DOI:
10.1039/D1RA08981A
(Review Article)
RSC Adv., 2022,
12, 13609-13627
Green aspects of photocatalysts during corona pandemic: a promising role for the deactivation of COVID-19 virus
Received
11th December 2021
, Accepted 17th March 2022
First published on 6th May 2022
Abstract
The selection of a facile, eco-friendly, and effective methodology is the need of the hour for efficient curing of the COVID-19 virus in air, water, and many food products. Recently, semiconductor-based photocatalytic methodologies have provided promising, green, and sustainable approaches to battle against viral activation via the oxidative capabilities of various photocatalysts with excellent performance under moderate conditions and negligible by-products generation as well. Considering this, recent advances in photocatalysis for combating the spread of the severe acute respiratory syndrome coronavirus 2 (SARS-CoV-2) are inclusively highlighted. Starting from the origin to the introduction of the coronavirus, the significant potential of photocatalysis against viral prevention and -disinfection is discussed thoroughly. Various photocatalytic material-based systems including metal-oxides, metal-free and advanced 2D materials (MXenes, MOFs and COFs) are systematically examined to understand the mechanistic insights of virus-disinfection in the human body to fight against COVID-19 disease. Also, a roadmap toward sustainable solutions for ongoing COVID-19 contagion is also presented. Finally, the challenges in this field and future perspectives are comprehensively discussed involving the bottlenecks of current photocatalytic systems along with potential recommendations to deal with upcoming pandemic situations in the future.
1. Introduction
1.1 Origin and spread of COVID-19
People with pneumonia who were bordering on acute respiratory patients in Wuhan, China, were first infected with a new, severe acute respiratory syndrome coronavirus (SARS-CoV-2) among different species.1,2 After the emergence of coronavirus, the SARS-Cov-2 has been found responsible for infecting millions of people worldwide, which has strong virulence, high spreading nature, as well as contagious nature of the coronavirus (COVID-19).1–4 The seventh coronavirus, SARS-CoV-2, is observed to infect humans. MERS-CoV, SARS-CoV-2, HKU1 and NL63 cause severe maladies, whereas OC43 and 229E offer mild and low symptoms.5,6 In addition, the SARS-Cov-2 virus acts as an etiologic agent for COVID-19, a global pandemic with no absolute cure according to the World Health Organization (WHO) and COVID-19 infections are still increasing about 250 million confirmed cases and 5 million deaths (on 13 November 2021) in more than 120 countries.7,8 Ideally, SARS-CoV-2 is a single-stranded ribonucleic acid (RNA) beta-virus having a non-segmented positive-sense with a spherical shape of 50–150 nm diameter range and club-shaped (S, glycoprotein) spike protein projections.9,10 Furthermore, the COVID-19 virus surface is composed of a membrane (M) of glycoproteins and an envelope (E) of the symmetrical nucleocapsid (N) helical with the genome of the virus as well.10–12 In addition, SARS-CoV-2 employs entry of cells in the human body with angiotensin-converting enzyme 2 (ACE2) receptor applications.11–13 Since, SARS-Cov-2 has been rapidly spreading in symptomatic or asymptomatic patients in different ways as respiratory droplets while talking, skin contacts, air particles or aerosols, and touching contaminated solid surfaces, such as paper, wood, glass, copper, steel, or cloths, and weather conditions, such as light, pH, temperature, or humidity.14–18
Owing to the corona pandemic, numerous human societies have to adopt voluntary or government-forced segregation or isolation, use of masks, protective gloves, sanitizer for hand washing, and social distancing by reduced personal contact in public places.19,20 Thus, infected or used protective clothes, gloves and masks might have viral traces, which are needed to be separated aside from tens of hours to seven days to self-destroy coronavirus.21,22 The scientific methods lack exact coronavirus destruction time, which depends on the components of materials used to manufacture face masks as woven or non-woven polymeric fibers. However, a customer might utilize sanitizing agents for virus protection via the interaction between porous fibers and alcohol sanitizers.21–23 All types of pathogens, including food, water, and air, might enter via different infection modes, resulting in ∼15 million casualties worldwide yearly.24,25 Various microbial contaminations, including viral contaminants of drinking water, breathing air, and food products pose huge environmental warnings along with adverse effects on the health of humans.26,27 In addition, water-and food-borne outbreaks might be related to environmental conditions with the ecosystem variation all over the world.28–31 Clearly, such viruses can be moved by a direct surface contact along with air transfer causing a majorly high risk of the disease compared to other microbial pathogenic contaminants.32–35 Due to the growing virus-based epidemic and pandemics in the world, swine flu virus (H1N1), SARS-CoV, MERS-CoV, and novel SARS-CoV-2 (COVID-19) have gained increasing attention of researchers and scientists about viral diseases and their efficient curing.36–40
1.2 Photocatalysts for COVID-19
Newly identified coronavirus disease (COVID-19) is caused by a highly infectious and rapidly transmitted severe acute respiratory syndrome of coronavirus (SARS-CoV-2).41 CoV-2 is a single-stranded (ss) ribose nucleic acid (RNA) genome and was first reported in Wuhan, China in December 2019.42 Rapidly transmitted COVID-19 has been declared a pandemic by the world health organization (WHO) since March 2020 in most countries including India, and hence, called the third zoonotic epidemic of the 21st century.43 The newly discovered beta SARS-CoV-2 belongs to the family Coronaviridae; order Nidovirales; genus betacoronavirus that resembled 96.2% with the bat CoV and 79.5% with the spherical SARS-CoV.44 SARS-CoV-2 is considered as the largest family of single-stranded enveloped genomic RNA (∼125 nm or 0.125 microns) with 5′-cap and 3′poly-A tail structures.45 The functionality of the structural components, including the envelope (E), membrane (M), spike (S), and nucleocapsid (N) are the four major proteins that normalize the function and the structure of CoV-2 (Fig. 1a). Enhanced levels of COVID-19 pandemic and the transmission risk globally are due to the higher estimated reproduction number of SARS-CoV-2 (R0, 2–3.5), as compared to SARS-CoV (1.77).46
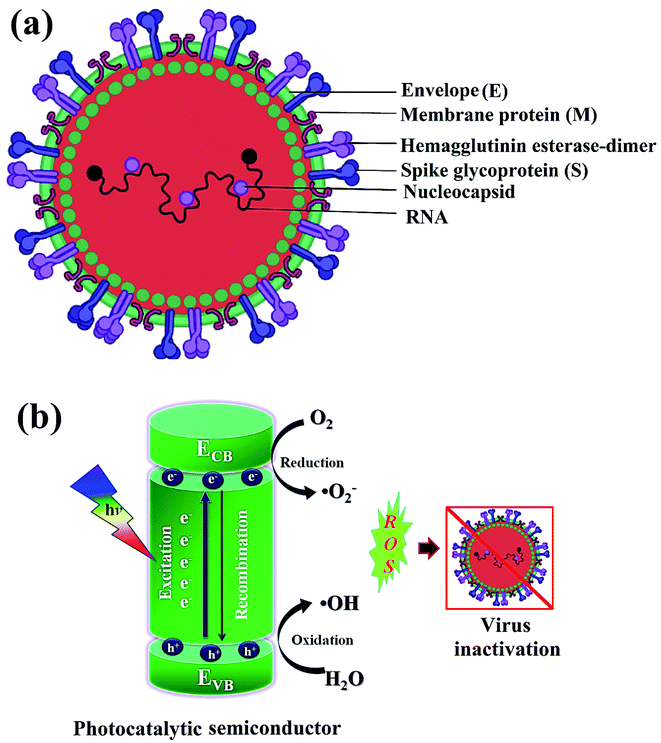 |
| Fig. 1 Schematics illustrating (a) structure of SARS-CoV-2 with structural protein and (b) coronavirus inactivation through photocatalysis by the generation of reactive oxidative species (ROS). | |
In the last two years, the COVID-19 virus has been intensively researched as extremely communicable where coronavirus efficiently transfers through respiratory droplets and aerosols in terms of cough, spit, or saliva during speaking, sneezing, as well as strong breathing, resulting in millions of cases worldwide. According to the U.S. Centres for Disease Control and Prevention (CDCP, USA) recommendations, personal protective equipment (PPE) including surgical or medical masks, and social distancing are the best and most efficient measures for self-protection from COVID-19 until a reliable or effective solution is obtained to check this coronavirus spread. Among the various types of masks, surgical and N95 respiratory masks are the most effective, which are composed of a thin polypropylene (PP) layer fabric (having negative charge) to filter efficiently particles and droplets containing viruses.47,48 Furthermore, various conventional techniques, including chromatography, pasteurization, acidic pH inactivation, precipitation, and UV inactivation are being used to remove virus particles from the wastewater. The use of various chemical methods and exposure of the virus to high temperatures (>65 °C) deactivates the coronavirus to some extent. However, all these conventional techniques are not able to disinfect harmful COVID-19 viruses completely.
Moreover, toxic chemicals or harmful radiations cause etching or allergy in the body when they come in contact with skins and sometimes may evaporate to form secondary contaminants.46–48 To overcome such inherited drawbacks associated with conventional methods, many approaches are enlightening different ways to disinfect harmful viruses present on any surface using nanomaterials. In addition, nanomaterials with significant properties invade viruses to enable the expression of antigens; thus, hold great potential to fight against COVID-19. Various nanomaterials having safe, less toxic, economic, and biocompatible properties, are well explored to prevent, detect and disinfect harmful coronaviruses.43,47–49
1.3 Green aspects of photocatalysis
Green or sustainable chemistry is mainly focused on the design of products and processes that eliminate the use and generation of harmful substances. There is a need to generate antiviral materials that are low-cost, renewable, and easily available to disinfect harmful viruses.43,49 In this regard, photocatalysis or artificial photosynthesis is an effective advanced oxidation technology that has received noteworthy attention owing to its ease of handling, low energy requirement and thus, considered as one of the most encouraging technologies for resolving climate-change or environmental pollution problems. Additionally, the provided whole amount of solar energy is more than sufficient, thereby, the real challenge is its effective collection and utilization for living beings.50 Of note, photo redox catalysis has several advantages for sustainability; moreover, it also accomplishes several principles of green chemistry.
In photocatalysis, the primary energy source (solar light) is free in an enormous amount, easily available, and environmentally friendly, where in, the absorbed photons provide a huge amount of energy without requiring high temperature or harsh conditions.51 The photocatalytic reaction occurs under the joint interaction of light with adequate frequency and the photocatalytic material absorbs a wide range of solar spectra (Fig. 1b). Typically, by acquiring an excited state after light absorption, a single-electron migration event triggers the generation of active electron (e−) and hole (h+) pairs as excitons in the CB and VB of photoactive semiconductor, respectively.52 The isolated e− in the CB can reduce dissolved O2 and produce H2O2, or ˙O2− along with H+ ions, whereas, the remaining h+ in the VB can oxidize the OH− ions from water to generate unstable ˙OH radicals.53 These generated reactive oxygen species (ROS; ˙OH, H2O2, H+, and ˙O2−) participate in the photocatalytic-disinfection and -degradation processes at room temperature.54,55 For instance, Matsuura et al. analysed a reduced surface spike morphology, increased size, and ∼99.9% antiviral efficiency of the photoactive TiO2 against SARS-CoV-2 after 20 min of solar irradiation.56 Similarly, Nica et al. found iron and nitrogen-doped TiO2 nanoparticles as potent antimicrobial candidates that displayed excellent anti-biofilm activity, yet with low toxicity against lung and dermal cells.55 Moreover, photoactive antiviral materials with a high surface area were also effectively coated on various textile materials. Furthermore, the virucidal activity of treated fabrics showed improved virus inactivation capacity, while uncoated fabrics showed no activity under light illumination. The hydrophobic interactions were majorly involved in the adsorption of viruses on the surface of coated fabrics, causing distortion of viral shape resulting in induced virus inactivation in ambient conditions. Thus, the surface effect was especially important for TiO2 coated samples to observe significant virucidal activity in dark as well. For example, –OH and –COOH functional groups modified TiO2 obtained from hydrosol, which significantly facilitated the retention of TiO2 particles within the fibers of fabrics. In addition, the presence of hydrosols might increase surface area by one to two orders of magnitude after the addition of TiO2 particles. Thus, generated free radicals from TiO2 particles might damage the viral surface proteins, which in turn reduce the adsorption capacity of viruses to host cells as well as damage the viral genome, preventing the replication process of the virus.55,56 So, the attachment of TiO2 particles to cellulose fibers could also be useful to produce virucidal activity in different fabrics. In hospitals, cotton-based items are usually washed and reused because the cotton fabric is covered with TiO2 particles, which provides a low level of wash resistance owing to the poor adhesion between TiO2 particles and fibers. The high surface area of TiO2 particles and hydrophilicity of the coated fabrics could contribute to the antiviral properties. Moreover, similar virus inactivation was observed after one cycle of washing TiO2-coated fabrics.58 Other potential photocatalytic semiconducting nanomaterials, such as WO3, graphene, carbon-based, and 2D nanomaterials are also reported as antiviral agents with long-term photostability under ambient conditions. Hence, heterogeneous photocatalysis using these antiviral semiconductors is relatively safe, non-hazardous and eco-friendly and does not generate any harmful by-products.
During the COVID-19 crisis, metal oxides having self-disinfecting properties were a useful tool for virus protection, interaction and its spread from person to person. In addition, titanium dioxide (TiO2) exhibits efficient antiviral, antibacterial, and photocatalytic activities in fighting the COVID-19 pandemic.57,58 Under UV light irradiation, the catalytic efficiency of TiO2 nanomaterials was diagnosed for virus-deactivation as TiO2 nanoparticle thin films deposited on glass, resulting in excellent virucidal performance.59 Thus, reactive efficiencies of TiO2 nanomaterial surfaces have been efficiently utilized for coronavirus disinfection. Subsequently, cobalt-doped TiO2 nanomaterials were reported for the treatment of SARS-CoV-2 infection using a cost-effective electrochemical bio-sensor in nasal or saliva secretions based on spike protein (RBD) sensing on the surface of coronavirus.60 Hence, the electrochemical anodization method has been used to fabricate functionalized TiO2 nanotubes in the wet-chemical process to precisely detect SARS-CoV-2 in patients in a short time and minimum amounts. Furthermore, TiO2 nanomaterials have displayed various benefits including catalytic activity, large surface area, and antiviral applications. Thus, hydrothermally grown photoactive TiO2 nanoparticles modify many pathogens of coronavirus at ∼375 nm wavelength under low incident light (∼0.4 mW cm2) irradiation61 resulting in efficient interaction with the human pathogen, SARS-CoV-2 virus. Here, ROS species formed during light absorption, particularly hydroxyl radicals (˙OH), play a crucial role in attaching and inactivating the SARS-CoV-2 pathogen, which showed huge applicability in medical, chemical and engineering research as well.60,61
Additionally, photocatalytic antimicrobial materials do not produce any immunological response and are easily compatible when exposed to living tissues. Meanwhile, the mask has been playing a crucial role in controlling the spread of the coronavirus (Fig. 2a) as a point, which displays a clear difference in the SEM images (Fig. 2b and c) of the uncoated and coated masks for virus protection.61 For instance, the absence of cytotoxicity after a short time of exposure to the TiO2-1% Fe–N-treated cotton knit system, highlighted its potential use in the development of effective antimicrobial agents.62 Moreover, photoactive nanomaterials are repeatedly used without any substantial loss in their photocatalytic antiviral activity. In a report, non-toxic photoactive SnO2 (core)@ZIF-8 (shell) composite with antiviral properties against chikungunya virus can be effectively reutilized for up to 5 consecutive cycles. In addition, a small amount of photoactive semiconductor material is required to perform effective redox reactions for viral disinfection. Mechanistically, induced oxidative stress (˙O2−, ˙OH) damaged structural proteins, coenzyme A and disturbed cellular respiration activity of the virus eventually causing cell lysis. Therefore, understanding the real disinfection mechanistic route might help in the development of more powerful photoactive nanomaterials. The green technology thus completely inactivates and degrades species and pollutants without generating any secondary pollution in the environment.63
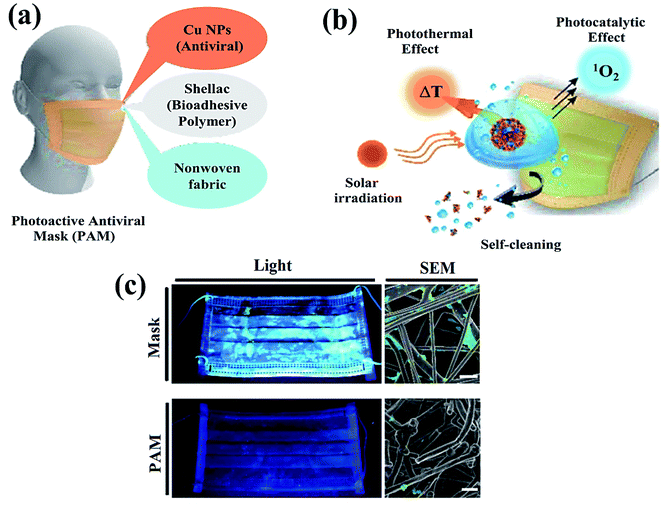 |
| Fig. 2 Schematic illustration representing (a) nanocomposite coating and individual components of a surgical mask, (b) viral disinfection via photocatalytic, photo-assisted heat treatment, and hydrophobic self-sterilization operations after sunlight illumination, and (c) digital scans (left) and scanning electron microscopy (SEM, right) results of the uncoated surgical mask (top) and photoactive mask (PAM, bottom) with E. coli after exposure to solar light for 24 h. Reproduced with permission from ref. 61, copyright American Chemical Society, 2020. | |
To date, various reports have been filed signifying photocatalytic viral inactivation via the generation of a broad spectrum of ROS causing oxidation of organic materials present in viral membranes followed by the destruction of the cell wall and cell rupture, leading to the complete deactivation of the virus. In view of the strong potential of photocatalysis to inactivate various virus species, for the first time, we aim to provide an all-inclusive study that depicts recent advances in the development of distinct photocatalytic materials to prevent and disinfect the SARS-CoV-2 virus. Hence, the green aspects of photocatalysis involving energy sources, types of photocatalytic materials and mechanistic insights are also highlighted and discussed. The significance of photocatalysis involving metal oxides, metal-free, and advanced 2D materials (MXenes, MOFs, and COFs) for antiviral activities is extensively reviewed. Also, a sustainable roadmap to overcome current bottlenecks and promote photocatalytic antiviral performance in order to battle similar forthcoming pandemics is also presented. In conclusion, various challenges in this field and future outlook are deliberated for a better understanding of current limitations and possible solutions to kill this deadly virus.
2. Discussion and types of semiconductor photocatalysts
Considering the current emphasis on sustainable energy development, photocatalysis offers a promising alternative for microbial inactivation and prevention with the fascinating features of low to a negligible generation of byproducts, the probable complete deactivation of microbial pathogens and substantial utilization of green solar energy.64 In 1994, Sierka and Sjogren's pioneer work represented virus disinfection in water for the first time by using photocatalysis technology.64 The study involved a TiO2 photocatalyst for the inactivation of MS2 microphages under the illumination of ultraviolet (UV) light.
As a result, the research focus shifted to developing more photocatalytic materials, such as metal oxides, iron-based materials, metal-free photocatalysts, metal–organic frameworks (MOFs), and covalent organic frameworks (COFs) for their potential utilization in antimicrobial activities.65–72 Considering this, the following section will summarize various photocatalytic materials and their potential for prevention and disinfection against viral pathogens.
2.1 General mechanism of photocatalysis
In the photocatalysis mechanism (Fig. 3), the photocatalysts excited by ultraviolet/visible light and valence band (VB) electrons move to the conduction band (CB). In the presence of light, the chemical oxidation initiates the disinfection of various types of coronaviruses through short-lived reactive oxygen species (ROS) using catalysts. The virus disinfection can only be obtained with mechanical destruction of the virus after heating or biocidal metal/metal oxide nanomaterials or their composites collectively applied for antimicrobial or catalytic properties.73 After light absorption, the formation of ROS efficiently damages the cytoplasmic membrane and cell wall during the virus disinfection mechanism. From VB, the holes interact with ROS species to form an active hydroxyl (˙OH) radical, a powerful oxidant that oxidizes chemicals in the shell and capsid of the virus. Subsequently, the excited e− of the CB interacts with O2 to produce hydroperoxide radical (˙OOH) followed by reduced into superoxide radical anion
with the H+ ion.74 Thus, disinfection of various pathogens might be controlled according to the choice of materials and ROS amount obtained under solar/visible/UV lights. The generated ROS attack interactive sites to control the inactivation of the virus on extra and -intracellular sites.75 Furthermore, cell walls and membranes of extracellular active sites have a complicated layered structure with a peptidoglycan layer, a lipopolysaccharide layer followed by phospholipid bilayers. In the cytoplasm of microbial cells, the intracellular active sites are composed of ribosomes, DNA, RNA, and enzymes.76 However, the size of catalyst nanomaterials is crucial for efficient catalytic activity and ROS diffusion might be reduced if the size of the catalyst in the cell is more than 300 nm. The cells might be disinfected by oxidation of a non-protein substance, coenzyme A, during the photocatalytic process.77 The proposed mechanism of the photocatalytic creation of free radicals follows: |
Photocatalyst + hν → eCB− + hVB+
| (1) |
|
O2(ads) + eCB− → ˙O2−
| (2) |
|
˙O2H + ˙O2H → O2 + O2H2
| (4) |
|
H2O + hVB+ → +H + OH˙
| (6) |
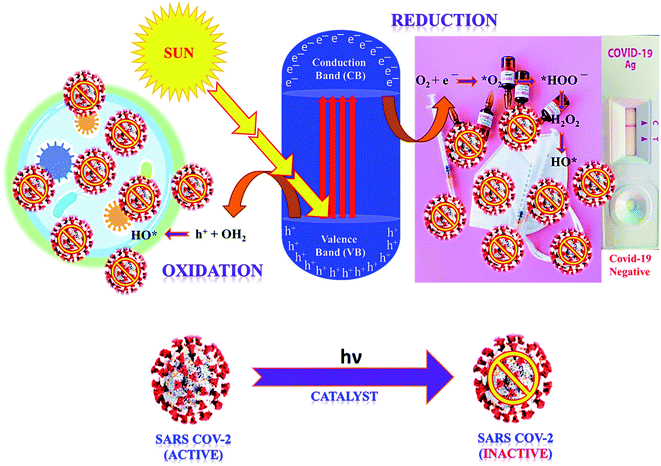 |
| Fig. 3 The proposed mechanism of SARS COV-2 virus inactivation in photocatalysis. | |
Thus, light-induced chemical oxidation (Fig. 3) is a promising disinfection method applied in ventilation, air conditioning, and heating to kill pathogenic microbes absorbed on the surface.78 However, such dead virus cells gradually reduce their activity with time and are repaired by the doping of catalyst nanomaterials, resulting in an excellent virucidal activity. Thus obtained ROS might impact the lipids, DNA, and cell membranes by the selective attack on nucleotides and sulfhydryl bonds.79 During the advanced oxidation process, the biocidal activity of ˙OH radicals might be assessed by its assault on unsaturated fatty acid (FA) chains that result in the peroxidation of lipids and start catalytic chain reactions to damage the cell membrane and FA chains of the viral pathogens.80 Thus, formed peroxyl (ROO˙) radicals have low reactivity, and a long half-life to inhibit FA chains than ˙OH radicals that create reactive intermediates. Thus, ROS supports cell damage in oxidative stress, which might be repaired via the antioxidant process in microbial cells, where in, microbes might inactivate ROS radicals and destruct healthy cells.81 Such ROS collapse different polypeptide chains of proteins by charge modification, resulting in structural modification of amino acids which inhibit the activity of protein due to less active site.
Additionally, ROS, particularly ˙OH radicals, have the ability to change the surface morphology of microbes, including Bacillus subtilis, Bacillus spores, and Serratia marcescens.82 Among them, Bacillus spore cells contain a hardcover of many layers, which breaks with ˙OH radicals interaction to change its spherical shape and death of cells. However, many microorganisms have the capability to override the oxidation using the superoxide dismutase (SOD) enzyme of the metalloenzyme (MnSOD, Cu/ZnSOD, and FeSOD) family, which degrades the power of oxidants.83 In general, E. coli produces MnSOD enzymes upon oxygen exposure, which is supported by superoxide radicals. In a similar way, Staphylococcus aureus protects ROS through various oxidative-defense methods based on the identification of molecular sentinels with a reply to oxidative stress signals.84 While, viruses lacking envelops show less damage, the enveloped viruses display more damage under hygroscopic environmental conditions.
2.2 Metal/metal oxide photocatalysts
Metal oxide photocatalysts offer vital potential to generate ROS under the illumination of solar light and can substantially facilitate photocatalytic viral disinfection. Typically, the generation of ROS involving ˙O2−, H2O2 and ˙OH radicals at the surface of photocatalyst is a major step responsible for the disinfection of viruses, due to their ability to oxidize various organic components of microorganisms such as lipid peroxidation resulting in cell wall and cell membrane rupture, protein alteration, and DNA destruction.85 So far, TiO2 nanomaterials (Fig. 4) are the most explored photocatalyst for microbial disinfection due to their considerably inert behavior, low noxiousness, and significant photostability.86 Interestingly, TiO2 is widely utilized in paints, self-sterilization windows, lacquers, photocatalytic water purification, and H2 generation.87,88 Moreover, TiO2-containing paints have shown a potential role in ambient air purification due to the ability of TiO2 to decompose volatile organic compounds (VOCs) under UV light illumination.89 However, the release of toxic products after the degradation of VOCs by TiO2-based photocatalytic paints makes this process challenging and questionable.87 In that regard, TiO2-doped paints could be of utmost interest for surface decontamination and deactivation of SARS-CoV-2 along with aerosol abatement through air filtration filters equipped with TiO2 photocatalysts that can be subjected to UV light irradiation.89
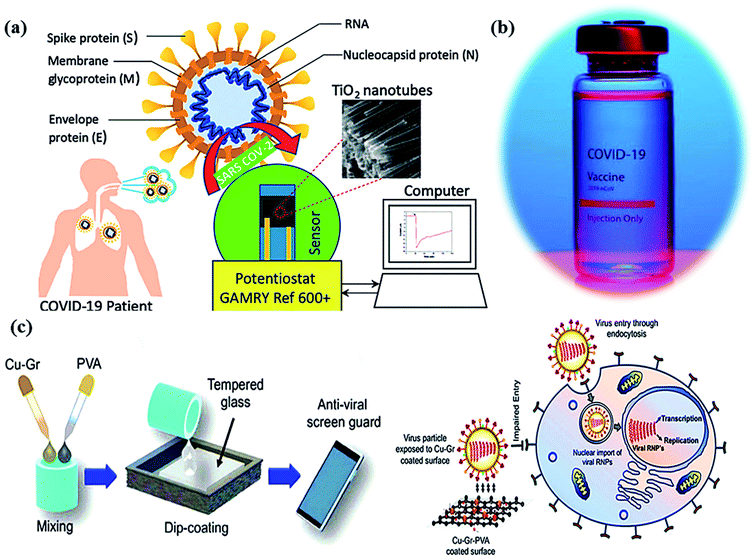 |
| Fig. 4 (a) Schematic of a Co-functionalized TiO2 nanotube (Co-TNT) based sensing platform for the detection of SARS-CoV-2. Reproduced from ref. 90 with permission from Multidisciplinary Digital Publishing Institute (MDPI), copyright 2020, (b) corona vaccine, and (c) an illustration diagrammatically illustrating the dip-coating technique for tempered glass units with PVA-based Cu–Gr nanocomposite substrates and the mechanism of action for tempered glass surfaces coated with Cu–Gr. Reproduced from ref. 93 with permission from the American Chemical Society, copyright 2020. | |
In molecular docking study, the different iron oxides as Fe2O3 and Fe3O4 are shown to interact with glycoproteins of SARS-CoV-2 necessary to bind with receptors of host cells that inactivate the virus by modifying its glycoproteins.90,91 Generally, COVID-19 can be measured using a reverse transcription-polymerase chain reaction (RT-PCR) test in the laboratory within ∼2 hours. Thus, a research team has developed a portable device (as nano-PCR) for quick testing within <20 minutes by using plasmonic heating through magneto-plasmonic nanomaterials.92 This nano-PCR device (Fig. 4a–c) is reliable, portable, and precise (∼500%), with high sensitivity (∼500%) and excellent specificity (∼500%). It gives on-site COVID-19 detection and provides the establishment of ambulatory clinics for many patients with outstanding accuracy of testing.93
The influenza virus is arbitrated via endocytosis with viral ribonucleoproteins (RNPs) imported into the nuclei of the host cells. The RNPs accomplish transcription, and replication of the virus in the nucleus to form novel RNPs.94 Another potential strategy to prevent viral spread and inactivate microorganisms is by developing photocatalytic antiviral face masks. Owing to hydrophobic features (Fig. 5a and b) and photocatalytic activeness of the coatings, vast research efforts have been put forward to exploit the self-cleaning properties of nanomaterials via photo-activation, which can be substantially beneficial for commercial and the medical sector.95–97 The surface coating of both Cu and CuO nanoparticles (Fig. 2) has been shown to possess considerable antimicrobial activity even against SARS-CoV-2 after 4 h of exposure to plastics and stainless steel, where the virus can live up to 72 h. Inspired by this, Kumar et al. reported a smart and facile technique to construct an antiviral mask by a coating a non-woven surgical mask with shellac/copper (Cu and CuO) nanoparticles to enhance the hydrophobicity of the mask's surface and make it resistant to aqueous droplets (Fig. 2a and b).60 The resulting photocatalytic mask exhibited remarkable photo activity and photothermal features for viral activity along with superior reusability and self-sterilization efficacy. As can be seen from Fig. 2c, under the illumination of solar light, PAM suppressed bacterial growth as compared to pristine masks, which is confirmed from SEM images. Under the exposure to light, the temperature at the surface of the mask increased up to 70 °C, leading to the generation of reactive radical species, which facilitated the membrane rupture of nanosized (∼100 nm) viruses.
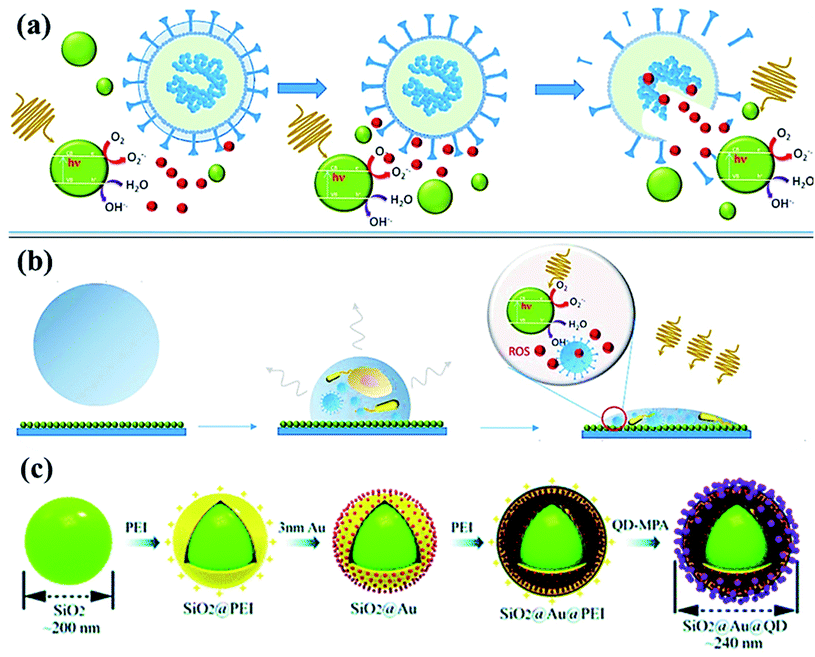 |
| Fig. 5 (a) Photoactivation of nanoparticles to produce reactive oxygen species (ROS) during photocatalytic degradation resulting in viral inactivation. (b) Photocatalysis at contaminated NPs coated solid surface upon irradiation, and (c) sequential process for fabricating dual-mode SiO2@Au@QD fluorescent labels. Reproduced with permission from ref. (Current Opinion in Chemical Engineering, 2019, 1:100716) from Elsevier, 2019 and ref. 98 of the American Chemical Society, copyright 2020. | |
Recently, SARS-CoV-2-based antibodies might be distinguished by quick, novel and accurate techniques using SiO2@Au@quantum-dot (QD) with spike proteins as a lateral flow immunoassay bio-sensor via fluorescent detection.98 By using serum (∼1 μL) sample, SiO2@Au@QD assay exhibits ∼100 times higher efficiency in a short time (<20 min) compared to Au-based assay.99 From Fig. 5, the assay developed in the layer-by-layer assembly of SiO2 nanosphere followed by the polymer [poly (ethylene imine), PEI] with the positive-charge formation on the surface. In addition, negatively-charged Au nanoparticles (Fig. 5c) of <5 nm size were formed followed by the PEI polymer coating, then, functionalized-quantum dots (carboxylate QDs) layer, which created a strong fluorescent colorimetric signal during sensing.98–100 Meanwhile, SARS-CoV-2 virus spike protein forms covalent-coupling in SiO2@Au@QDs (Fig. 5c) followed by deposition of anti-human IgM/IgG on the test lines to obtain efficient, selective and COVID-19 virus-sensitive reports.
2.3 Metal-free photocatalysts
Visible light active metal-free photocatalysts represent a promising category of cost-effective, non-toxic, and stable semiconductor materials, which should be substantially explored for energy generation and water treatment applications.101–105 Although metal-free photocatalysts (Fig. 6) such as graphene, graphene oxide, and g-C3N4 have not much exploited for fighting SARS-CoV-2 infection yet, their fascinating physicochemical and antiviral activities recommend the potential usage of these materials in preventing and disinfecting COVID-19 viruses via the construction of coated air filters, face-masks and waste-water disinfectants. Typically, the antimicrobial activity of graphene nanomaterials relies on various effects such as membrane stress, oxidative stress, and photothermal stress along with charge migration and entrapment influence of graphene derivatives.106 However, in contrast with the bactericidal activity, the antiviral activity of graphene-based nanomaterials is less studied mainly due to the difference in the size of virus (2–300 nm) and bacteria (500–5000 nm) making analysis problematic and expensive. Akhavan et al. investigated the antiviral activity of graphene–tungsten oxide nanocomposite for photo-assisted inactivation of bacteriophage MS2 viruses.106,107 For understanding mechanistic insights into the deactivation process, the destruction of protein capsid and efflux of viral RNA encapsulated in protein was investigated. The results further revealed that graphene–tungsten oxide nanocomposite showed significant recyclability with less than 10% decline in the RNA efflux after 20 catalytic cycles under 60 h irradiation period.
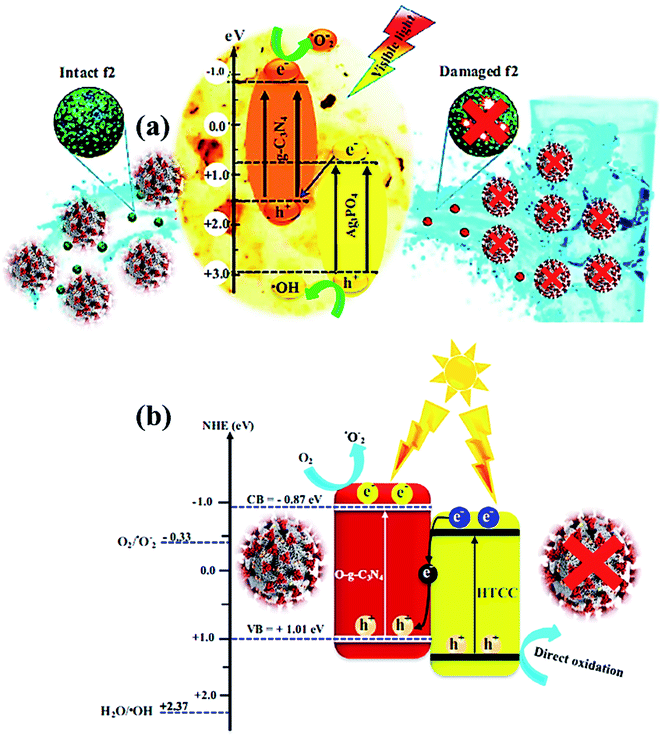 |
| Fig. 6 (a) Photocatalytic mechanism for bacteriophage f2 inactivation by AgCN photocatalysts under visible light. Reproduced with permission from ref. 108 copyright 2018 Elsevier, (b) possible Z-scheme mechanism for O-g-C3N4/HTCC photocatalyst. Reproduced with permission from ref. 110 copyright 2019 Elsevier. | |
The photocatalytic inactivation was applied using Ag3PO4/g-C3N4 nanocomposites (AgCN) in hydrothermal synthesis to study the bacteriophage f2 virus.108 Due to the combined effects of Ag3PO4 and g-C3N4, the performance was boosted as the proposed photocatalytic Z-scheme mechanism (Fig. 6a) via wide absorption of the visible spectrum and efficient charge carrier separation. The inactivation Z-scheme mechanism of the f2 virus by binary nanocomposite catalysts was performed with radical quenching tests, which exhibited catalytic disinfection efficiency of the f2 virus found of ∼6.5
log in 80 min on visible-light exposure due to selective virus damage by the ROS after charge separation through g-C3N4 and Ag3PO4 components.109 Hence, a novel, promising nanocomposite photocatalyst was developed for viral disinfection originating from contaminated water. In addition, a new metal-free nanocomposite was synthesized in a two-step hydrothermal process as oxygen-doped g-C3N4/hydrothermal carbonation carbon (O-g-C3N4/HTCC) microspheres. This nanocomposite displayed excellent virucidal efficiency for HAdV-2 with visible-light absorption to disinfect 5
log in 2 h under optimized conditions. Thus, improved disinfection activity of O-g-C3N4/HTCC nanocomposite against the virus was governed through the Z-scheme mechanism with efficient OH creation to heavy damage of the rigid capsid of HAdV-2 (Fig. 6b) after an excellent charge separation process.110,111
2.4 Antiviral activity of GO
In general, GO is the oxidized product of graphene with different oxygen-based function groups on the surface as ketone, epoxide, hydroxyl, and carboxyl groups.112 GO displays excellent hydrophilicity, bonding ability, and wettability compared to pristine graphene due to the presence of more active sites on the edges and surfaces.113 In addition, GO is applicable as an antibacterial and anti-cancer agent owing to its high conductivity, mechanical strength, surface-to-volume ratio, and 2-D monolayer structure with a negatively charged surface. GO might interact with the plasma membrane of viruses to produce reactive oxygen species (ROS) and the antiviral activity of GO was measured with a DNA virus called porcine herpes virus, resulting in the inhibition of virus infection in non-cytotoxic samples.114 In addition, GO/PVP nanocomposite has exhibited strong antiviral activity owing to the non-ionic nature of PVP polymer where GO might damage virus structure by reducing virus insertion into host cells.115 Graphene oxide (GO) is another potential semiconductor material possessing surface functionalization of hydroxyls, ketones, epoxides, diols, and carboxyl groups, which was found beneficial for rupture of coating proteins leading to efflux of RNA under aqueous conditions.112–114 The oxygen atoms present at basal planes render superior hydrophilicity, dispersibility in H2O and attachability to GO in contrast with graphene, leading to better antimicrobial activity. However, the interaction of GO with proteins after the inactivation process results in superficial bioreduction of GO into graphene form. On the other hand, the reduced form of GO, called reduced graphene oxide (rGO) functionalized with polysulfated dendritic polyglycerol has also exhibited considerable inactivation features against several viruses encompassing orthopoxviruses, equine herpesvirus type 1 (EHV-1), and herpes simplex virus type 1 (HSV-1).115,116 With further advancements in technology, graphene-based materials are widely explored for decontamination and disinfection of SARS-CoV-2 by constructing self-sterilized air filters and antiviral face masks, which are necessary prevention measures against COVID-19. For instance, Stanford et al. developed a self-sterilized air filter equipped with laser-induced graphene (LIG), which is microporous and conductive in nature.116,117 Typically, a free-standing LIG membrane comprising a carpet of porous fibers (Fig. 7a–c), which promoted the capture of microorganisms, specifically bacteria along with a restriction on the proliferation of filtered microorganisms. Furthermore, LIG membrane filter, as shown in Fig. 7d, was supported with periodic Joule-heating, which boosted the temperature (>300 °C) and facilitated the decomposition of bacteria along with other molecules and microorganisms such as pyrogens, endotoxins, exotoxins, allergens, mycotoxins, prions, and nucleic acids (Fig. 7e). Therefore, by integrating nanosized membrane technology with a greener and advanced photocatalytic process, graphene-based materials could be effectively utilized to prevent and fight against COVID-19.
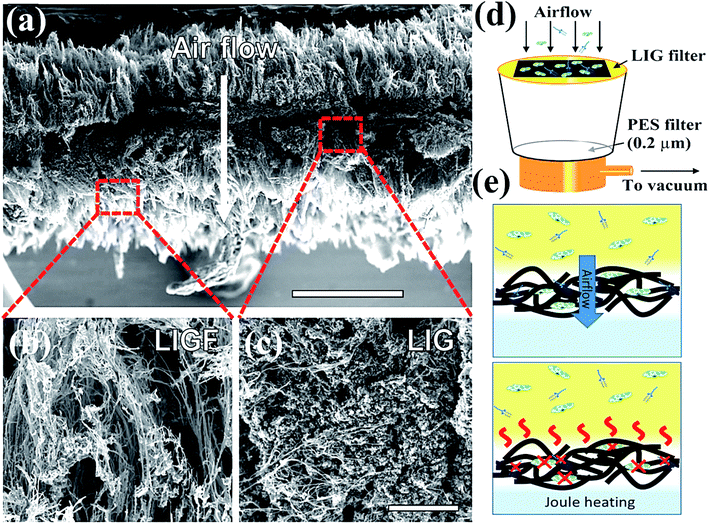 |
| Fig. 7 Schematics representing (a) intrinsic morphology and thickness of laser-induced graphene (LIG) filter, which boosts filtration efficacy (b) SEM image depicting the outer fibrous carpet region of the LIG filter useful to trap larger constituents and aerosols, (c) SEM image showing porous graphene portion possessing tortuous 2.86–8.94 nm pores, which facilitate bacterial and finer particle capture, (d) LIG filter system placed on a vacuum filtration arrangement, (e) schematic depiction of filtration (top) leading to sterilization and Joule-heating assisted depyrogenation (bottom). Reprinted with permission from ref. 117, copyright American Chemical Society, 2019. | |
As a metal-free photocatalyst, g-C3N4 can be another potential candidate for visible light-enabled photocatalytic inactivation of the SARS-CoV-2 virus. To date, the antiviral and antibacterial activity of g-C3N4-based photocatalysts has been substantially evaluated owing to its suitable bandgap of 2.7 eV with appropriate valence and conduction band potentials. For instance, g-C3N4 exhibited superior inactivation of E. coli,118 Staphylococcus aureus (S. aureus),119 MS2 bacteriophages,120 human adenoviruses,121 and Bacillus anthracis endospores122 by the generation of reactive radical species under the illumination of UV-visible light.
2.5 Other 2D materials
For catalytic deactivation of SARS CoV-2 virus, several 2D materials such as MXenes, metal–organic frameworks (MOFs), and covalent organic frameworks (COFs) are emerging potential semiconductor photocatalysts with alluring features involving good conductivity, layered structure, mechanically firm, flexible, large surface area, and offer high affinity for guest materials.123,124 Typically, 2D carbides and nitrides (MXenes) having formula Mn+1XnTx (where M = Ti, Zr, V, Mo, etc., X = C and/or N, and Tx depicts surface-functionalized
O, –OH, –F, and –Cl groups) with n ranging from 1 to 4, offer significantly high surface area and porosity leading to superior adsorption of guest molecules and viruses.125,126 Moreover, the visible light activity of these materials facilitates the inactivation of the surface adsorbed virus via the generation of ROS. Additionally, the plasmon resonance property of MXenes (Fig. 8) under visible or infrared (IR) light exposure helps to convert light into heat (photothermal effect), which further adds up to deactivate viral species and even enables their application in phototherapy.127 Recently, in order to amend the photocatalytic antibacterial activity of MXenes, a Schottky heterojunction with interfacial engineering based on the work function values of Ti2C2Tx MXenes coupled with Bi2S3 was constructed.128 The work function engineering helped boost charge carrier transference and enabled the fast killing of bacteria. Other than that, MXenes and their composites can be utilized as protective coatings on the personal protective equipment (PPE) leading to a promising alternative to develop reusable PPEs in order to overcome the burgeoning disposal waste crisis.127,129,130
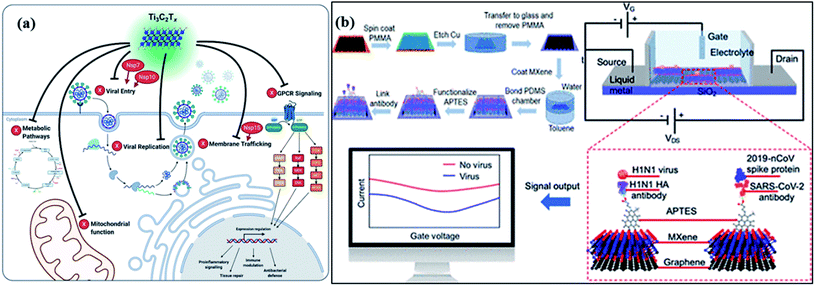 |
| Fig. 8 (a) The mechanism of MXene-dependent viral inhibition of Ti3C2Tx to explore viral inhibition activity at the cell surface, and (b) an illustration of antibody-antigen sensing in a FET circuit and change in the drain-source current is achieved by MXene–graphene VSTM deposition. Photograph: courtesy of “Yanxiao Li”. Copyright 2020 and the image is of the free domain. | |
In addition, an MXene-dependent antiviral action mechanism (Fig. 8) has been proposed using proteomics data for docking analysis to compare with SARS-CoV-2 protein interactions. Various SARS-CoV proteins, including NSP7, NSP10 and NSP15, interact with MXenes (as Ti3C2Tx) to produce obstacles in the viral lifecycle via membrane trafficking followed by G-protein coupled receptor (GPCR) signaling, and mitochondrial function for metabolic pathways resulting in viral replication.131 Therefore, the capability of MXenes provides an excellent antiviral activity by modulation of viral proteins (Fig. 8a) containing host proteins such as GRPEL1, NUTF2 and GNG5, which regulate antiviral activity. Furthermore, the interaction of host proteins with SARS-CoV (red-colored) proteins. In addition, viral protein, NSP7 is present in membrane trafficking and GPCR signaling while NSP15 is involved in nuclear transport and vesicle trafficking in SARS-CoV-2 infection. Frequently, NSP7 and NSP10 modify endomembrane to support the insertion of a virus and its replication. Hence, the Ti3C2Tx treatment functioned in all pathways of Vero E6 cells (Fig. 8b), resulting in SARS-CoV-2 inhibition.132
Another fascinating class of 2D materials with excellent photocatalytic activities involves metal–organic frameworks (MOFs) and covalent organic frameworks (COFs). The potential features of these materials involve high porosity, physiochemical stability, structural tunability, wide host–guest interactions, sorption, and ion release ability, which make them a potential candidate for various photocatalytic applications and in biomedical fields.133,134 Recently, zinc-based imidazole MOFs (ZIF-8) have shown outstanding ∼100% virus-inactivation efficiency in 30 minutes against E. coli under solar light owing to their excellent destruction of the cell wall of bacteria. Hence, MOFs (Fig. 9) might be applied in the industrial-size production of filters for air-cleaning masks, cloths, ventilators, and air purifiers.135 Recently, bismuth and bismuth–graphene (Bi@graphene) nanocomposites were developed as a photocatalytic air purifier under UV irradiation. Furthermore, Bi@graphene nanocomposites showed excellent photocatalytic deactivation performance against E. coli compared to the pristine bismuth nanospheres.136 Thus, the improved antibacterial activity of nanocomposites related to highly oxidative ROS generation from Bi surface followed by graphene results in strong excitation and fast charge transfer process.137 Subsequently, aluminum-terephthalate-based MOFs (Fig. 9a) have been reported to construct air purifiers for airborne bacterial removal and humidity management for indoor applications. Importantly, monohydroxy terephthalate-based MOFs displayed excellent disinfecting photocatalytic performance of ∼99.94% under <60% RH, and ∼500 cycling performance against E. coli bacteria.138 Specifically, monohydroxy-terephthalate-coated air filters of non-woven fabric protect efficiently against sudden humidity changes of air in outdoor conditions. Thus, this work gives a meaningful outcome for the next-generation development of antibacterial, water adsorbents, and active filters to manage the quality of air and humidity under indoor conditions.136–138
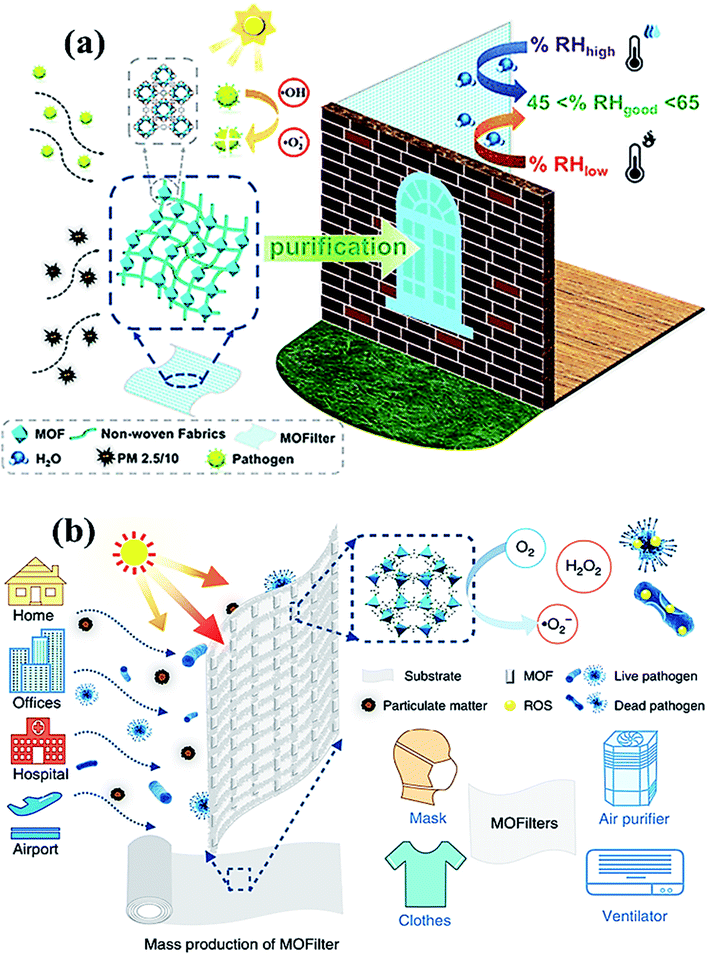 |
| Fig. 9 (a) MOF filter (CAU-1) using functionalized terephthalic acid ligands for indoor humidity, and microbial growth, to prevent pollution. Reproduced with permission from ref. 138, copyright American Chemical Society, 2020. And (b) MOF-based filters used in different living areas. Reprinted with permission from ref. 141, copyright © 2019 Springer Nature Limited, Nature, 2019. | |
Owing to their tunable structure, biocompatibility, and encapsulation ability, both COFs and MOFs have been utilized as drug delivery agents with optimal efficacy.139,140 Nevertheless, to prevent and control the viral spread, MOFs embedded air filters, which possess superior adsorption and photocatalytic virucidal and bactericidal activities have also been explored and reported lately.141 Moreover, Li et al. reported [Ag4(μ-PTA)2(μ3-PTA)2(μ4-pma)(H2O)2]n·6nH2O (bioMOF 1) bioactive metal–organic framework (bioMOF 1) and investigated antiviral, antibacterial, and antifungal properties under exposure to light. The report suggested that the bioactive MOF (Fig. 9b) successfully disinfected E. coli, P. aeruginosa and S. aureus bacteria along with C. albicans yeast with considerably lower minimum inhibitory concentration (MIC) values. Furthermore, bioMOF 1 also showed HAdV-36 deactivation activity and high cytotoxicity toward abnormal epithelioid cervix carcinoma (HeLa) cell line, suggesting the potential of MOFs against the COVID-19 virus. Therefore, MOFs and COFs could deactivate the SARS-CoV-2 virus by eliminating crown-like spike proteins by perforating the lipid membrane along with the efflux of RNA content for about 3 h under the illumination of UV light. However, the generation of ROS leads to impairment of spike proteins, resulting in superior virucidal activity under the photocatalytic inactivation process.142,143
2.6 Durability of photocatalysts
To detect viral particles in the air, various photocatalyst-based methods have been applied for virus inactivation during photocatalysis, which efficiently reduces the virus load in the air under indoor conditions. Thus, photocatalysis has been used to effectively remove various pollutants in gas or aqueous phases.144 Despite the precise removal of biological pollutants, the photocatalytic viral model might not be useful to different viruses in indoor air applications, such as volatile organic compounds (VOCs), pesticides, and dyes. During photocatalysis, virus inactivation is tuned according to the temperature and humidity of the environment with a change in the rate of photocatalysis. In airborne viruses, the viral inactivation mechanism might proceed through chemical oxidation through toxic metal ions released along with viral surface destruction.145 The flower-like TiO2 micro-nanoparticles were deposited on a cotton-type fabric surface using the hydrothermal method. The high thickness silver-nanoparticle (Ag NPs) film was formed uniformly on the surface of the TiO2@cotton fabric after sodium hydroxide pretreatment followed by in situ AgNO3 reduction. During the hydrothermal reaction, the concentration of AgNO3 has a crucial impact on microorganisms to tune their antibacterial activity along with high UV protective ability, having excellent 56.39 ultraviolet protection factor values.
Thus, the prepared fabric or textiles display excellent mechanical strength and durability after the rough cleaning and abrasion process. The multilayer surface roughness explores its mechanical stability, resulting in longer durability at high pressure of ∼255 kPa during textile, medical, or hygiene applications.146 During the COVID-19 pandemic, such fabric might repel coronaviruses, such as SARS-CoV-2 resulting in excellent safety for healthcare workers. Thus, PPE kits or medical clothing could be fabricated from various types of bacteria-, protein, blood, and virus-repelling fabrics in the near future (Table 1).
Table 1 Various types of photocatalysts applied for viral disinfection
Photocatalyst |
Types of virus |
Amount of catalyst (mg L−1) |
Light source |
Time of disinfection |
References |
TiO2 |
Phage f2 |
1000 |
Black light |
15 min |
78 |
TiO2 |
Murine norovirus |
10 |
UV lamp |
24 h |
16 |
Ag–TiO2 |
Hepatitis B |
100 |
UV lamp |
12 h |
12 |
nAg/TiO2 |
Phage MS2 |
100 |
UV lamp |
80 min |
76 |
Cu–TiO2 |
Bacteriophage f2 |
50 |
Xe lamp |
120 min |
47 |
Mn–TiO2 |
Phage MS2 |
100 |
Xe lamp |
60 min |
26 |
SiO2–TiO2 |
Phage MS2 |
100 |
UV lamp |
1.8 min |
71 |
C60/SiO2 |
Phage MS2 |
500 |
Sunlight |
75 min |
25 |
C70/SiO2 |
Phage MS2 |
300 |
Sunlight |
90 min |
87 |
g-C3N4 |
Phage MS2 |
135 |
Xe lamp |
240 min |
85 |
O-doped g-C3N4 |
Human adenovirus |
— |
LED lamp |
120 min |
31 |
Ag3PO4/g-C3N4 |
Bacteriophage f2 |
100 |
UV lamp |
80 min |
49 |
Ag–AgI/Al2O3 |
Human retrovirus |
320 |
Xe arc lamp |
40 min |
22 |
FeO |
Phage MS2 |
5 |
Simulated solar |
30 min |
17 |
Summarily, a perspective of using different semiconductor photocatalysts against COVID-19 could be a potential strategy to combat the ongoing COVID-19 crisis. However, research on photocatalytic prevention and inactivation of the SARS-CoV-2 virus is still in the infancy stage and requires substantial efforts for better outcomes. Based on the antiviral activities of different photocatalytic materials involving metal oxides (TiO2, WO3, CuO, etc.), metal-free photocatalysts (graphene, GO, rGO, and g-C3N4), and 2D semiconductor materials, they are being employed as coatings for masks and air filters, paints, and disinfectants to prevent community spread of the deadly virus.148–150 Additionally, owing to the photothermal effect of MXenes and targeted drug-carrier ability of MOFs/COFs, it is highly anticipated that these advanced materials can be smartly and intensively explored for the therapeutic treatment of COVID-19 disease in the future.
3. Significance and roadmap to sustainable solutions for pandemics
The impact of highly contagious COVID-19 on people and society is highly changeable and unthinkable, resulting in a high mortality rate in various countries.147 Thereby, keeping personal protection from rapid SARS-CoV-2 infection remains a serious and highly noteworthy challenging issue.150 Over the past few years, photocatalysis has been vastly explored for antiviral and antibacterial applications in living beings. The progression in photocatalysis in combating virus inactivation is depicted in Fig. 10. There are several measures including the use of PPE kits, social distancing, proper sanitization, surgical and medical (single use) masks that are suggested for COVID-19 control, and prevention for self-protection.147–150 Nevertheless, massive use of these preventive measures encounters significant challenges in the recycling and sterilizing of various utensils and cloths. The advantages of photocatalytic viral disinfection over homogeneous-phase advanced oxidation processes are well documented.
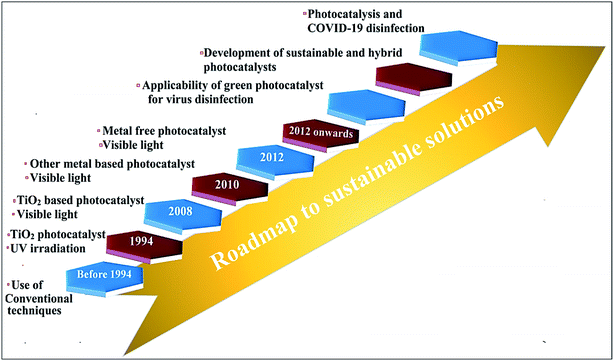 |
| Fig. 10 Roadmap depicting progression toward a sustainable solution for combating viruses through photocatalytic technology. | |
3.1 Designing rejuvenated, reusable, and biodegradable masks
To address various drawbacks associated with single useable masks, photocatalytic rejuvenation is a highly significant strategy that can sterilize the harmful microbes facially in the presence of light. Instead of using polypropylene as a precursor, alternative materials such as polyethylene oxide, cellulose nanofibers, and electrospinning of polyvinyl alcohol are also explored as effective materials for the fabrication of masks. As-fabricated sustainable and environmentally-friendly masks containing N–TiO2/TiO2 coating had 100% bacterial disinfection, indicating the efficiency of personal protective equipment (PPE) under ongoing and future pandemics.148 Photocatalytic self-sterilizing masks are designed for superior biodegradability, filterability, breathability, and mechanical strength that facilitate their handling and environmental impact.
3.2 Developing novel photoreactors
The spatial distribution of absorbed radiation in photocatalytic annular reactors is a crucial parameter for disinfecting harmful airborne microbes. Considering that, vast research efforts have been made to construct new photoreactors, such as packed-bed, monolithic, photocatalytic membrane, and micro-reactors to improve the efficiency and design of existing ones. In addition, the retention of airborne microbes in the bed, i.e., filtration and the action of the generated ˙OH radicals in photoreactors are two main processes involved in photocatalytic disinfection. In a study, TiO2 coated glass rings with high filtration capacity, and low-pressure drops were filled inside a reactor that was irradiated by UV-A lamps both internally and externally. A 100% disinfection rate of bacterial spores in 1 h was observed inside the reactor, highlighting the excellent packing material employed in bio-aerosol deactivation to mitigate ongoing and future pandemics.150
3.3 Photocatalytic assisted microwave plasma-based pyrolyzer
Highly hazardous biomedical waste, including sharps, masks, gloves, bottles, pathological, chemical, radioactive, paper, etc. can be effectively treated with a simple, affordable, and highly efficient photocatalytic chemical route with high efficiency. Complete pyrolysis of typical wastes at high temperatures into liquid oil, gases, and char using an explorable microwave plasma-based pyrolyzer is a highly sustainable approach.147 Typically, pyrolysis of polystyrene plastic gives styrene, toluene like essential oils as reported in the literature survey, describing the efficiency of this process. Furthermore, it is a high energy-saving process as char obtained after pyrolysis can be effectively utilized as solid fuels, for generating carbon nanofilaments. Other different catalysts with high surface areas and generated pyrolytic oils with organic, inorganic compounds have much use as combustion fuels, transportation fuels, power generation, wood preservatives, etc.147,148
3.4 Use of photocatalytic air filters
It is of immediate importance to develop a potent prevention tool to reduce rapid transmission along with an improved inactivation rate of harmful pathogens. Thus, disposable face masks neither inactivate microbes nor block their entry from natural surroundings efficiently. The development of photoactive TiO2 nanowires-based reusable air filters with 100–200 m2 filter capacity is a promising sustainable solution reported for the first time. As-synthesized filters are much more effective owing to their high surface area, polycrystalline counterparts, and super-hydrophilicity. Generated ˙OH, HO2˙, H2O2, 1O2, ˙O2− on the nanoporous TiO2 surface sustainably disinfect airborne bacteria, viruses including CoV-2. Moreover, as-fabricated filters further can also be used for purification or as conditioners.149,150 Thus, these above-mentioned sustainable, reusable, and eco-friendly methods could be a great alternative solution in dealing with the ongoing COVID-19 contagion.
4. Conclusions and future outlook
In summary, the global COVID-19 pandemic emergency has necessitated an urgent need for the development of a smart and efficient way to combat the coronavirus worldwide. Among various strategies, photocatalysis has been reported for the synergistic improvement of prevention, detection, diagnosis, and treatment of COVID-19 by using different types of catalysts under light illumination. As a green approach, solar light-driven photocatalysts have already been explored in the prevention and disinfection of various viruses and may ignite a ‘fresh start’ for the inactivation of SARS-CoV-2 with no issues such as stability, toxicity, cost-effectiveness, and availability. Considering that, in this review article, the existing progress of photocatalytic virucidal activities has been discussed and studied to present the latest advancements in fighting against the COVID-19 virus in an environmentally friendly way. Herein, the green aspects of photocatalysis have been explored along with its significance with respect to different photocatalysts including metal-oxide, metal-free, and other 2D materials for antiviral activities. Studies concerning the prevention and disinfection of distinct viruses and a promising path toward sustainable solutions for future pandemics have been pondered upon. Despite considerable research on photocatalytic viral inactivation, certain bottlenecks associated with semiconductor materials and process limits their photoactivity, which must be overcome in order to employ photocatalysis for SARS-CoV-2 disinfection in the future. Consequently, potential challenges in this field with a plausible future outlook are presented below:
• The recovery and the reusability of the suspended photocatalysts from the reaction solution are quite challenging owing to adsorbed species at the surface of the photocatalyst, which may or may not be degraded completely. Typically, the disinfection process via photocatalysis can be unsafe if the inactivation process is not completed and the photocatalyst still holds harmful viral species at the surface of the catalyst. Therefore, it is important to subject the photocatalyst under light irradiation after complete recovery from the reaction mixture in order to ensure the complete inactivation of any adsorbed viral species.
• In the case of photocatalytic coatings and membrane filters, the concept of self-sterilization is very crucial for long-run applicability and decline in critical waste generated via the disposal. However, there are very less reports which explain various strategies to improve the self-sterilization of photocatalytic coatings and enhance their reusability. Therefore, more research efforts are highly desirable to develop facile strategies, which promote the prevention, disinfection and reusability of photocatalytic coating materials.
• For waste water disinfection, the aggregation of nanosized photocatalytic material results in less active surface sites hence reduced photoactivity. Notably, immobilizing a photocatalytic material onto the porous or floating substrate can improve recovery along with agglomeration issues leading to enhanced reusability.
To conclude, for photocatalytic water disinfection, the barriers, which hinder long-scale applicability must be considered on priority bases for better results. Consequently, the complete eradication of the deadly SARS-CoV-2 by semiconductor-based photocatalysis demands much more extensive research.
Conflicts of interest
There are no conflicts to declare.
Acknowledgements
This project was funded by the Deanship of Scientific Research (DSR), King Abdulaziz University, Jeddah, Saudi Arabia under grant no. (KEP-34-130-40). The authors, therefore, acknowledge with thanks DSR's technical and financial support.
References
- J. A. Otter, C. Donskey, S. Yezli, S. Douthwaite, S. D. Goldenberg and D. J. Weber, J. Hosp. Infect., 2016, 92, 235–250 CrossRef CAS PubMed.
- Y. Bai, L. Yao and T. Wei, et al., Jama, 2020, 323, 1406–1407 CrossRef CAS PubMed.
- World Health Organization, WHO Director-General’s Opening Remarks at the Mission Briefing on COVID-19, 2020 Search PubMed.
- M. Pal, G. Berhanu, C. Desalegn and V. Kandi, Cureus, 2020, 12, e7423 Search PubMed.
- V. M. Corman, D. Muth, D. Niemeyer and C. Drosten, Adv. Virus Res., 2018, 100, 163–188 CAS.
- N. Zhu, D. Zhang, W. Wang, X. Li, B. Yang, J. Song, X. Zhao, B. Huang, W. Shi and R. Lu, et al., N. Engl. J. Med., 2020, 382, 727–733 CrossRef CAS PubMed.
- E. Dong, H. Du and L. Gardner, Lancet Infect. Dis., 2020, 20, 533–534 CrossRef CAS PubMed.
- F. Wu, S. Zhao, B. Yu, Y. M. Chen, W. Wang, Z. G. Song, Y. Hu, Z. W. Tao, J. H. Tian and Y. Y. Pei, et al., Nature, 2020, 579, 265–269 CrossRef CAS PubMed.
- M. Meselson, N. Engl. J. Med., 2020, 382, 2063 CrossRef PubMed.
- M. Bueckert, R. Gupta, A. Gupta, M. Garg and A. Mazumder, Materials, 2020, 13, 5211 CrossRef CAS PubMed.
- V. Doremalen, N. Bushmaker, T. Morris, D. H. Holbrook, M. G. Gamble, A. Williamson, B. N. Tamin, A. Harcourt, J. L. Thornburg and N. J. Gerber, et al., N. Engl. J. Med., 2020, 382, 1564–1567 CrossRef PubMed.
- L. Mahmoudian, A. K. Jashni, S. N. Hosseini and M. Paryan, Process Saf. Environ. Prot., 2019, 122, 328–338 CrossRef.
- N. Hutasoit, B. Kennedy, S. Hamilton, A. Luttick, R. A. Rahman Rashid and S. Palanisamy, Manuf. Lett., 2020, 25, 93–97 CrossRef PubMed.
- B. Pastorino, F. Touret, M. Gilles, X. D. Lamballerie and R. N. Charrel, Emerging Infect. Dis., 2020, 26, 2256–2257 CrossRef CAS PubMed.
- S. Riddell, S. Goldie, A. Hill, D. Eagles and T. W. Drew, Virol. J., 2020, 17, 145 CrossRef CAS PubMed.
- J. E. Lee, K. D. Zoh and G. P. Ko, Appl. Environ. Microbiol., 2008, 74, 2111–2117 CrossRef CAS PubMed.
- S. Giannakis, S. Liu, A. Carratala, S. Rtimi, M. T. Amiri, M. Bensimon and C. Pulgarin, J. Hazard. Mater., 2017, 339, 223–231 CrossRef CAS PubMed.
- G. Kampf, D. Todt, S. Pfaender and E. Steainmann, J. Hosp. Infect., 2020, 104, 246–251 CrossRef CAS PubMed.
- J. Otter, C. Donskey, S. Yazli, S. Douthwaite, S. Goldenberg and D. Weber, J. Hosp. Infect., 2016, 92, 235–250 CrossRef CAS PubMed.
- L. Casanova, S. Jeon, W. Rutala, D. Weber and M. Sobsey, Appl. Environ. Microbiol., 2010, 76, 2712–2717 CrossRef CAS PubMed.
- L. Casanova, W. Rutala, D. Weber and M. Sobsey, Epidemiol, 2010, 31, 560–561 Search PubMed.
- X. Hu, C. Hu, T. Peng, X. Zhou and J. Qu, Environ. Sci. Technol., 2010, 44, 7058–7062 CrossRef CAS PubMed.
- E. Cesewski and B. N. Johnson, Biosens. Bioelectron., 2020, 159, 112214 CrossRef CAS PubMed.
- A. El-Sayed and M. Kamel, Environ. Sci. Pollut. Res. Int., 2020, 27, 22336–22352 CrossRef CAS PubMed.
- K. J. Moor and J. H. Kim, Environ. Sci. Technol., 2014, 48, 2785–2791 CrossRef CAS PubMed.
- D. Venieri, I. Gounaki, V. Binas, A. Zachopoulos, G. Kiriakidis and D. Mantzavinos, Appl. Catal., B, 2015, 178, 54–64 CrossRef CAS.
- B. Prevost, M. Goulet, F. S. Lucas, M. Joyeux, L. Moulin and S. Wurtzer, Water Res., 2016, 91, 68–76 CrossRef CAS PubMed.
- N. Doss, G. Carré, V. Keller, P. André and N. Keller, Water, Air, Soil Pollut., 2018, 229, 29 CrossRef.
- M. Cho, H. Chung, W. Choi and J. Yoon, Appl. Environ. Microbiol., 2005, 71, 270–275 CrossRef CAS PubMed.
- T. Lupia, S. Scabini, S. M. Pinna, G. Di Perri, F. G. De Rosa and S. Corcione, J. Global Antimicrob. Resist., 2020, 21, 22–27 CrossRef PubMed.
- C. Zhang, M. Zhang, Y. Li and D. Shuai, Appl. Catal., B, 2019, 248, 11–21 CrossRef CAS.
- M. Xie and Q. Chen, Int. J. Infect. Dis., 2020, 94, 119–124 CrossRef CAS PubMed.
- Z. Xu, Y. Wu, F. Shen, Q. Chen, M. Tan and M. Yao, Aerosol Sci. Technol., 2011, 45, 1337–1349 CrossRef CAS.
- J. Kim and J. Jang, Aerosol Sci. Technol., 2018, 52, 557–566 CrossRef CAS.
- C. C. Lai, T. P. Shih, W. C. Ko, H. J. Tang and P. R. Hsueh, Int. J. Antimicrob. Agents, 2020, 55, 105924 CrossRef CAS PubMed.
- A. Bleibtreu, M. Bertine, C. Bertin, N. H. Fidouh and B. Visseaux, Médecine et Maladies Infectieuses, 2020, 50, 670–675 CrossRef PubMed.
- Y. Liu, A. A. Gayle, A. W. Smith and J. Rocklöv, Journal of Travel Medicine, 2020, 27, 1–4 Search PubMed.
- N. Petrosillo, G. Viceconte, O. Ergonul, G. Ippolito and E. Petersen, Clin. Microbiol. Infect., 2020, 26, 729–734 CrossRef CAS PubMed.
- H. Li, S. M. Liu, X. H. Yu, S. L. Tang and C. K. Tang, Int. J. Antimicrob. Agents, 2020, 56, 105949 CrossRef PubMed.
- P. K. Raghav and S. J. M. h. Mohanty, Med. Hypotheses, 2020, 144, 110031 CrossRef PubMed.
- M. K. Ahmed, M. Afifi and V. Uskoković, Journal of Infection and Public Health, 2020, 13, 1243–1246 CrossRef PubMed.
- A. M. Nasir, N. Awang, S. K. Hubadillah, J. Jaafar, M. H. D. Othman, W. N. W. Salleh and A. Ismail, J. Water Proc. Eng., 2021, 42, 102111 CrossRef.
- B. Bayarri, A. Cruz-Alcalde, N. Lopez-Vinent, M. M. Micó and C. J. Sans, J. Hazard. Mater., 2021, 415, 125658 CrossRef CAS PubMed.
- P. L. Truong, A. Kidanemariam and J. Park, J. Ind. Eng. Chem., 2021, 100, 19–39 CrossRef CAS.
- T. Teymoorian, T. Teymourian, E. Kowsari and S. Ramakrishna, J. Water Proc. Eng., 2021, 42, 102193 CrossRef.
- R. Barouki, M. Kogevinas, K. Audouze, K. Belesova, A. Bergman, L. Birnbaum, S. Boekhold, S. Denys, C. Desseille and E. Drakvik, Environ. Int., 2021, 146, 106272 CrossRef CAS PubMed.
- X. Zheng, Z.-P. Shen, C. Cheng, L. Shi, R. Cheng and D.-H. Yuan, Environ. Pollut., 2018, 237, 452–459 CrossRef CAS PubMed.
- E. V. Campos, A. E. Pereira, J. L. De Oliveira, L. B. Carvalho, M. G. Casagrande, R. De Lima and L. Fraceto, J. Nanobiotechnol., 2020, 18, 1–23 CrossRef PubMed.
- R. Cheng, L.-J. Shen, J.-H. Yu, S.-Y. Xiang and X. Zheng, Catalysts, 2018, 8, 406 CrossRef.
- P. Raizada, V. Soni, A. Kumar, P. Singh, A. A. P. Khan, A. M. Asiri, V. K. Thakur and V. H. Nguyen, Journal of Materiomics, 2020, 7, 388–418 CrossRef.
- V. Soni, P. Raizada, A. Kumar, V. Hasija, S. Singal, P. Singh, A. Hosseini-Bandegharaei, V. K. Thakur and V. Nguyen, Environ. Chem. Lett., 2021, 19, 1065–1095 CrossRef CAS.
- V. Soni, C. Xia, C. K. Cheng, V.-H. Nguyen, D. L. T. Nguyen, A. Bajpai, S. Y. Kim, Q. Van Le, A. A. P. Khan and P. Singh, Appl. Mater. Today, 2021, 24, 101074 CrossRef.
- V. Soni, P. Raizada, P. Singh, H. N. Cuong, S. Rangabhashiyam, A. Saini, R. V. Saini, Q. Van Le, A. K. Nadda and T. Le, Environ. Res., 2021, 202, 111622 CrossRef CAS PubMed.
- I. C. Nica, M. S. Stan, M. Popa, M. C. Chifiriuc, V. Lazar, G. G. Pircalabioru, I. Dumitrescu, M. Ignat, M. Feder, L. C. Tanase, I. Mercioniu, L. Diamandescu and A. Dinischiotu, Int. J. Mol. Sci., 2017, 18, 249 CrossRef PubMed.
- R. Matsuura, C. W. Lo, S. Wada, J. Somei, H. Ochiai, T. Murakami, N. Saito, T. Ogawa, A. Shinjo and Y. J. V. Benno, Viruses, 2021, 13, 942 CrossRef CAS PubMed.
- S. Mallakpour and V. Ramezanzade, Int. J. Biol. Macromol., 2020, 162, 512–522 CrossRef CAS PubMed.
- D. C. Souza, S. M. Amorim, R. D. Cadamuro, G. Fongaro, R. A. Peralta, R. M. Peralta, G. L. Puma and R. F. Moreira, Carbohydr. Polym., 2021, 3, 100182 Search PubMed.
- S. Khaiboullina, T. Uppal, N. Dhabarde, V. R. Subramanian and S. C. Verma, Viruses, 2021, 13, 1–15 Search PubMed.
- B. S. Vadlamani, T. Uppal and S. C. Verma, Sensors, 2020, 20, 5871–5881 CrossRef CAS PubMed.
- S. Kumar, M. Karmacharya, S. R. Joshi, O. Gulenko, J. Park, G. H. Kim and Y. K. Cho, Nano Lett., 2020, 21, 337–343 CrossRef PubMed.
- M. S. Stan, I. C. Nica, A. Dinischiotu, E. Varzaru, O. G. Iordache, I. Dumitrescu, M. Popa, M. C. Chifiriuc, G. G. Pircalabioru and V. J. M. Lazar, Mater. Lett., 2016, 9, 789 Search PubMed.
- D. Durgalakshmi, R. A. Rakkesh, S. Rajendran and M. Naushad, Principles and Mechanisms of Green Photocatalysis, Green Photocatalysts, Springer, 2020, vol. 34, pp. 1–24 Search PubMed.
- C. Zhang, Y. Li, D. Shuai, W. Zhang, L. Niu, L. Wang and H. Zhang, Chemosphere, 2018, 208, 84–92 CrossRef CAS PubMed.
- J. C. Sjogren and R. A. Sierka, Appl. Environ. Microbiol., 1994, 60, 344–347 CrossRef CAS PubMed.
- J. Pasquet, Y. Chevalier, J. Pelletier, E. Couval, D. Bouvier and M. A. Bolzinger, Colloids Surf., A, 2014, 457, 263–274 CrossRef CAS.
- A. M. Allahverdiyev, E. S. Abamor, M. Bagirova and M. Rafailovich, Future Microbiol., 2011, 6, 933–940 CrossRef CAS PubMed.
- M. Ahmad, S. J. A. Zaidi, S. Zoha, M. S. Khan, M. Shahid, T. J. Park and M. A. Basit, Colloids Surf., A, 2020, 603, 125200 CrossRef CAS.
- C. Zhang, J. Guo, X. Zou, S. Guo, Y. Guo, R. Shi and F. Yan, Adv. Healthcare Mater., 2021, 2100775 CrossRef CAS PubMed.
- R. Li, T. Chen and X. Pan, ACS Nano, 2021, 15, 3808–3848 CrossRef CAS PubMed.
- Y. Sun, X. Qi, R. Li, Y. Xie, Q. Tang and B. Ren, Opt. Mater., 2020, 108, 110170 CrossRef CAS.
- H. R. Jafry, M. V. Liga, Q. Li and A. R. Barron, Environ. Sci. Technol., 2011, 45, 1563–1568 CrossRef CAS PubMed.
- S. Banerjee, D. D. Dionysiou and S. C. Pillai, Appl. Catal., B, 2015, 176, 396–428 CrossRef.
- P. Ganguly, C. Byrne, A. Breen and S. C. Pillai, Appl. Catal., B, 2018, 225, 51–75 CrossRef CAS.
- R. A. French, A. R. Jacobson, B. Kim, S. L. Isley, L. Penn and P. C. Baveye, Environ. Sci. Technol., 2009, 43, 1354–1359 CrossRef CAS PubMed.
- C. Zhang, Y. Li, D. Shuai, Y. Shen and D. Wang, Chem. Eng. J., 2019, 355, 399–415 CrossRef CAS.
- M. V. Liga, E. L. Bryant, V. L. Colvin and Q. Li, Water Res., 2011, 45, 535–544 CrossRef CAS PubMed.
- Z. D. Bolashikov and A. K. Melikov, Build. Environ., 2009, 44(7), 1378–1385 CrossRef CAS PubMed.
- X. Zuo, X. Chu and J. Hu, Chemosphere, 2015, 136, 118–124 CrossRef CAS PubMed.
- M. Cheng, G. Zeng, D. Huang, C. Lai, P. Xu, C. Zhang and Y. Liu, Chem. Eng. J., 2016, 284, 582–598 CrossRef CAS.
- S. Ede, L. Hafner, P. Dunlop, J. Byrne and G. Will, Photochem. Photobiol., 2012, 88, 728–735 CrossRef CAS PubMed.
- M. Bai, Z. Zhang, Y. Tian and M. J. Bai, J. Air Waste Manag. Assoc., 2012, 62, 393–397 CrossRef CAS PubMed.
- R. Gaupp, N. Ledala and G. A. Somerville, Front. Cell. Infect. Microbiol., 2012, 2, 33 Search PubMed.
- J. Hao, S. Qiu, H. Li, T. Chen, H. Liu and L. Li, Int. J. Food Microbiol., 2012, 155, 99–104 CrossRef CAS PubMed.
- J. A. Byrne, P. S. M. Dunlop, J. W. J. Hamilton, P. F. Ibáñez, I. P. López, P. K. Sharma and A. S. M. Vennard, Molecules, 2015, 20, 5574–5615 CrossRef CAS PubMed.
- C. Zhang, Y. Li, W. Zhang, P. Wang and C. Wang, Chemosphere, 2018, 195, 551–558 CrossRef CAS PubMed.
- J. P. Kaiser, S. Zuin and P. Wick, Sci. Total Environ., 2013, 442, 282–289 CrossRef CAS PubMed.
- K. J. Moor, D. C. Valle, C. Li and J. H. Kim, Environ. Sci. Technol., 2015, 49, 6190–6197 CrossRef CAS PubMed.
- C. Weiss, M. Carriere, L. Fusco, I. Capua, J. A. R. Nava, M. Pasquali, J. A. Scott, F. Vitale, M. A. Unal and C. Mattevi, ACS Nano, 2020, 14, 6383–6406 CrossRef CAS PubMed.
- B. S. Vadlamani, T. Uppal and S. C. Verma, Sensors, 2020, 20, 5871–5881 CrossRef CAS PubMed.
- Y. A. Zeid, N. S. M. Ismail, G. R. Mclean and N. M. Hamdy, Eur. J. Pharm. Sci., 2020, 153, 105465 CrossRef PubMed.
- J. Cheong, H. Yu, C. Y. Lee, J. Lee, H. Choi, J. Lee, H. Lee and J. Cheon, Nat. Biomed. Eng., 2020, 4, 1159–1167 CrossRef CAS PubMed.
- I. D. Jana, P. Kumbhakar, S. Banerjee, C. C. Gowda, N. Kedia, S. K. Kuila, S. Banerjee, N. C. Das, A. K. Das, I. Manna, C. S. Tiwary and A. Mondal, ACS Appl. Nano Mater., 2021, 4, 352–362 CrossRef.
- J. Zhong, E. L. Rosch, T. Viereck, M. Schilling and F. Ludwig, ACS Sens., 2021, 6, 976–984 CrossRef CAS PubMed.
- A. Lafuma and D. Quéré, Nat. Mater., 2003, 2, 457–460 CrossRef CAS PubMed.
- C. W. Lo, C. C. Wang and M. C. Lu, Adv. Funct. Mater., 2014, 24, 1211–1217 CrossRef CAS.
- A. Kumar, V. Soni, P. Raizada and P. Singh, Nanostruct. Photocatal., 2021, 363–387 Search PubMed.
- C. Wang, X. Yang, B. Gu, H. Liu, Z. Zhou, L. Shi, X. Cheng and S. Wang, Anal. Chem., 2020, 92, 15542–15549 CrossRef CAS PubMed.
- H. Liu, E. Dai, R. Xiao, Z. Zhou, M. Zhang, Z. Bai, Y. Shao, K. Qi, J. Tu, C. Wang and S. Wang, Sens. Actuators, B, 2021, 329, 129196 CrossRef CAS PubMed.
- J. V. D. Broek, D. Bischof, N. Derron, S. Abegg, P. A. Gerber, A. T. Gu and S. E. Pratsinis, Anal. Chem., 2021, 93, 1170–1178 CrossRef PubMed.
- A. Kumar, P. Raizada, A. Hosseini-Bandegharaei, V. K. Thakur, V. H. Nguyen and P. Singh, J. Mater. Chem., 2021, 9, 111–153 RSC.
- A. Kumar, P. Raizada, P. Singh, A. H. Bandegharaei and V. K. Thakur, J. Photochem. Photobiol., A, 2020, 397, 112588 CrossRef CAS.
- V. Soni, A. Khosla, P. Singh, V. H. Nguyen, Q. Van Le, R. Selvasembian, C. M. Hussain, S. Thakur and P. Raizada, J. Environ. Manage., 2022, 308, 114617 CrossRef CAS PubMed.
- C. Li, Y. Xu, W. Tu, G. Chen and R. Xu, Green Chem., 2017, 19, 882–899 RSC.
- M. Z. Rahman, M. G. Kibria and C. B. Mullins, Chem. Soc. Rev., 2020, 49, 1887–1931 RSC.
- T. Seifi and A. R. Kamali, Colloids Surf., B, 2020, 199, 111509 CrossRef PubMed.
- O. Akhavan, M. Choobtashani and E. Ghaderi, J. Phys. Chem. C, 2012, 116, 9653–9659 CrossRef CAS.
- R. Cheng, L. J. Shen, J. H. Yu, S. Y. Xiang and X. Zheng, Catalysts, 2018, 8, 406 CrossRef.
- M. V. Liga, S. J. M. Boyle, H. R. Jafry, A. R. Barron and Q. Li, Environ. Sci. Technol., 2013, 47, 6463–6470 CrossRef CAS PubMed.
- C. Zhang, M. Zhang, Y. Li and D. Shuai, Appl. Catal., B, 2019, 248, 11–21 CrossRef CAS.
- Y. Li, C. Zhang, D. Shuai, S. Naraginti, D. Wang and W. Zhang, Water Res., 2016, 106, 249–258 CrossRef CAS PubMed.
- E. Aliyev, V. Filiz, M. M. Khan, Y. J. Lee, C. Abetz and V. Abetz, Nanomaterials, 2019, 9, E1180 CrossRef PubMed.
- D. Ege, A. R. Kamali and A. R. Boccaccini, Adv. Eng. Mater., 2017, 19, 1700627 CrossRef.
- B. Ziem, W. Azab, M. F. Gholami, J. P. Rabe, N. Osterrieder and R. Haag, Nanoscale, 2017, 9, 3774–3783 RSC.
- S. Ye, K. Shao, Z. Li, N. Guo, Y. Zuo, Q. Li, Z. Lu, L. Chen, Q. He and H. Han, ACS Appl. Mater. Interfaces, 2015, 7(38), 21571–21579 CrossRef CAS PubMed.
- B. Ziem, H. Thien, K. Achazi, C. Yue, D. Stern, K. Silberreis, M. F. Gholami, F. Beckert, D. Gröger and R. Mülhaupt, Adv. Healthcare Mater., 2016, 5, 2922–2930 CrossRef CAS PubMed.
- M. G. Stanford, J. T. Li, Y. Chen, E. A. McHugh, A. Liopo, H. Xiao and J. M. Tour, ACS Nano, 2019, 13, 11912–11920 CrossRef CAS PubMed.
- J. Huang, W. Ho and X. Wang, Chem. Commun., 2014, 50, 4338–4340 RSC.
- Y. Orooji, M. Ghanbari, O. Amiri and M. S. Niasari, J. Hazard. Mater., 2020, 389, 122079 CrossRef CAS PubMed.
- Y. Li, C. Zhang, D. Shuai, S. Naraginti, D. Wang and W. Zhang, Water Res., 2016, 106, 249–258 CrossRef CAS PubMed.
- C. Zhang, M. Zhang, Y. Li and D. Shuai, Appl. Catal., B, 2019, 248, 11–21 CrossRef CAS.
- J. H. Thurston, N. M. Hunter, L. J. Wayment and K. A. Cornell, J. Colloid Interface Sci., 2017, 505, 910–918 CrossRef CAS PubMed.
- I. Maqbool, F. Rehman, F. Soomro, Z. Bhatti, U. Ali, A. H. Jatoi, B. Lal, M. Iqbal, S. Phulpoto and A. Ali, ChemBioEng Rev., 2021, 8, 67–77 CrossRef CAS.
- F. Ghaemi, A. Amiri, M. Y. Bajuri, N. Y. Yuhana and M. Ferrara, Sustain. Cities Soc., 2021, 72, 103046 CrossRef PubMed.
- B. Anasori, M. R. Lukatskaya and Y. Gogotsi, Nat. Rev. Mater., 2017, 2, 1–17 Search PubMed.
- G. Deysher, C. E. Shuck, K. Hantanasirisakul, N. C. Frey, A. C. Foucher, K. Maleski, A. Sarycheva, V. B. Shenoy, E. A. Stach and B. Anasori, ACS Nano, 2019, 14, 204–217 CrossRef PubMed.
- N. Dwivedi, C. Dhand, P. Kumar and A. Srivastava, Adv. Mater., 2021, 2, 2892–2905 RSC.
- J. Li, Z. Li, X. Liu, C. Li, Y. Zheng, K. W. K. Yeung, Z. Cui, Y. Liang, S. Zhu and W. Hu, Nat. Commun., 2021, 12, 1–10 CrossRef PubMed.
- Q. S. Rao, S. Y. Liao, X. W. Huang, Y. Z. Li, Y. D. Liu and Y. G. Min, Polymers, 2020, 12, 2192 CrossRef CAS PubMed.
- S. Bhattacharjee, R. Joshi, A. A. Chughtai and C. R. Macintyre, Adv. Mater. Interfaces, 2019, 6, 1900622 CrossRef CAS PubMed.
- Y. Li, S. Huang, C. Wei, C. Wu and V. N. Mochalin, Nat. Commun., 2019, 10, 1–8 CrossRef PubMed.
- Y. Dong, S. Chertopalov, K. Maleski, B. Anasori, L. Hu, S. Bhattacharya, A. M. Rao, Y. Gogotsi, V. N. Mochalin and R. Podila, Adv. Mater., 2018, 30, 1705714 CrossRef PubMed.
- A. Adalja and T. Rev, Expert Rev. Anti Infect. Ther., 2019, 17, 467–470 CrossRef CAS PubMed.
- H. Cai, Y. L. Huang and D. Li, Coord. Chem. Rev., 2019, 378, 207–221 CrossRef CAS.
- S. Lu, G. Meng, C. Wang and H. Chen, Chem. Eng. J., 2021, 404, 126526 CrossRef CAS PubMed.
- P. Li, J. Li, X. Feng, J. Li, Y. Hao, J. Zhang, H. Wang, A. Yin, J. Zhou, X. Ma and B. Wang, Nat. Commun., 2019, 10, 1–10 CrossRef PubMed.
- K. Li, P. Chen, J. Li, Y. Sun, Y. Chu and F. Dong, Catal. Sci. Technol., 2018, 8, 4600–4603 RSC.
- J. Zhang, P. Li, X. Zhang, X. Ma and B. Wang, ACS Appl. Mater. Interfaces, 2020b, 12, 46057–46064 Search PubMed.
- M. M. Almaraz, R. Gref, V. Agostoni, C. Kreuz, P. Clayette, C. Serre, P. Couvreur and P. Horcajada, J. Mater. Chem. B, 2017, 5, 8563–8569 RSC.
- M. C. Scicluna and L. V. Zarb, ACS Appl. Nano Mater., 2020, 3, 3097–3115 CrossRef CAS.
- P. Li, J. Li, X. Feng, J. Li, Y. Hao, J. Zhang, H. Wang, A. Yin, J. Zhou and X. Ma, Nat. Commun., 2019, 10, 1–10 CrossRef PubMed.
- Y. Yu, F. Bu, H. Zhou, Y. Wang, J. Cui, X. Wang, G. Nie and H. Xiao, Mater. Chem. Front., 2020, 4, 930–1953 Search PubMed.
- F. Ghaemi, A. Amiri, M. Y. Bajuri, N. Y. Yuhana and M. Ferrara, Sustain. Cities Soc., 2021, 72, 103046 CrossRef PubMed.
- S. Rahimi, A. Poormohammadi, B. Salmani, M. Ahmadian and M. Rezaei, J. Water Reuse Desalin., 2016, 6, 574–582 CrossRef CAS.
- M. Abdennouri, M. Baâlala, A. Galadi, M. El Makhfouk, M. Bensitel, K. Nohair, M. Sadiq, A. Boussaoud and N. Barka, Photocatalytic degradation of pesticides by titanium dioxide and titanium pillared purified clays, Arabian J. Chem., 2016, 9, S313–S318 CrossRef CAS.
- A. J. Galante, S. Haghanifar, E. G. Romanowski, R. M. Q. Shanks and P. W. Leu, Superhemophobic and Antivirofouling Coating for Mechanically Durable and Wash-Stable Medical Textiles, ACS Appl. Mater. Interfaces, 2020, 12(19), 22120–22128 CrossRef CAS PubMed.
- S. Suresha, A. Suresh, S. Katti and J. Verma, J. Indian Chem. Soc., 2020, 97, 2623–2632 Search PubMed.
- S. Kumar, M. Karmacharya, S. R. Joshi, O. Gulenko, J. Park, G. H. Kim and Y. Cho, Nano Lett., 2020, 21, 337–343 CrossRef PubMed.
- E. Horváth, L. Rossi, C. Mercier, C. Lehmann, A. Sienkiewicz and L. Forró, Adv. Funct. Mater., 2020, 30, 2004615 CrossRef PubMed.
- S. M. Zacarías, A. Manassero, S. Pirola, O. M. Alfano and M. P. Satuf, Environ. Sci. Pollut. Res. Int., 2021, 28, 23859–23867 CrossRef PubMed.
- Q. Li, Y. Yin, D. Cao, Y. Wang, P. Luan, X. Sun, W. Liang and H. Zhu, ACS Nano, 2021, 15, 11992–12005 CrossRef CAS PubMed.
|
This journal is © The Royal Society of Chemistry 2022 |