Y. Lei, High performance supercapacitor for efficient energy storage under extreme environmental temperatures, Nano Energy, 2014, 8, 231–237 CrossRef CAS.
- A. S. Westover, J. W. Tian, S. Bemath, L. Oakes, R. Edwards, F. N. Shabab, S. Chatterjee, A. V. Anilkumar and C. L. Pint, A multifunctional load-bearing solid-state supercapacitor, Nano Lett., 2014, 14, 3197–3202 CrossRef CAS PubMed.
- J. Chmiola, C. Largeot, P. L. Taberna, P. Simon and Y. Gogotsi, Monolithic carbide-derived carbon films for micro-supercapacitors, Science, 2010, 328, 480–483 CrossRef CAS PubMed.
- D. Pech, M. Brunet, H. Durou, P. Huang, V. Mochalin, Y. Gogotsi, P. L. Taberna and P. Simon, Ultrahigh-power micrometre-sized supercapacitors based on onion-like carbon, Nat. Nanotechnol., 2010, 5, 651–654 CrossRef CAS PubMed.
- J. Duay, S. A. Sherrill, Z. Gui, E. Gillette and S. B. Lee, Self-limiting electrodeposition of hierarchical MnO2 and M(OH)2/MnO2 nanofibril/nanowires: mechanism and supercapacitor properties, ACS Nano, 2013, 7, 1200–1214 CrossRef CAS PubMed.
- A. K. Singh, D. Sarkar, G. G. Khan and K. Mandal, Hydrogenated NiO nanoblock architecture for high performance pseudocapacitor, ACS Appl. Mater. Interfaces, 2014, 6, 4684–4692 CrossRef CAS PubMed.
- Y. Z. Su, K. Xiao, N. Li, Z. Q. Liu and S. Z. Qiao, Amorphous Ni(OH)2@ three-dimensional Ni core–shell nanostructures for high capacitance pseudocapacitors and asymmetric supercapacitors, J. Mater. Chem. A, 2014, 2, 13845–13853 RSC.
- K. Wang, H. Wu, Y. Meng and Z. Wei, Conducting polymer nanowire arrays for high performance supercapacitors, Small, 2014, 10, 14–31 CrossRef CAS PubMed.
- G. F. Chen, Z. Q. Liu, J. M. Lin, N. Li and Y. Z. Su, Hierarchical polypyrrole based composites for high performance asymmetric supercapacitors, J. Power Sources, 2015, 283, 484–493 CrossRef CAS.
- A. Ramadoss, K. N. Kang, H. J. Ahn, S. I. Kim, S. T. Ryu and J. H. Jang, Realization of high performance flexible wire supercapacitors based on 3-dimensional NiCo2O4/Ni fibers, J. Mater. Chem. A, 2016, 4, 4718–4727 RSC.
- J. W. Mao, C. H. He, J. Q. Qi, A. B. Zhang, Y. W. Sui, Y. Z. He, Q. K. Meng and F. X. Wei, An asymmetric supercapacitor with mesoporous NiCo2O4 nanorod/graphene composite and N-doped graphene electrodes, J. Electron. Mater., 2017, 47, 512–520 CrossRef.
- Z. Wu, Y. Zhu and X. Ji, NiCo2O4-based materials for electrochemical supercapacitors, J. Mater. Chem. A, 2014, 2, 14759–14772 RSC.
- H. Tong, S. Yue, F. Jin, L. Lu, Q. Meng and X. Zhang, Honeycomb-like NiCo2O4@Ni(OH)2 supported on 3D N-doped graphene/carbon nanotubes sponge as an high performance electrode for supercapacitor, Ceram. Int., 2017, 44, 3113–3121 CrossRef.
- Y. Sun, X. Xiao, P. Ni, Y. Shi, H. Dai, J. Hu, Y. Wang, Z. Li and Z. Li, DNA-templated synthesis of nickel cobaltite oxide nanoflake for high-performance electrochemical capacitors, Electrochim. Acta, 2014, 121, 270–277 CrossRef CAS.
- L. Zhang, L. Dong, M. Li, P. Wang, J. Zhang and H. Lu, Ultrahigh-rate, ultralong-life asymmetric supercapacitors based on few-crystalline, porous NiCo2O4 nanosheet composites, J. Mater. Chem. A, 2018, 6, 1412–1422 RSC.
- H. Jiang, J. Ma and C. Li, Hierarchical porous NiCo2O4 nanowires for high-rate supercapacitors, Chem. Commun., 2012, 48, 4465–4467 RSC.
- R. Zou, K. Xu, T. Wang, G. He, Q. Liu, X. Liu, Z. Zhang and J. Hu, Chain-like NiCo2O4 nanowires with different exposed reactive planes for high-performance supercapacitor, J. Mater. Chem. A, 2013, 1, 8560–8566 RSC.
- E. Jokar, A. Irajizad and S. Shahrokhian, Synthesis and characterization of NiCo2O4 nanorods for preparation of supercapacitor electrodes, J. Solid State Electrochem., 2015, 19, 269–274 CrossRef CAS.
- C. An, Y. Wang, Y. Huang, Y. Xu, C. Xu, L. Jiao and H. Yuan, Novel three-dimensional NiCo2O4 hierarchitectures: solvothermal synthesisand electrochemical properties, CrystEngComm, 2014, 16, 385–392 RSC.
- Y. Zhu, X. Ji, R. Yin, Z. Hu, X. Qiu, Z. Wu and Y. Liu, Nanorod-assembled NiCo2O4 hollow microspheres assisted by an ionic liquid as advanced electrode materials for supercapacitors, RSC Adv., 2017, 7, 11123–11128 RSC.
- Y. Li, X. Hou, Z. Zhang, Z. Hai, H. Xu, D. Cui, S. Zhuiykov and C. Xue, NiCo2O4 particles with diamond-shaped hexahedron structure for high-performance supercapacitors, Appl. Surf. Sci., 2018, 436, 242–251 CrossRef CAS.
- W. Liu, C. Lu, K. Liang and B. K. Tay, A three dimensional vertically aligned multiwall carbon nanotube/NiCo2O4 core/shell structure for novel high-performance supercapacitors, J. Mater. Chem. A, 2014, 2, 5100–5107 RSC.
- L. Wang, X. Jiao, P. Liu, Y. Ouyang, X. Xia, W. Lei and Q. Hao, Self-template synthesis of yolk–shelled NiCo2O4 spheres for enhanced hybrid supercapacitors, Appl. Surf. Sci., 2018, 427, 174–181 CrossRef CAS.
- H. Chen, C. K. Hsieh, Y. Yang, X. Y. Liu, C. H. Lin, C. H. Tsai, Z. Q. Wen, F. Dong and Y. X. Zhang, Hierarchical nickel cobaltate-manganese dioxides core–shell nanowire arrays on graphene-decorated nickel foam for high-performance supercapacitors, ChemElectroChem, 2017, 4, 2414–2422 CrossRef CAS.
- D. Cai, B. Liu, D. Wang, L. Wang, Y. Liu, R. Wang, Q. Li and T. Wang, Construction of unique NiCo2O4 nanowire@CoMoO4 nanoplate core/shell arrays on Ni foam for high areal capacitance supercapacitors, J. Mater. Chem. A, 2014, 2, 4954–4960 RSC.
- H. Wang, C. M. B. Holt, Z. Li, X. Tan, B. S. Amirkhiz, Z. Xu, B. C. Olsen, T. Stephenson and D. Mitlin, Graphene-nickel cobaltite nanocomposite asymmetrical supercapacitor with commercial level mass loading, Nano Res., 2012, 5, 605–617 CrossRef CAS.
- M. J. Allen, V. C. Tung and R. B. Kaner, Honeycomb carbon: a review of graphene, Chem. Rev., 2010, 110, 132–145 CrossRef CAS PubMed.
- K. S. Novoselov, A. K. Geim, S. V. Morozov, D. Jiang, Y. Zhang, S. V. Dubonos, I. V. Grigorieva and A. A. Firsov, Electric field effect in atomically thin carbon films, Science, 2004, 306, 666–669 CrossRef CAS PubMed.
- S. S. Jayaseelan, S. Radhakrishnan, B. Saravanakumar, M. K. Seo, M. S. Khil, H. Y. Kim and B. S. Kim, Mesoporous 3D NiCo2O4/MWCNT nanocomposite aerogels prepared by a supercritical CO2 drying method for high performance hybrid supercapacitor electrodes, Colloids Surf., A, 2018, 538, 451–459 CrossRef CAS.
- C. Zhang, C. Lei, C. Cen, S. Tang, M. Deng, Y. Li and Y. Du, Interface polarization matters: enhancing supercapacitor performance of spinel NiCo2O4 nanowires by reduced graphene oxide coating, Electrochim. Acta, 2018, 260, 814–822 CrossRef CAS.
- H. Yang, C. Zeng, C. Sun, M. Wang and Y. Gao, Mesopores NiCo2O4 nano-needles directly grown on Ni foam as high-performance electrodes for supercapacitors, Mater. Lett., 2020, 279, 128523 CrossRef CAS.
- Z. Wei, J. Guo, M. Qu, Z. Guo and H. Zhang, Honeycombed-like nanosheet array composite NiCo2O4/rGO for efficient methanol electrooxidation and supercapacitors, Electrochim. Acta, 2020, 362, 137145 CrossRef CAS.
- N. I. Kovtyukhova, P. J. Ollivier, B. R. Martin, T. E. Mallouk, S. A. Chizhik, E. V. Buzaneva and A. D. Gorchinskiy, Layer-by-layer assembly of ultrathin composite films from micron-sized graphite oxide sheets and polycations, Chem. Mater., 1999, 11, 771–778 CrossRef CAS.
- X. Li, L. Jiang, C. Zhou, J. Liu and H. Zeng, Integrating large specific surface area and high conductivity in hydrogenated NiCo2O4 double-shell hollow spheres to improve supercapacitors, NPG Asia Mater., 2015, 7(3), e165 CrossRef CAS.
- W. Zhang, W. Xin, T. Hu, Q. Gong, T. Gao and G. Zhou, One-step synthesis of NiCo2O4 nanorods and firework-shaped microspheres formed with necklace-like structure for supercapacitor materials, Ceram. Int., 2019, 45(7), 8406–8413 CrossRef CAS.
- S. Sahoo and J. J. Shim, Facile synthesis of three-dimensional ternary ZnCo2O4/reduced graphene oxide/NiO composite film on nickel foam for next generation supercapacitor electrodes, ACS Sustainable Chem. Eng., 2016, 5, 241–251 CrossRef.
- M. C. Biesinger, B. P. Payne, L. W. M. Lau, A. Gerson and R. S. C. Smart, X-ray photoelectron spectroscopic chemical state quantification of mixed nickel metal, oxide and hydroxide systems, Surf. Interface Anal., 2009, 41, 324–332 CrossRef CAS.
- L. Wang, X. Jiao, P. Liu, Y. Ouyang, X. Xia, W. Lei and Q. Hao, Self-template synthesis of yolk–shelled NiCo2O4 spheres for enhanced hybrid supercapacitors, Appl. Surf. Sci., 2018, 427, 174–181 CrossRef CAS.
- Y. Changzhou, L. Jiaoyang, H. Linrui, Z. Xiaogang, S. Laifa and L. X. Wen, Ultrathin mesoporous NiCo2O4 nanosheets supported on Ni foam as advanced electrodes for supercapacitors, Adv. Funct. Mater., 2012, 22, 4592–4597 CrossRef.
- A. Lv, S. Lu, W. Xu, Z. Wang, Y. Shen and G. Liu, One-pot synthesis of NiCo2O4/rGO/NF hybrid electrode materials realizing ultrahigh capacitance and rapid charge/discharge at large current density, Appl. Surf. Sci., 2020, 511, 145538 CrossRef CAS.
- W. Zou, L. Zhang, L. Liu, X. Wang, J. Sun, S. Wu, Y. Deng, C. Tang, F. Gao and L. Dong, Engineering the Cu2O-reduced graphene oxide interface to enhance photocatalytic degradation of organic pollutants under visible light, Appl. Catal., B, 2016, 181, 495–503 CrossRef CAS.
- L. Zhang, D. Zhang, Z. Ren, M. Huo, G. Dang, F. Min, Q. Zhang and J. Xie, Mesoporous NiCo2O4 micro/nanospheres with hierarchical structures for supercapacitors and methanol electroeoxidation, ChemElectroChem, 2017, 4, 441–449 CrossRef CAS.
- M. Ye, L. Ma, M. Gan, Y. Zhou, X. Li, F. Cao, F. Yan and Y. Zhai, One-step integration of the C/NiCo2O4 mesoporous nanoneedle arrays on Ni foam for high-performance hybrid supercapacitors, Appl. Surf. Sci., 2018, 456, 390–397 CrossRef CAS.
- Z. Fan, J. Yan, T. Wei, L. Zhi, G. Ning, T. Li and F. Wei, Asymmetric supercapacitors based on graphene/MnO2 and activated carbon nanofiber electrodes with high power and energy density, Adv. Funct. Mater., 2011, 21, 2366–2375 CrossRef CAS.
- A. Singh, S. K. Ojha and A. K. Ojha, Facile synthesis of porous nanostructures of NiCo2O4 grown on rGO sheet for high performance supercapacitors, Synth. Met., 2020, 259, 116215 CrossRef CAS.
- D. R. Kumar, K. R. Prakasha, A. S. Prakash and J. J. Shim, Direct growth of honeycomb-like NiCo2O4@Ni foam electrode for pouch-type high-performance asymmetric supercapacitor, J. Alloys Compd., 2020, 836, 155370 CrossRef CAS.
- C. Huang, Y. Ding, C. Hao, S. Zhou, X. Wang, H. Gao, L. Zhu and J. Wu, PVP-assisted growth of Ni–Co oxide on N-doped reduced graphene oxide with enhanced pseudocapacitive behavior, Chem. Eng. J., 2019, 378, 122202 CrossRef CAS.
Footnote |
† Electronic supplementary information (ESI) available. See DOI: 10.1039/d1ra09139e |
|
This journal is © The Royal Society of Chemistry 2022 |