DOI:
10.1039/D1RA08935H
(Paper)
RSC Adv., 2022,
12, 2300-2309
On crystallization of water confined in liposomes and cryoprotective action of DMSO
Received
8th December 2021
, Accepted 10th January 2022
First published on 14th January 2022
Abstract
In this work, the phase behavior of cryoprotective mixtures based on dimethyl sulfoxide (DMSO) mixed with a lipid bilayer consisting of dimyristoyl phosphatidylcholine (DMPC) was studied. This system represented a model of a biological cell and its membrane. The aim of the work was to clarify the origin of the cryoprotective action of low-concentrated mixtures (1–10 vol%) DMSO in water, representing mixtures used in cryopreservation in cell therapy. The combination of experimental techniques of differential scanning calorimetry (DSC) and positron annihilation lifetime spectroscopy (PALS) allowed a study of crystallization behavior of water confined in liposomes imitating the intracellular environment. The ability of liposomes to show the fundamental aspects of water phase behavior seen during freezing of biological cells was proved. The presence of an amorphous freeze-concentrated phase of DMSO in the frozen state was confirmed and its possible crystallization into the DMSO trihydrate and ice during thawing was demonstrated. Correlation between the critical temperature range for the loss of cell viability during slow thawing and the temperatures of freeze-concentrated phase crystallization was found. Based on this finding, possible mechanisms of DMSO cryoprotection are discussed with support brought by results for the studied model system. Quantification of the ice phase fraction in the frozen mixtures revealed that even low concentrations of DMSO can induce a considerable decrease in the amount of ice present.
Introduction
Cryopreservation of cells allows a long-term storage without a risk of degradation since low temperatures practically stop the biochemical processes occurring in the cells.1 Cryopreservation has a wide application in medicine and research – applied or fundamental.2 There are several stresses acting on cells during freezing or thawing, mainly ice crystallization, cell dehydration, increased salt concentrations or ice recrystallization by which integrity of biological membranes or macromolecular complexes is threatened.3–6 In the 1950s, it was found that after addition of dimethyl sulfoxide (DMSO) into a cell freezing medium, cell post-thaw viability increased.7 Currently, there are several successful slow cooling protocols (1–5 K min−1) being used in cryopreservation of cells with therapeutic application, typically containing 5 to 10 vol% DMSO in the cell medium.8 Some studies report even lower concentrations of DMSO successfully applied in cryopreservation of cell therapy products – 2.2 vol%9 or 2.5 vol%.10 Cell post-thaw viability depends on cryoprotectant concentration,11 with a higher amount of DMSO securing higher cell viability, but on the other hand introducing its own toxicity regarding specialized cellular functions or toxicity for the human body when cells are applied to a patient during cell therapy.12,13 Thus, an optimization of cryopreservation protocols is needed.
Hypothesized mechanisms of cryoprotective action are often ascribed to the ability of DMSO to colligatively reduce the ice formation, cell dehydration, salt concentration4 or increase the membrane stability against these stresses,14 but complete understanding of this action at molecular level is still missing. Stresses during freezing or thawing of cells relate to water phase transitions. Water can exist in either crystalline or amorphous form, while the latter is thought to be harmless regarding cell survival. On the other hand, intracellular ice crystallization is thought to cause cryoinjury leading to the loss of cell viability.15–17 DMSO can form an amorphous fraction in the freezing mixture with water, which allows intracellular vitrification.18,19 It was shown that this amorphous fraction consisting of freeze-concentrated DMSO is metastable and can crystallize during slow thawing, threatening cell viability.18
In this work, the solidification and melting processes of water–DMSO mixtures containing liposomes were studied. Liposomes are supramolecular aggregates of lipid molecules aligned into a lipid bilayer. Lipid bilayer represents the structural basis of biological membranes. Integrity of plasma membrane or other internal membranes is critical for cell survival and function. Liposomes are closed structures confining water, which can serve as a model of intracellular water. Liposomes serve as model systems of a biological cell and its membrane. Knowledge of physico-chemical properties of intracellular water is critical for predictions of crystallization temperature, physical state of intracellular water during freezing or thawing, water transport through plasma membrane or other properties relevant for optimization of cryopreservation protocols.20
In this work, the combination of experimental methods of differential scanning calorimetry (DSC) and positron annihilation lifetime spectroscopy (PALS) is applied. Combination of these experimental techniques has been proven to be suitable for studying the phase behavior of various substances and mixtures.18,21,22 PALS is an experimental method of nuclear physics using the triplet bound state of positron and electron – ortho-positronium (o-Ps) – as a probe for detecting microstructural changes in the studied materials during variations of external parameters such as temperature, pressure or time. The advantage of the PALS method is high sensitivity of the o-Ps lifetime to changes in microstructure related to phase transitions or other structural rearrangements.23,24 Combination of these experimental methodologies in this work enabled the investigation of the phase behavior of water confined in liposomes and verification of the presence of the amorphous freeze-concentrated phase formed by DMSO and its temperature and time stability.
Experimental
Materials and methods
The anhydrous 99.9% dimethyl sulfoxide (DMSO, C2H6OS) from Sigma-Aldrich and pure water LC-MS Ultra CHROMASOL V from Honeywell/Riedel-de Haen were used to prepare binary mixtures of interest. Dimyristoyl phosphatidylcholine (DMPC, C36H72NO8P) purchased from Sigma-Aldrich was used as only component of liposomes.
Concentrations of DMSO in the mixtures with water are given volumetrically, defined as the ratio of volumes of pure substances (VDMSO/(VDMSO + Vwater)) × 100%. Concentration of DMSO in the studied mixtures was in the range of 1 to 10 vol%, which were prepared by mixing at standard laboratory temperature and pressure.
To obtain multilamellar liposomes, DMPC lipid was hydrated directly as a powder. Care was taken to homogenize samples – with use of ultrasound (1 MHz) for 45 min. Final mixtures contained 5 mg ml−1 of DMPC per volume of studied water–DMSO mixture. Spontaneously formed lipid structures were mostly multilamellar liposomes with size ranging from 200 nm to 5 μm. The size of liposomes was measured by dynamic light scattering (DLS) technique using the Zetasizer Nano ZS90 (Malvern Instruments, UK) at 25 °C. Optics with scattering detector angle of 90° was used. The refraction indices and viscosities of aqueous solutions (water–DMSO mixtures) containing liposomes needed for the correct size determination were taken from published data.25
Unilamellar liposomes were prepared as follows: at first, the DMPC was dissolved in chloroform, then dried with gaseous N2 and after 24 h evaporation in vacuum chamber, it was hydrated by a mixture of water–DMSO. In order to obtain unilamellar liposomes, samples were then extruded through the 200 nm polycarbonate membranes with Mini Extruder from Avanti. Presence of DMSO (2.2 and 10 vol%) did not significantly alter the size of extruded liposomes. All mixtures contained liposomes with diameter of about 200 nm and polydispersity index about 0.1.
Differential scanning calorimetry
Calorimetric analysis of investigated samples was performed by differential scanning calorimetry (DSC) using power-compensated calorimeter PerkinElmer DSC8500 equipped with IntraCooler II. Samples were encapsulated in aluminum pans at standard pressure and nitrogen was used as dynamic atmosphere with flow of 20 ml min−1. Weight of encapsulated samples was about 25 mg. Thermal scans were performed with the cooling rate of 5 K min−1 and heating rate of 1 K min−1, if not stated otherwise. In the case of crystallization, characteristic temperature is given by extrapolated onset of heat flow peak, while in the case of melting, it is the peak maximum. Enthalpic change during phase transition was determined by integrating an area under the heat flow curve against the hypothetical sigmoidal baseline.
Positron annihilation spectroscopy
22Na was used as a positron source with an activity of about 1 MBq. It was put between two identical pieces of the sample (“sandwich” geometry). The sample container was connected to the cooling part of cryo-generator and fixed between two detectors of PALS spectrometer. The time resolution function was determined by Si defect-free sample and it was about 320 ps (FWHM). Temperature measurements in the range of 100–300 K were carried out by the closed cycle He-refrigerator JANIS CCS-450 System with temperature stability of ±0.2 K. The hermetically closed sample containers were kept under the vacuum during temperature experiments. PALS data were analyzed by freely available LT Polymers software,26 details of this program can be found in the paper of its author.27 Three component analysis was performed. The shortest component was attributed to the para-positronium (p-Ps) annihilation with fixed value of 125 ps, the medium component to a direct annihilation without Ps formation and the longest lifetime component was attributed to a pick-off annihilation of ortho-positronium (o-Ps). The pick-off annihilation is the process, when the positron from o-Ps annihilates with an electron from the surrounding material what shortens the o-Ps lifetime depending on the local arrangement of surrounding atoms or molecules. The ratio of p-Ps and o-Ps intensities was fixed to 1
:
3.
Calculations of water activity and unfrozen fraction of water
Thermodynamical activity of water (aW) was calculated from the melting point depression data by following relationship (for full derivation see book by Tosun):28 |
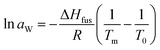 | (1) |
where ΔHfus is enthalpy of fusion of pure ice – 333.5 J g−1,29 Tm is actual melting point of ternary mixture, R is the gas constant and T0 is the normal melting point of ice.
Unfrozen fraction of water describes the portion of water in the mixture that did not crystallize during solidification. It is calculated by relationship:
|
 | (2) |
where Δ
Hexp stands for experimentally determined enthalpic change during the ice melting, normalized to the water weight in a mixture. Δ
H is the heat of fusion of pure ice.
Results and discussion
Phase behavior of water in a mixture with liposomes
During freezing of multilamellar liposomes in water, two exothermic events with onset temperatures of 258 K and 235 K were observed (black line in Fig. 1). Since the lipid bilayer consisting of DMPC has no phase transitions in this temperature range reported,30 both of these events are attributed to crystallization of water. The crystallization with onset of 258 K resembles supercooling of water without a lipid bilayer (at same experimental conditions) and the temperature of 235 K is close to the reported temperature of homogeneous nucleation of ice.31 Liposomes are closed objects confining a certain amount of water that can diffuse through the lipid bilayer but it is not in direct contact with extraliposomal water surrounding these structures. The structure of liposomes in this part of study was multilamellar, what means that water confined inside of the liposomes is separated from the outside water by several lipid bilayers. The presence of the crystallization near the temperature of homogeneous nucleation indicates that it is the confinement of water brought by liposomes what allows for such extreme supercooling. This interpretation is supported by absence of this low-temperature crystallization for pure water under the same experimental conditions (data not shown). Crystallization at 258 K then corresponds to the crystallization of the water surrounding the liposomes, which forms the dispersion medium. Similar phase behavior was reported for cell suspensions during the freezing,32 where first to crystallize was the extracellular water after some supercooling below the equilibrium freezing point of a cell medium. Usually, intracellular water requires greater supercooling than extracellular water for crystallization to begin, with onset temperature being cell type dependent.6
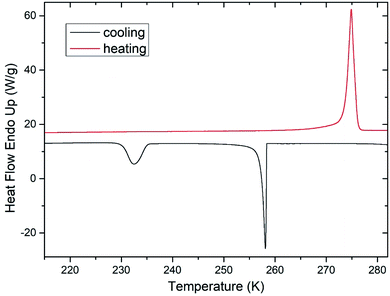 |
| Fig. 1 DSC thermogram showing the phase behavior of water during freezing and thawing of multilamellar liposomes in pure water. Both heating and cooling rates were 5 K min−1 and lipid content is 40 wt%. | |
These results indicate the adequacy of the model system used in this study as liposomes show all fundamental features of the water phase behavior in biological cells. Qualitatively the same water phase behavior was observed for mixtures of multilamellar liposomes containing DMSO as well – the two-step crystallization during cooling was detected for every studied mixture, but with altered quantitative characteristics of water phase transitions.
Effect of DMSO on water phase behavior in a mixture with liposomes
After the addition of DMSO into a mixture, depression of ice melting temperature was observed (Fig. 2). The melting temperature of ice decreased with increasing DMSO concentration – a drop-down to temperature of about 268 K for 10% DMSO was observed. Similar manifestation was observed for the crystallization of water confined in liposomes – DMSO induced depression of crystallization temperature from 235 K for water down to 228 K for 10% DMSO. Melting point depression of ice is caused by lowered chemical potential of liquid water, which is induced by presence of DMSO. In the work of Wang et al.,33 it was shown that same mechanism is responsible for the depression of homogeneous nucleation temperature of ice as well. Dependence of the water crystallization temperature in liposomes on the DMSO concentration is similar to the results obtained with emulsified binary water–DMSO mixtures,34 where emulsification allowed extreme supercooling of mixtures to homogeneous nucleation temperature of ice. This suggests that the crystallization of water confined in liposomes is also triggered by homogeneous nucleation. Thus, the DMPC lipid bilayer does not significantly affect the temperature of crystallization, what indicates its inefficiency as a catalyst for ice nucleation. Results of this study for the influence of DMSO on crystallization temperature in liposomes are even in relatively good agreement with the work of Körber et al.,35 where water phase behavior was studied in cell suspensions containing human lymphocytes with varied amount of DMSO in cell cryopreservation medium. Authors observed a depression of intracellular ice crystallization temperature as a result of an increase in DMSO concentration in the cell medium – for 2% DMSO it was 232 K and for 10% DMSO about 227 K. Slightly lower temperatures compared to our results can be explained by additional depression due to other solutes present in intracellular environment beyond DMSO (inorganic salts, proteins etc.).
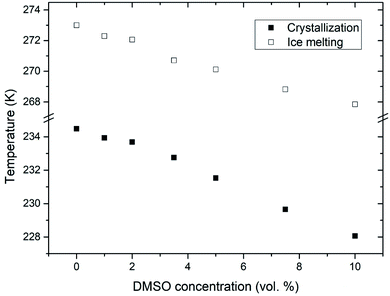 |
| Fig. 2 Melting point depression and depression of crystallization temperature of ice inside the liposomes induced by DMSO. | |
Fig. 3 shows calculated values of water activity and unfrozen fraction of water based on the eqn (1) and (2), respectively. Increasing the concentration of DMSO in a mixture, had as a result decrease of the water activity – as expected from the melting point depression data. Unfrozen fraction of water increased with DMSO concentration, what means decrease in amount of ice present. During the crystallization of ice in water–DMSO mixtures, there is an ice-free phase separating from the ice crystals that is still liquid. It consists of a freeze-concentrated phase of DMSO in water. Separation of this phase occurs during the formation of ice, since DMSO is excluded from the ice crystal lattice.36–38 Concentration of DMSO in this ice-free phase increases up to the eutectic concentration, when there is a maximum depression in the ice freezing point in a binary water–DMSO mixture.39 Eutectic concentration is close to the composition given by a molecular ratio of 3 DMSO
:
1 H2O, what is related to energetically favorable intermolecular interactions.40 This is also confirmed by the negative deviation of the actual freezing point of ice from the one dictated by the ideal mixture behavior,34 which does not take into account the molecular interactions of water with DMSO. These interactions reduce the amount of “free” water in the mixture. This also results in a reduction in the amount of crystalline ice phase with proportionality to the concentration of DMSO in the mixture, what is exactly the result plotted in Fig. 3. Correlation between the unfrozen fraction (water plus solutes) in the freezing medium and the viability of erythrocytes was found – the higher unfrozen fraction corresponding to the higher viability, with a critical dependence on this parameter.31 Increase of the unfrozen fraction (at constant temperature) is associated with an increase in DMSO concentration in the medium. Presence of the unfrozen fraction (ice-free phase) obviously reduces the likelihood of direct contact between extracellular ice crystals and the plasma membrane during freezing, which may prevent the nucleation of intracellular ice41 and/or mechanical damage to the plasma membrane. The physical state and the amount of unfrozen fraction that depends on the concentration of the cryoprotective substance may therefore be behind the concentration dependence of the cryoprotective effect of DMSO in low-concentrated mixtures up to 10 vol%, which are often used for cryopreservation of cell therapy products. This idea is supported by the following part of this study conducted on unilamellar liposomes.
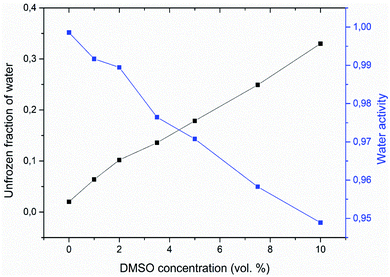 |
| Fig. 3 Double Y-axis showing the unfrozen fraction of water (black) and corresponding water activity (blue) for different concentrations of DMSO in water with multilamellar liposomes. | |
Unilamellar liposomes
Calorimetric study of 200 nm unilamellar liposomes showed a qualitative difference of the water freezing behavior when it was only component hydrating liposomes and when in mixture with DMSO. For unilamellar liposomes in pure water, low-temperature crystallization is absent (Fig. 4). Water confined in unilamellar liposomes appears to undergo crystallization at a higher temperature together with water surrounding the liposomes. However, for unilamellar liposomes in a mixture of water and DMSO, the low-temperature crystallization was observed for every studied concentration of DMSO. We interpret these results in a way that single lipid bilayer does not represent a sufficient barrier against the ice crystallization front. Integrity of lipid bilayer is breached by extraliposomal ice, what results in crystallization of intraliposomal ice. On the other hand, when DMSO is present in the solution, it forms the ice-free phase that physically surrounds the lipid bilayer. This ice-free phase thus forms a protective layer that is still liquid while the probability of contact between extraliposomal ice and the lipid bilayer is reduced. While single lipid bilayer is not a sufficient barrier to stop the ice crystallization front, multiple lipid bilayers in multilamellar liposomes are. This is evidenced by the fact that in the case of multilamellar liposomes, the low-temperature crystallization near homogeneous nucleation temperature was observed in every case – whether the medium contained DMSO or was made up only by water (Fig. 1 and 2).
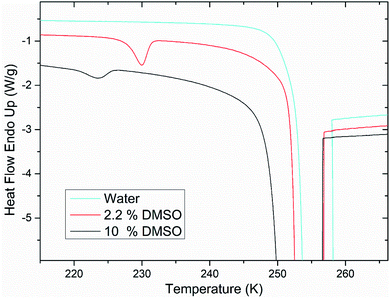 |
| Fig. 4 DSC thermograms showing a qualitative difference of the water freezing behavior inside the unilamellar liposomes. In the case of pure water, there is no low-temperature crystallization. Peaks cut off in the range of 250–260 K are associated with the crystallization of water surrounding liposomes. | |
This qualitative difference in the water phase behavior induced by addition of DMSO into the solution containing unilamellar liposomes is similar to the effect observed in biological cells when a cryoprotective substance (DMSO and glycerol) caused significant depression of the intracellular ice crystallization temperature from about 261 K to 238 K for eight-cell mouse embryos,42 from 261 K to 233 K for bovine and hamster oocytes43 or from 243 K to 228 K for human lymphocytes.32 This effect is so pronounced (depression of the equilibrium freezing point in these cases is at most few degrees celsius, see the ice melting temperature in Fig. 2), that cryoprotective substances are thought to induce a qualitative change in the nucleation mechanism of intracellular ice crystallization: low-temperature crystallization is thought to be triggered by heterogeneous nucleation by intracellular macromolecules, which represent thermodynamically “weaker” nucleation centers,44 while nature of the nucleation mechanism at higher temperatures is more questionable. It is known that for this kind of intracellular ice nucleation to take place, physical contact of the plasma membrane with extracellular ice is necessary.41,45,46 There are several hypotheses on how this nucleation can occur. One is, that nucleation of intracellular ice occurs through protein pores in the plasma membrane.47 Different hypothesis “plasma membrane catalyzed ice nucleation”,44 describes the indirect role of extracellular ice, which induces structural changes of the membrane that makes it an effective ice nucleator on the intracellular side – but possible mechanisms are speculative and have not been elucidated. Another obvious hypothesis assumes a loss of plasma membrane integrity when extracellular ice is present, what allows intracellular ice nucleation. When it comes to liposomes in our study, protein pores are not present in the simple lipid bilayer studied, so this explanation is not needed. Compared to “plasma membrane catalyzed nucleation”, it is easier to hypothesize that the nucleation of ice crystallization in liposomes at higher temperatures is a direct consequence of loss of the lipid bilayer integrity. The ability of DMSO to prevent this process can be explained by the fact that the ice-free phase separating during ice crystallization – consisting of a freeze-concentrated DMSO – forms a protective layer of lipid bilayer during the freezing. When the medium does not contain DMSO, ice-free phase is not present and ice comes into a direct contact with the lipid bilayer, what ultimately leads to a breach in its integrity and the nucleation of the intraliposomal ice.
Relevance of this result to cellular systems lies in the significance of the ice-free phase for safe freezing and thawing of cells. During the freezing, freeze-concentrated phase of DMSO is liquid at higher subzero temperatures. This phase eventually can vitrify at lower temperatures, what is important for an intact biological membrane. The temperature and time stability of the amorphous freeze-concentrated phase against possible crystallization was also examined with results shown in the following section.
Phase behavior of freeze-concentrated phase of DMSO
Positron annihilation lifetime spectroscopy allowed an observation of temperature and time dependence of the ortho-positronium (o-Ps) lifetime in mixtures of water and DMSO with unilamellar liposomes. o-Ps is a probe sensitive to microstructural changes related to phase transitions or other structure rearrangements. The temperature measurements consisted of stepwise heating and cooling with isothermal measurements (1 h) at different temperatures in the temperature range of 100 to 300 K. At first, a sample was (continuously) cooled from room temperature down to the temperature of 100 K with the cooling rate of 1.9 K min−1 without any measurement taking place. Then the temperature cycle started with the stepwise heating up to 300 K and measurements taking place. After completion of the heating to 300 K, measurements were taken during the stepwise cooling down to 100 K. The results of selected concentrations of 5 and 10% DMSO in water with lipid bilayer DMPC are shown in Fig. 5. At about 270 K a sharp increase of the o-Ps lifetime with rising temperature was observed, this phenomenon, which is related to the melting of ice,48 was observed for both studied mixtures. Similarly, during cooling, there is a sharp decrease of o-Ps lifetime at somewhat lower temperatures compared to the case of melting, what is related to a certain degree of supercooling before the onset of crystallization. The gradual increase of the o-Ps lifetime in the range of 150 K to 250 K with temperature is the feature of pure water (ice) in this temperature range.48 This effect is described by a capture of o-Ps in temperature-created defects of the crystalline ice structure, mainly vacancies.49,50 Deviation from this trend was observed in temperature range of 165 K to 210 K in mixtures with DMSO. During the stepwise cooling, higher o-Ps lifetimes were observed in this temperature range, what indicates a less ordered structure – presence of a supercooled liquid. During the stepwise heating that followed the initial cooling of the sample to 100 K from room temperature, the o-Ps lifetime dropped down during the heating above 160 K, indicating a tighter arrangement of the local structure, what can be a result of (re)crystallization. This is a sign of at least partially amorphous system formed during freezing. During thawing, there is relatively sharp, statistically significant increase of the o-Ps lifetime when the sample is heated from 205 to 210 K for both 5 and 10% DMSO content with lipid bilayer (Fig. 6 – enlarged portion of critical temperature range). Temperature of 210 K perfectly agrees with the reported melting temperature of the DMSO trihydrate – ice eutectic mixture.39,51 Existence of the crystalline DMSO trihydrate was recently confirmed experimentally with identification of its crystal structure.52 We therefore consider the decrease in the o-Ps lifetime in the range of 165 to 200 K to be caused by crystallization of DMSO-trihydrate and ice from amorphous freeze-concentrated phase of DMSO generated during freezing. Subsequent increase in o-Ps lifetime at 210 K is then related to the melting of this eutectic mixture. In the past, it was shown that in a two-component water–DMSO mixture (without lipid bilayer), slow heating during the thawing led to a decrease of the o-Ps lifetime in the critical temperature range of 165 K to 200 K, as long as quick heating suppressed the drop-down of o-Ps lifetime, what was interpreted as avoiding the crystallization.18 There is a work,53 where a positive correlation was found between the cell post-thaw viability and the heating rate through the temperature range of 180 K to 200 K during thawing, what matches critical temperature range for crystallization of water–DMSO eutectic mixture observed in our experiments. It is known that during slow (equilibrium) cooling, biological cells are directly surrounded by the freeze-concentrated phase of cryoprotective agents and other dissolved substances in cryopreservation cell medium (inorganic salts, etc.).4,36,54 DMSO is present in intracellular compartment as well, so the cell interior is also filled with this freeze-concentrated phase. It is therefore obvious that it is crucial to know in what state—crystalline or amorphous—this concentrated phase exists during the freezing and thawing regarding the cell viability.
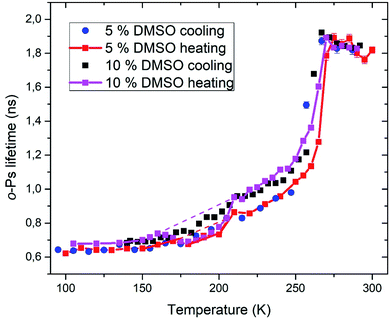 |
| Fig. 5 Temperature dependence of ortho-positronium lifetime for 5 and 10% DMSO with unilamellar liposomes. | |
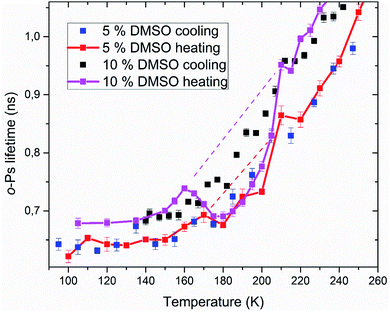 |
| Fig. 6 Enlarged portion of Fig. 5 showing the critical temperature range where crystallization of the amorphous phase occurs during thawing. | |
PALS method also allows relative quantification of the amount of amorphous phase in the frozen system. Amorphous fraction is proportional to the degree of hysteresis (Table 1) between the o-Ps lifetime during thawing and the hypothetical dependence of the o-Ps lifetime during freezing (dashed line in Fig. 5 and 6). This hypothetical dependence of the o-Ps lifetime corresponds to the mixture of ice and completely amorphous freeze-concentrated phase of DMSO in the critical temperature range where crystallization occurs during slow thawing. The adequacy of this description results from our previous work with the two-component water–DMSO mixture, where higher o-Ps lifetimes were observed as long as the heating rate was higher through this critical temperature range when o-Ps lifetimes followed the values measured during freezing.18 In this sense, the hypothetical temperature dependence of the o-Ps lifetime can be perceived as a limit for high heating rates, when no crystallization of freeze-concentrated phase occurs. The size of the hysteresis shows that the amount of amorphous phase is proportional to the DMSO concentration in the mixture, what also matches the result of the DSC analysis for the unfrozen fraction of water (Fig. 3).
Table 1 Quantitative characteristics of o-Ps temperature dependence. Hysteresis area between o-Ps lifetime dependencies during freezing and thawing HC/H, hysteresis area between o-Ps lifetime during thawing and hypothetical dependency during freezing HChyp/H (corresponding to mixture of ice and fully amorphous freeze-concentrated phase of DMSO), onset of crystallization of amorphous phase during thawing TC and temperature of completed crystallization TCend
Sample |
HC/H (ns K) |
HChyp/H (ns K) |
TC (K) |
TCend (K) |
5% DMSO |
0.67 |
1.99 |
172 ± 2 |
180 ± 2 |
10% DMSO |
2.53 |
4.92 |
163 ± 2 |
177 ± 2 |
Analyzed PALS spectra carry another information besides the lifetime of positron(ium). Every lifetime has a corresponding intensity. In Fig. 7, the temperature dependence of positronium formation intensity is shown. Its value corresponds to the percentual ratio of annihilation events by ortho- and para-positronium, the rest being made up by direct annihilations of positron with electron without Ps formation. During the ice melting and crystallization, there is an abrupt decrease and increase in Ps formation respectively (250–270 K) for both 5 and 10% DMSO with lipid bilayer (Fig. 7), what mirrors the trends observed on o-Ps lifetimes. For 10% DMSO, there is also significant hysteresis between Ps formation intensity during cooling and heating measurements in the temperature range of 155–210 K, similar to the hysteresis seen for the o-Ps lifetimes in Fig. 5. The “V-shaped” temperature dependence of Ps formation intensity during the heating with vertex at about 160 K is obviously linked to devitrification of the amorphous freeze-concentrated phase of DMSO and its crystallization into the DMSO trihydrate and ice. For 5% DMSO with lipid bilayer, size of the hysteresis between cooling and heating is less pronounced, what is caused by lower amount of DMSO in the mixture and thus lower amorphous fraction. Globally higher values of Ps formation intensity for 5% DMSO can be linked to higher fraction of crystalline ice in the system. This trend was also observed for the binary water–DMSO mixtures studied by PALS.18
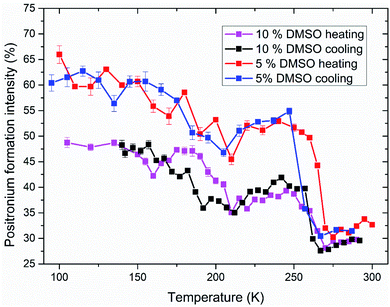 |
| Fig. 7 Intensity of positronium formation in studied systems of 5 and 10% DMSO with unilamellar liposomes during freezing and thawing. Values corresponding to the o-Ps lifetimes shown in Fig. 5 and 6. | |
Time stability of amorphous fraction
Using the PALS method, a time stability of the amorphous freeze-concentrated phase was also studied. Isothermal time measurements at the temperature of 180 K were carried out. This temperature corresponds to the minimum of o-Ps lifetime seen in temperature measurement, what is considered to be the state of completed crystallization of DMSO trihydrate and ice during thawing (Table 1). Results of these time measurements are shown in Fig. 8. The time dependence of the o-Ps lifetime at 180 K in the system consisting of lipid bilayer and 10% DMSO demonstrated relative stability of the amorphous phase for at least 12 hours as long as the system was cooled from room temperature to a final temperature of 180 K with the cooling rate of 1.9 K min−1. In another variant of this experiment – cooling from the room temperature to 70 K, subsequent quick heating to 180 K followed by the isothermal time measurement – value of o-Ps lifetime was initially comparable to the previous variant of the experiment, however after 2–3 hours it decreased to the minimum value of about 0.7 ns seen in temperature measurements at the same temperature. This phenomenon confirms the interpretation of the temperature measurements that during initial cooling through the critical temperature range of 165–210 K, the ice-free phase consisting of freeze-concentrated DMSO enters an amorphous state. The crystallization of this phase then occurs during the heating of the mixture above the glass transition temperature, which is in the case of 10% DMSO about 150 K.55
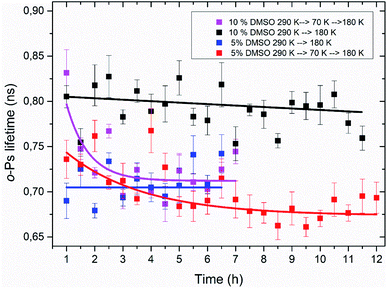 |
| Fig. 8 Time dependence of o-Ps lifetime at constant temperature 180 K with different thermal history of 5 and 10% DMSO mixtures with unilamellar liposomes. Thermal history is described in the text. Lines are meant only as guideline for an eye. | |
In the case of lipid bilayer with 5% DMSO system, a less pronounced difference between the two variants of the isothermal experiment was observed – especially during the first 4 hours at 180 K, a significant difference between the two dependencies is lacking. Later, for times of more than 5 h for the variant “cooling to 70 K and then heating to 180 K”, a certain decrease of the o-Ps lifetime was observed, that may correspond to the process of slow crystallization, especially when a globally decreasing trend right from the beginning of the time measurement is considered. Smaller differences also reflect a lower amorphous fraction in the system, which is related to a lower DMSO concentration (5 vs. 10%), which can also be seen in the results from temperature measurements and the size of the hysteresis between cooling and heating (Table 1).
These results of isothermal time measurements imply that heating rate applied during thawing of frozen cells may be critical for their viability, what matches the actual results for such experiments performed on biological cells. Although it appears that short-term storage at 180 K is possible without eutectic crystallization occurring (temperature of 180 K is roughly the temperature of typical freezers used for such purposes), these results suggest that it is safer to “complete” the solidification process and store the cells at temperature lower than glass transition temperature of water–DMSO mixtures what is about 150 K.
Conclusions
Presence of DMSO in the liposome containing medium induced qualitative change in the phase behavior of water inside the unilamellar liposomes – its crystallization was postponed down to temperatures lower than 235 K. This result was interpreted as indication of lipid bilayer being protected against ice crystallization front by presence of the ice-free phase consisting of freeze-concentrated DMSO. This phase surrounds the cells during freezing what justifies the hypothesis that one aspect of DMSO cryoprotective action stems from the ability of the ice-free phase to reduce the probability of the contact between extracellular ice crystals and plasma membrane, preventing the nucleation of intracellular ice and/or direct mechanical damage to the membrane during freezing.
During the freezing, vitrification of freeze-concentrated phase was observed, but its possible crystallization upon thawing was demonstrated. The found correlation between the critical temperature range for cell viability loss during slow thawing and temperatures of crystallization of freeze-concentrated phase of DMSO is of critical importance for successful cryopreservation of biological cells. Based on this correlation and the afore-mentioned results we may hypothesize that another aspect of cryoprotection brought by DMSO is that the freeze-concentrated phase remains in amorphous state during thawing as well. Since DMSO is also present in cytoplasm, a possible threat brought by eutectic crystallization is increased.
Considering these two proposed mechanisms of cryoprotection induced by DMSO, the concentration dependence of its cryoprotective action may be ascribed to two factors: (1) since the amount of ice-free phase in the freezing mixture is directly proportional to the DMSO concentration, the probability of contact between the plasma membrane and extracellular ice crystals is reduced more when a higher concentration of DMSO is used, (2) extent of cell dehydration during slow (equilibrium) freezing should be decreased by presence of DMSO in cytoplasm with assumption that concentration of DMSO is roughly the same on intracellular and extracellular side of plasma membrane during the freezing process. Again, higher concentration of DMSO means higher absolute number of DMSO molecules in a cell what leads to bigger cell volume and thus decreased stress from cell dehydration.
For the design of cryopreservation protocols with application of novel experimental cryoprotectants, this study implies the choice of such additives that can easily form an amorphous phase in freezing cell medium. Additives, that are characterized by strong intermolecular interactions with water, what is manifested by increased freezing point depression and higher amount of amorphous phase in frozen state, can be good candidates for novel cryoprotectants.
Conflicts of interest
There are no conflicts to declare.
Acknowledgements
This study was supported by the Slovak Grant Agency VEGA (project no. 2/0134/21), Slovak Research and Development Agency (projects no. APVV-16-0369 and APVV-19-0019), NAWA International Academic Partnership Programme and Foundation for Cell Transplantation. The authors would also like to thank prof. RNDr. Melánia Babincová, DrSc. and Mgr. Zuzana Garaiová, PhD for help with liposome preparation and characterization.
Notes and references
- D. E. Pegg, Principles of cryopreservation, Preserv. Hum. Oocytes From Cryobiol. Sci. to Clin. Appl., 2009, 368, pp. 12–24 Search PubMed.
- T. H. Jang, S. C. Park, J. H. Yang, J. Y. Kim, J. H. Seok, U. S. Park, C. W. Choi, S. R. Lee and J. Han, Cryopreservation and its clinical applications | Elsevier Enhanced Reader, Integr. Med. Res., 2017, 6, 12–18 CrossRef PubMed.
- W. F. Rall, P. Mazur and H. Souzu, Physical-Chemical Basis of the Protection of Slowly Frozen Human Erythrocytes by Glycerol, Biophys. J., 1978, 23, 101–120 CrossRef CAS PubMed.
- P. Mazur, Freezing of living cells: mechanisms and implications, Am. J. Physiol., 1984, 247, C125–C142 CrossRef CAS PubMed.
- J. E. Lovelock, Het mechanism of the protective action of glycerol against haemolysis by freezing and thawing, Biochim. Biophys. Acta, 1953, 11, 28–36 CrossRef CAS.
- P. Mazur, in Life in the Frozen State, 2004, pp. 3–65 Search PubMed.
- J. E. Lovelock and M. W. H. Bishop, Prevention of Freezing Damage to Living Cells by Dimethyl Sulphoxide, Nature, 1959, 183, 1394–1395 CrossRef CAS PubMed.
- L. A. Marquez-Curtis, A. Janowska-Wieczorek, L. E. McGann and J. A. W. Elliott, Mesenchymal stromal cells derived from various tissues: biological, clinical and cryopreservation aspects, Cryobiology, 2015, 71, 181–197 CrossRef CAS PubMed.
- J. Lakota and P. Fuchsberger, Autologous stem cell transplantation with stem cells preserved in the presence of 4.5 and 2.2% DMSO, Bone Marrow Transplant., 1996, 18, 262–263 CAS.
- G. Chen, A. Yue, Z. Ruan, Y. Yin, R. Wang, Y. Ren and L. Zhu, Comparison of the effects of different cryoprotectants on stem cells from umbilical cord blood, Stem Cells Int., 2016, 2016, 1396783 Search PubMed.
- Z. Yuan, S. D. S. Lourenco, E. K. Sage, K. K. Kolluri, M. W. Lowdell and S. M. Janes, Cryopreservation of human mesenchymal stromal cells expressing TRAIL for human anti-cancer therapy, Cytotherapy, 2016, 18, 860–869 CrossRef CAS PubMed.
- K. Hornberger, G. Yu, D. McKenna and A. Hubel, Cryopreservation of Hematopoietic Stem Cells: Emerging Assays, Cryoprotectant Agents, and Technology to Improve Outcomes, Transfus. Med. Hemother., 2019, 46, 188–196 CrossRef PubMed.
- Z. Shu, S. Heimfeld and D. Gao, Hematopoietic SCT with cryopreserved grafts: adverse reactions after transplantation and cryoprotectant removal before infusion, Bone Marrow Transplant., 2014, 49, 469–476 CrossRef CAS PubMed.
- R. Spindler, W. F. Wolkers and B. Glasmacher, Dimethyl sulfoxide and ethylene glycol promote membrane phase change during cryopreservation, Cryo-Letters, 2011, 32, 148–157 CAS.
- P. Mazur, The role of intracellular freezing in the death of cells cooled at supraoptimal rates, Cryobiology, 1977, 14, 251–272 CrossRef CAS PubMed.
- P. Mazur, in Cellular Injury and Resistance in Freezing Organisms: proceedings, 1967, 2, pp. 171–189 Search PubMed.
- P. Mazur, S. P. Leibo and E. H. Y. Chu, A two-factor hypothesis of freezing injury, Exp. Cell Res., 1972, 71, 345–355 CrossRef CAS PubMed.
- K. Čechová, I. Maťko, J. Rusnák, H. Švajdlenková, I. Klbik, J. Lakota and O. Šauša, Microstructural free volume and dynamics of cryoprotective DMSO–water mixtures at low DMSO concentration, RSC Adv., 2019, 9, 34299–34310 RSC.
- J. Meneghel, P. Kilbride, J. G. Morris and F. Fonseca, Physical events occurring during the cryopreservation of immortalized human T cells, PLoS One, 2019, 14, e0217304 CrossRef CAS PubMed.
- J. Liu, E. J. Woods, Y. Agca, E. S. Critser and J. K. Critser, Cryobiology of rat embryos II: a theoretical model for the development of interrupted slow freezing procedures, Biol. Reprod., 2000, 63, 1303–1312 CrossRef CAS PubMed.
- I. Maťko, O. Šauša, E. Macová and D. Berek, Combined study of confined water in controlled pore glasses by differential scanning calorimetry and positron annihilation lifetime spectroscopy, J. Therm. Anal. Calorim., 2015, 121(1), 163–168 CrossRef.
- I. Maťko, O. Šauša, K. Čechová and K. Jesenák, Study of water in Ca-montmorillonite by thermal analysis and positron annihilation lifetime spectroscopy, J. Therm. Anal. Calorim., 2018, 133, 247–254 CrossRef.
- V. Majerník, J. Krištiak, O. Šauša and M. Iskrová-Miklošovičová, “In Situ” Observation of Crystallization in Propylene Carbonate, Salol and M-Toluidine, Mater. Sci. Forum, 2012, 733, 84–87 Search PubMed.
- B. Zgardzińska and K. Standzikowski, A New Approach to the Presentation of the Positron Annihilation Lifetime Spectroscopy Results, n-Alkanes, Acta Phys. Pol., A, 2017, 132, 1496–1501 CrossRef.
- R. G. LeBel and D. A. I. Goring, Density, Viscosity, Refractive Index, and Hygroscopicity of Mixtures of Water and Dimethyl Sulfoxide, J. Chem. Eng. Data, 1962, 7, 100–101 CrossRef CAS.
- J. Kansy, LT Polymers, 2009, available from, http://prac.us.edu.pl/%7Ekansy/index.php?id=ltpolym Search PubMed.
- J. Kansy, Microcomputer program for analysis of positron annihilation lifetime spectra, Nucl. Instrum. Methods Phys. Res. A: Accel. Spectrom. Detect. Assoc. Equip., 1996, 374, 235–244 CrossRef CAS.
- I. Tosun, The Thermodynamics of Phase and Reaction Equilibria, 2012, pp. 509–512 Search PubMed.
- N. S. Osborne, Heat of fusion of ice. A revision, J. Res. Natl. Bur. Stand., 1939, 23, 643 CrossRef CAS.
- M. Caffrey and J. Hogan, LIPIDAT: a database of lipid phase transition temperatures and enthalpy changes. DMPC data subset analysis, Chem. Phys. Lipids, 1992, 61, 1–109 CrossRef CAS PubMed.
- A. E. Carte, The Freezing of Water Droplets, Proc. Phys. Soc., London, Sect. B, 1956, 69, 1028–1037 CrossRef.
- G. Bryant, DSC Measurement of Cell Suspensions during Successive Freezing Runs: Implications for the Mechanisms of Intracellular Ice Formation, Cryobiology, 1995, 32, 114–128 CrossRef CAS PubMed.
- Q. Wang, L. Zhao, C. Li and Z. Cao, The decisive role of free water in determining homogenous ice nucleation behavior of aqueous solutions, Sci. Rep., 2016, 6, 26831 CrossRef CAS PubMed.
- K. Miyata and H. Kanno, Supercooling behavior of aqueous solutions of alcohols and saccharides, J. Mol. Liq., 2005, 119, 189–193 CrossRef CAS.
- C. Körber, S. Englich and G. Rau, Intracellular ice formation: cryomicroscopical observation and calorimetric measurement, J. Microsc., 1991, 161, 313–325 CrossRef PubMed.
- P. Mazur, W. F. Rall and N. Rigopoulos, Relative contributions of the fraction of unfrozen water and of salt concentration to the survival of slowly frozen human erythrocytes, Biophys. J., 1981, 36, 653–675 CrossRef CAS PubMed.
- L. Weng, W. Li, J. Zuo and C. Chen, Osmolality and Unfrozen Water Content of Aqueous Solution of Dimethyl Sulfoxide, J. Chem. Eng. Data, 2011, 56, 3175–3182 CrossRef CAS.
- D. E. Pegg and M. P. Diaper, The ‘Unfrozen Fraction’ Hypothesis of Freezing Injury to Human Erythrocytes: A Critical Examination of the Evidence, Cryobiology, 1989, 26, 30–43 CrossRef CAS PubMed.
- D. H. Rasmussen and A. P. Mackenzie, Phase Diagram for the System Water–Dimethylsulphoxide, Nature, 1968, 220, 1315–1317 CrossRef CAS PubMed.
- B. Kirchner and M. Reiher, The Secret of Dimethyl Sulfoxide–Water Mixtures. A Quantum Chemical Study of 1DMSO– n Water Clusters, J. Am. Chem. Soc., 2002, 124, 6206–6215 CrossRef CAS PubMed.
- M. Toner, E. G. Cravalho, M. Karel and D. R. Armant, Cryomicroscopic analysis of intracellular ice formation during freezing of mouse oocytes without cryoadditives, Cryobiology, 1991, 28, 55–71 CrossRef CAS PubMed.
- W. F. Rall, P. Mazur and J. J. McGrath, Depression of the ice-nucleation temperature of rapidly cooled mouse embryos by glycerol and dimethyl sulfoxide, Biophys. J., 1983, 41, 1–12 CrossRef CAS PubMed.
- M. Shabana and J. J. McGrath, Cryomicroscope investigation and thermodynamic modeling of the freezing of unfertilized hamster ova, Cryobiology, 1988, 25, 338–354 CrossRef CAS PubMed.
- M. Toner, E. G. Cravalho and M. Karel, Thermodynamics and kinetics of intracellular ice formation during freezing of biological cells, J. Appl. Phys., 1990, 67, 1582–1593 CrossRef.
- J. A. Mugnano, T. Wang, J. R. Layne, A. L. DeVries and R. E. Lee, Antifreeze glycoproteins promote intracellular freezing of rat cardiomyocytes at high subzero temperatures, Am. J. Physiol.: Regul., Integr. Comp. Physiol., 1995, 269, R474–R479 CrossRef CAS PubMed.
- M. Köseoğlu, A. Eroğlu, M. Toner and K. C. Sadler, Starfish Oocytes Form Intracellular Ice at Unusually High Temperatures, Cryobiology, 2001, 43, 248–259 CrossRef PubMed.
- P. Mazur, The Role of Cell Membranes in the Freezing of Yeast and Other Single Cells, Ann. N. Y. Acad. Sci., 2006, 125, 658–676 CrossRef PubMed.
- O. Šauša, M. Iskrová, B. Sláviková, V. Majerník and J. Krištiak, Positron Annihilation in Undercooled Water and Ice, Mater. Sci. Forum, 2010, 666, 115–118 Search PubMed.
- M. Eldrup, O. Mogensen and G. Trumpy, Positron Lifetimes in Pure and Doped Ice and in Water, J. Chem. Phys., 1972, 57, 495–504 CrossRef CAS.
- O. E. Mogensen and M. Eldrup, Vacancies in Pure Ice Studied by Positron Annihilation Techniques, J. Glaciol., 1978, 21, 85–99 CrossRef CAS.
- S. S. N. Murthy, Some Insight into the Physical Basis of the Cryoprotective Action of Dimethyl Sulfoxide and Ethylene Glycol, Cryobiology, 1997, 36, 84–96 CrossRef PubMed.
- A. D. Fortes, J. Ponsonby, O. Kirichek and V. García-Sakai, On the crystal structures and phase transitions of hydrates in the binary dimethyl sulfoxide-water system, Acta Crystallogr., Sect. B: Struct. Sci., Cryst. Eng. Mater., 2020, 76, 733–748 CrossRef CAS PubMed.
- W. F. Rall, D. S. Reid and C. Polge, Analysis of slow-warming injury of mouse embryos by cryomicroscopical and physiochemical methods, Cryobiology, 1984, 21, 106–121 CrossRef CAS PubMed.
- S. Mori, J. Choi, R. V. Devireddy and J. C. Bischof, Calorimetric measurement of water transport and intracellular ice formation during freezing in cell suspensions, Cryobiology, 2012, 65, 242–255 CrossRef CAS PubMed.
- B. Sydykov, H. Oldenhof, H. Sieme and W. F. Wolkers, Storage stability of liposomes stored at elevated subzero temperatures in DMSO/sucrose mixtures, PLoS One, 2018, 13, e0199867 CrossRef PubMed.
|
This journal is © The Royal Society of Chemistry 2022 |