DOI:
10.1039/D1RA08765G
(Review Article)
RSC Adv., 2022,
12, 7789-7820
Secondary metabolites from marine sponges of the genus Agelas: a comprehensive update insight on structural diversity and bioactivity
Received
1st December 2021
, Accepted 3rd March 2022
First published on 9th March 2022
Abstract
As one of the most common marine sponges in tropical and subtropical oceans, the sponges of the genus Agelas, have emerged as unique and yet under-investigated pools for discovery of natural products with fabulous molecular diversity and myriad interesting biological activities. The present review highlights the chemical structure and biological activity of 355 compounds that have been isolated and characterized from the members of Agelas sponges, over the period of about five decades (from 1971 to November 2021). For a better understanding, these numerous compounds are firstly classified and presented according to their carbon skeleton as well as their biosynthetic origins. Relevant summaries focusing on the source organism and the associated bioactivity of these compounds belonging to different chemical classes are also provided. This review highlights sponges of the genus Agelas as exciting source for discovery of intriguing natural compounds.
1. Introduction
Natural products have historically served as potential sources of lead compounds for drug development.1 Under physically variable and competitive environments, marine sponges have necessitated the evolution of thorough metabolic capability to produce diverse physiologically active secondary metabolites. About 9500 new compounds have been isolated from marine sponges during the period of 1950 to 2019, most of them exhibiting a wide range of biological activities.2,3 As one of the most common sponges in tropical and subtropical areas around the world, marine sponges of the genus Agelas (class Demospongiae, order Agelasida, family Agelasidae), include to date 36 valid species, and knowledge of its species continues to expand.4 The reasons for us discussing this genus of sponges are the chemical richness and interesting biological activity of their secondary metabolites as well as the wide geographical distribution in the global ocean, especially in the Okinawa Sea, the Caribbean Sea and the South China Sea. Many of these metabolites are of mixed biogenetic origin, as illustrated by alkaloids (especially pyrrole alkaloids and terpenoid alkaloids), glycosphingolipids, sterols, carotenoids, and so on. Although Zhang et al. have reviewed the 291 secondary metabolites isolated from Agelas sponges according to their biological sources,5 few reports have analysed the structure and chemical classification of the diverse Agelas-derived natural products. In order to better illustrate the intriguing chemistry and biology of these natural products and rationally exploit the resources of Agelas sponges, the 355 compounds isolated from Agelas sponges, covering the period from 1971 to November 2021, are systematically categorized and grouped into several families according to their carbon skeleton as well as biosynthetic origins, and their sources and a wide range of bioactivities are also summarized.
2. Pyrrole alkaloids
As a fascinating group of a large variety of secondary metabolites produced by Agelas sponges, pyrrole alkaloids are of historic importance in marine natural products chemistry due to their high chemodiversity and interesting bioactivities. Structurally, most of them share a bromo- or debromopyrrole-2-carboxamide core with diverse linear side chains (linear pyrrole alkaloids) and fused cyclic systems (fused cyclic pyrrole alkaloids). Furthermore, the monomers can dimerize to give a large variety of dimers (dimeric pyrrole alkaloids). It is interesting to note that, despite their structural diversity, the biosynthetic origin of these pyrrole alkaloids can be traced back to a small number of possible precursors. According to the stunning logic of nature, only a few fundamental amino acids, including proline and lysine, are necessary to construct the simple pyrrole alkaloids (e.g., oroidin), which are building blocks to form complex pyrrole alkaloids with various molecular architectures.6
2.1. Linear pyrrole alkaloids
Most of the linear pyrrole alkaloids are pyrrole-imidazole alkaloids (PIAs) constructed by the bromo- or debromopyrrole-2-carboxamide core connected with an aminoimidazole group through an aliphatic segment. These chemically interesting alkaloids, produced exclusively by marine sponges, have been regarded as useful chemotaxonomic markers for axinellid sponges that were once allied with the Agelasida, such as the sponges of the genus Agelas, Axinella, Acanthella, Hymeniacidon, Phakellia and Pseudaxinyssa.7 Additionally, the linear pyrrole alkaloids lacking an aminoimidazole moiety in the molecules have also been reported from Agelas sponges.
2.1.1. Pyrrole-imidazole alkaloids (PIAs). From Fig. 1—the first member of the PIAs to be isolated was oroidin (1), initially from A. oroides collected in the bay of Naples in 1971.8 However, the initial structure of oroidin was not exactly right; afterwards the structure was reassigned based on a chemical synthesis.9 Subsequently, three closely related analogues, including the nonbrominated clathrodin (2) from the Caribbean A. clathrodes collected from Desecheo Island,10 the monobrominated hymenidin (3) and N-methylated derivative sventrin (4) from Bahamas A. sventres11 have been discovered. The simplest PIAs oroidin (1), clathrodin (2) and hymenidin (3), the structures of which differ only in bromine content, often served as the key monomeric building blocks to biosynthesize a large variety of chemically complex PIAs with amazing molecular diversity. Several lines of investigation aimed at understanding the origin and the reactivity of the precursors 1–3 and possible pathways for their conversion to higher-order PIAs have been undertaken.6 As a consequence, proline was strongly suggested as the precursor of the pyrrolyl moiety in 1–3, while the 2-aminoimidazole part of 1–3 was thought to originate from lysine (Scheme 1). These biosynthesis speculations on PIAs have been demonstrated by feeding experiments with radioisotopes, precursor-guided mining and detection of marine sponge metabolomes, and de novo synthesis using cell-free enzyme preparations.12–15 Biological evaluation revealed that compound 1 had a broad spectrum of bioactivities, including antibacterial,16 antifouling,17 antipredatory defense against reef fish,18–20 antimalarial,21 acetylcholinesterase inhibitory and radical scavenging,22 inhibitory action towards voltage-gated potassium channels,23 and inhibiting biofilm formation of pathogenic and marine environment derived bacteria.24–27 Compound 2 exhibited cytotoxicity against Chinese hamster ovary (CHO)-K1 cells (ED50, 1.33 μg mL−1), blocking activity against cholinergic receptors on the isolated frog skeletal muscle, and modulatory effect on voltage-gated sodium and potassium channels,10,23,28 while compound 3 was proved to be an inhibitor of the voltage-gated potassium channels,23 and compound 4 was feeding deterrent against omnivorous reef fish.11
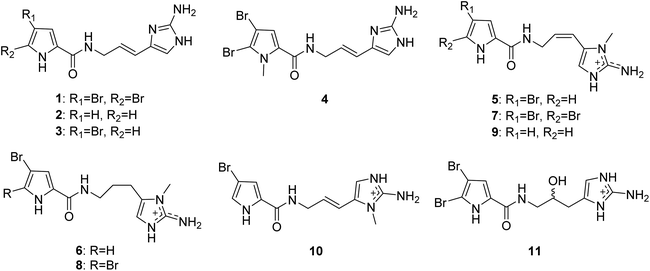 |
| Fig. 1 Chemical structures of 1–11. | |
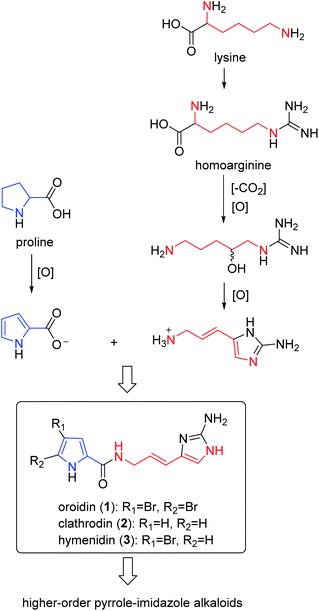 |
| Scheme 1 Origin of the building blocks oroidin (1), clathrodin (2) and hymenidin (3) from amino acid precursors. | |
Keramadine (5),29 9,10-dihydrokeramadine (6),30 2-bromokeramadine (7) and 2-bromo-9,10-dihydrokeramadine (8) identified from Okinawan sponge Agelas sp.,31 and debromokeramadine (9) from the Solomon Islands A. cf. mauritiana32 are oroidin congeners with an N-methylated aminoimidazole moiety. It is worth noting that in contrast to E configuration of the vinylic double bond in oroidin and many other analogues, Z configuration is characteristic of compounds 5–9.32 Biological studies have identified that compound 5 was an effective antagonist of serotonergic receptors, and had antibacterial activities against Bacillus subtilis, Staphylococcus aureus and Micrococcus luteus (MIC, 40, 35 and 4.0 μg mL−1, respectively).29,31,33 In addition, a recent report on Okinawan sponges Agelas sp. described two closely related congeners, 9E-keramadine (10) and 9-hydroxydihydrooroidin (11).34
From Fig. 2—four oroidin derivatives with an aminoimidazolone moiety, dispacamides A–D (12–15), were reported from Caribbean species A. dispar, A. clathrodes, A. longissima and A. conifer, collected from Little San Salvador Island, by Fattorusso's group. All the four compounds were evaluated for the antihistaminic activity on the guinea pig ileum, with compounds 12 and 13 being more potent than 14 and 15, indicating the insertion of a hydroxylic group in the central chain causes a marked reduction of the antihistaminic activity.35,36 Further biological researches suggested that compound 12 served as the primary chemical defense of Agelas sponges,37 while compound 13 emerged as an antimalarial lead compound with significant activity and low toxicity towards mammalian cells.38 Two nonbrominated analogues, debromodispacamides B (16) and D (17), were purified from the Solomon Islands collection of A. mauritiana, and a biomimetic one-step reaction gave the chiral form of the natural product stereoselectively.39 Recently, the study of A. oroides collected near the Israeli Mediterranean coastline and Okinawan sponges Agelas sp. yielded E-dispacamide (18)27 and 9-hydroxydihydrodispacamide (19),34 respectively.
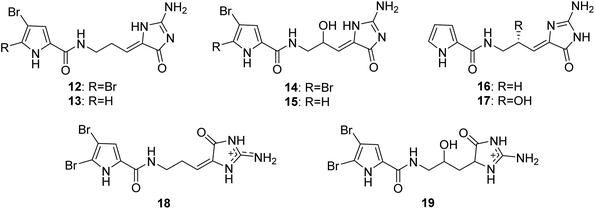 |
| Fig. 2 Chemical structures of 12–19. | |
From Fig. 3—further examples of Agelas-derived PIAs were oroidin derivatives with a hydantoin unit (20–28). Midpacamide (20) and 5-debromomidpacamide (21) were obtained from the Enewetak Atoll A. mauritiana,40 while an Okinawan specimen of A. nakamurai was the source of mukanadins A (dispacamide D, 15) and B (22),41 and chemical investigations of Mediterranean A. oroides, collected off the Tel Aviv coast, and the Okinawan Agelas sp. afforded mukanadins D (23)27 and F (24),42 respectively. It should be noted that unlike the usually encountered racemic or scalemic mixtures of PIAs, compound 24 was isolated as a single enantiomer with the absolute configuration established using Mosher's esters.42 The Agelas sponges from the Xisha Islands in the South China Sea were demonstrated to contain 9-oxethyl-mukanadin F (25) from Agelas sp.,43 the nonbrominated nakamurine D (26) and a known congener 27 from A. nakamurai44,45 and nemoechine H (28) from A. nemoechinata.46 Among which, compound 28 possessed cytotoxic activity against human leukemia cell K562 and human liver cell L-02 (IC50, 6.1 and 12.3 μM, respectively).46
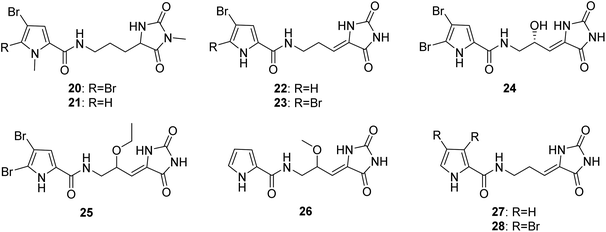 |
| Fig. 3 Chemical structures of 20–28. | |
From Fig. 4—the sponges of the genus Agelas were also the source of a quite rare family of PIAs with a taurine moiety. The first member of this family is the Fijian species A. mauritiana derived mauritamide A (29), which is an oroidin derivative having a taurine methyl ester group.47 Two congeners, mauritamides B (30) and C (31) were detected in the Indonesian A. linnaei.48 Investigation of the chemistry of Mediterranean A. oroides afforded taurodispacamide A (32), which displayed a good antihistaminic activity tested on the isolated guinea pig ileum and antimicrobial activities against Bacillus subtilis, Staphylococcus aureus, Escherichia coli, Pseudomonas aeruginosa and Candida albicans with IC50 values ranging from 0.21 to 1.4 μM.16,49 A series of taurine containing PIAs, tauroacidins A (33) and C–E (34–36),31,50 nagelamides M (37), N (38),51 U (39) and V (40)52 were isolated from Okinawan Agelas sp., by Kobayashi's group. Structurally, compound 37 possesses a unique 2-amino-octahydropyrrolo[2,3-d]imidazole ring,51 while compounds 39 and 40 are the first example for a pyrrole alkaloid with a γ-lactam ring.52 Biosynthetically, compounds 37–40 could be derived from the same precursor (32) through oxidation and cyclization.51,52 In the bioassays, compound 33 not only exhibited tyrosine kinase inhibitory effect (IC50, 20 μg mL−1) but also had antibacterial activity against M. luteus (MIC, 8.0 μg mL−1),31,53 while compounds 37 and 38 had antimicrobial activity against Aspergillus niger (MIC, 33.3 μg mL−1, each),51 and compound 39 was active against C. albicans (IC50, 4 μg mL−1).52
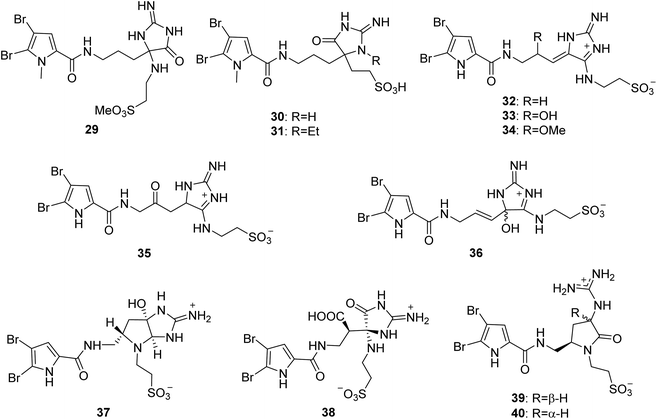 |
| Fig. 4 Chemical structures of 29–40. | |
From Fig. 5—three unique PIAs 41–43 were identified from the prolific Okinawan Agelas sponges, which were proved to be a rich source of structurally intriguing compounds. Compound 41 (nagelamide W) is the first monomeric pyrrole alkaloid containing two aminoimidazole units in the molecule, and exhibited antimicrobial activity against C. albicans (IC50, 4 μg mL−1).52 Compound 42 (mukanadin G) with a tricyclic skeleton consisting of a fused tetrahydrobenzaminoimidazole and 2,5-dioxopyrrolidine moieties, was found to have antifungal activity against C. albicans and Cryptococcus neoformans (IC50, 16 and 8.0 μg mL−1, respectively).31 Compound 43 (agelamadin F) is the first example for a pyrrole alkaloid with the coexistence of aminoimidazole and pyridinium moieties.50 In a further study of Caribbean Agelas specimens collected from Little San Salvador Island, Fattorusso's group discovered two pairs of diastereomeric bromopyrrole alkaloids sharing an uncommon N-methylimidazolinium moiety, clathramides A (44) and B (45) from A. clathrodes, and clathramides C (46) and D (47) from A. dispar. All the four clathramides (44–47) displayed moderate antifungal activity against A. niger (100 μg of the mixture caused a zone of inhibition of 7–8 mm).33,54 The Okinawan A. nakamurai was the source of the unusual PIAs sharing a tetrabydrofuro[2,3-d]imidazolidin-2-one moiety, slagenins A–C (48–50), with 49 and 50 showing cytotoxic activity against routine leukemia L1210 cells (IC50, 7.5 and 7.0 μg mL−1, respectively).55 Recently, slagenin D (51), presenting opposite absolute configuration of stereogenic centers relative to those of slagenin A (48), was discovered from Mediterranean A. oroides, collected in Tel Aviv.27 Examination of Xisha Islands A. aff. nemoechinata led to the isolation of nemoechine A (52) bearing an uncommon cyclopentane-fused imidazole ring system.56 Ageladine A (53) containing 2-aminoimidazolopyridine and agelanin A (54) with 2-aminoimidazolo-tetrahydropyridinol, were characterized from Kuchinoerabu-jima Island A. nakamurai57 and Thousand Islands A. linnaei,48 respectively. In addition to the important pharmacological activity as antiangiogenic compound and metallo-protease inhibitor, compound 53 was a reliable and stable fluorescent pH sensor, implying utility as a membrane permeable dye.57,58
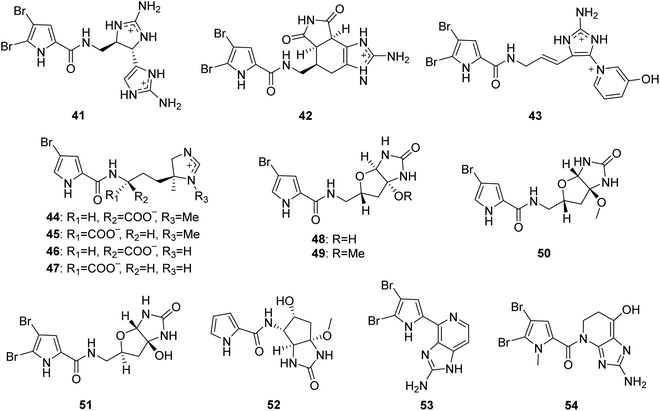 |
| Fig. 5 Chemical structures of 41–54. | |
2.1.2. Pyrrole alkaloids without an imidazole moiety. From Fig. 6—the first investigation of the constituents of A. oroides collected in the bay of Naples identified the pyrrole alkaloids without an imidazole moiety (55–58),8 which were common metabolites encountered in Agelas species and had many bioactivities. Compound 55, 4,5-dibromopyrrole-2-carboxylic acid, displayed immunosuppressive effect and feeding deterrent activity towards reef fishes,18,59 while compound 56, 4,5-dibromopyrrole-2-methylcarboxylate, could mildly inhibit protein tyrosine phosphatase 1B (PTP1B),60 and compound 58, 4,5-dibromopyrrole-2-carboxamide, not only had the ability to promote larval metamorphosis of the ascidian Ciona savignyi17 but also inhibited Pseudomonas aeruginosa PAO1 biofilms.27 A study of Great Barrier Reef collection of A. oroides resulted in the isolation of pyrrole-2-carboxamide (59) and N-formyl-pyrrole-2-carboxamide (60).61 2,3-Dibromopyrrole (61) and 2,3-dibromo-5-methoxymethylpyrrole (62) were discovered from Agelas sp. without collection location data.62 The Papua New Guinean sponge A. nakamurai was the source of 5-bromopyrrole-2-carboamide (63) and 5-bromopyrrole-2-(-N-methoxymethyl)carboxamide (64) with 63 being active against some Gram-positive bacteria and fungi,63 while 4-bromopyrrole-2-carboxylic acid (65) and 4-bromopyrrole-2-carboxamide (66) have been sourced from Menjangan Island specimen of A. nakamurai.48 The first report of a chemical study on Caribbean A. cerebrum collected from Cuba, concluded 5-bromopyrrole-2-carboxylic acid (67) and 3,4-bromopyrrole-2-carboxylic acid (68).64 The species A. mauritiana from Enewetak Atoll could produce methyl N-methyl-4,5-dibromopyrrole-2-carboxylate (69).40
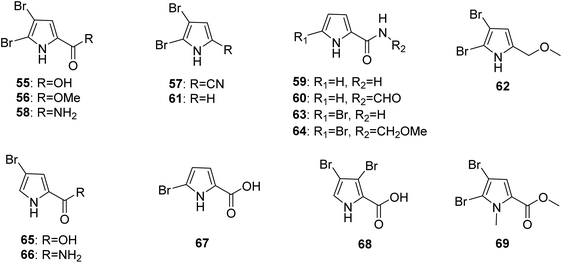 |
| Fig. 6 Chemical structures of 55–69. | |
From Fig. 7—agelongine (70) and N-methylagelongine (71), obtained from San Salvador Island specimens of A. longissima and A. citrina, respectively, contain an uncommon pyridinium ring in place of the imidazole nucleus as usually found in PIAs.65,66 Manzacidin C (72), isolated from the Indonesian Agelas sp. collected from Likpan, is the first pyrrole alkaloid with a tetrahydropyrimidine unit from Agelas sponges.67 In the bioactivity study, compound 70 displayed antiserotonergic activity tested in vitro on rat stomach fundus strip.66 The chemistry study on Caribbean A. dispar collected near the Venezuelan island La Blanquilla yielded dispyrin (73), which is featured with a rare bromopyrrole tyramine motif and represent a notable variation from the oroidin family.68 With no antibacterial or anticancer activity, compound 73 was a potent ligand and antagonist of several therapeutically relevant G protein-coupled receptors, the α1D and α2A adrenergic receptors and the H2 and H3 histamine receptors.69,70 The Indonesian specimen A. linnaei collected from Thousand Islands could produce a number of linear pyrrole alkaloids without an imidazole moiety, including agelanesins A–D (74–77), N-methyl-4,5-dibromopyrrole-2-carboxylic acid (78), butanoic acid derivative of 4,5-dibromo-1-methyl-1H-pyrrole-2-carboxamide (79), agelanin B (80), and mauritamide D (81). Compounds 74–77 are the first naturally occurring compounds featuring a halogenated tyramine unit linked to a brominated pyrrole unit. Furthermore, replacement of the bromine functionality by an iodine residue in the tyramine moiety as found in 75 and 77 makes these compounds even more attractive. Among halogenated natural products, iodo-alkaloids are known as very rare compounds due to the fact that most reported halogenases catalyze reactions with chlorine and bromine donors.71 Compounds 74–77 were found to have mild cytotoxic activity against L5178Y mouse lymphoma cells (IC50, 9.25–16.76 μM).48 The sponges A. wiedenmayeri collected off the coast of Florida Keys and Okinawan Agelas sp. contained 4-bromopyrrole-2-carboxyhomoarginine (82)72 and mukanadin E (83),42 respectively. Compound 82 is of interest because it does not correspond to the proposed biosynthesis of the oroidin-like alkaloids and may be alternatively a biosynthetic precursor of oroidin/hymenidin related alkaloids.72 Pyrrolosine (84), comprised by two symmetric 4,5-dibromopyrrole-2-carboxamide moieties was discovered from Mediterranean A. oroides, collected in Tel Aviv.27
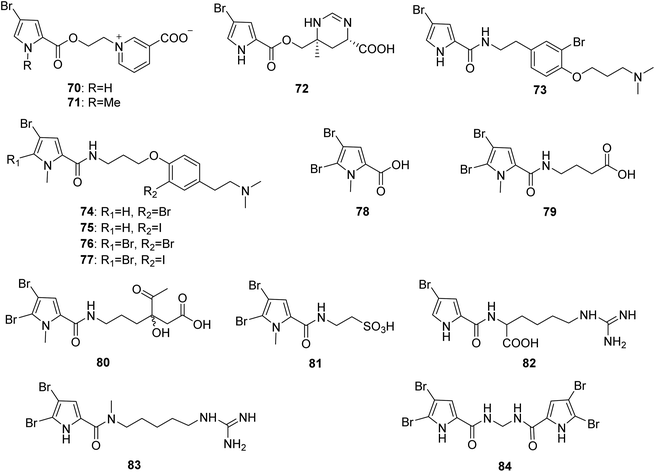 |
| Fig. 7 Chemical structures of 70–84. | |
From Fig. 8—Agelas sponges from the Xisha Islands were an excellent source of structurally novel pyrrole alkaloids without an imidazole moiety, including 4-bromo-N-(butoxymethyl)-1H-pyrrole-2-carboxamide (85) from A. mauritiana,73 3-oxethyl-4-[1-(4,5-dibromopyrrole-2-yl)-formamido]-butanoic acid methyl ester (86) and 2-oxethyl-3-[1-(4,5-dibromopyrrole-2-yl)-formamido]-methyl propionate (87) from Agelas sp.,43 nakamurines A–C (88–90) and E (91) along with seven known congeners 92–98 from A. nakamurai,44,45 nemoechine C (99) and the previously reported 100 and 101 from A. aff. nemoechinata.56 Besides, exploration for specialized metabolites of Okinawan Agelas sp. resulted in isolation of two analogues, agesasines A (102) and B (103).34
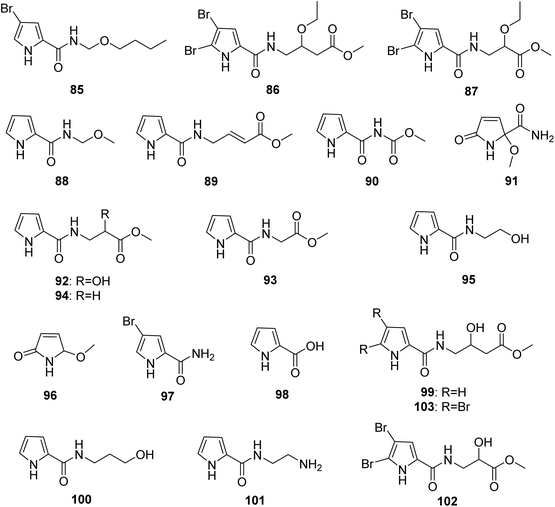 |
| Fig. 8 Chemical structures of 85–103. | |
Among pyrrole alkaloids without an aminoimidazole moiety, the simple pyrroles (such as compounds 55–69 and 96–98) may serve as precursors or intermediates involved in biosynthesis of this family of alkaloids, whereas other compounds containing an ester group at the end of the side chain (such as the Xisha Islands derived compounds 86, 87, 89, 90, 92–94 and 99, and the Okinawa derived compounds 102 and 103) might be artifacts during the extraction and isolation process.34,43
2.2. Fused cyclic pyrrole alkaloids
By analysing the structures of fused cyclic pyrrole alkaloids, we can easily find that the position of fusion usually occurred between C-1 and C-2, with the fused ring being five- or six-membered. Additionally, these alkaloids often share a carbonyl moiety in α-position to the bridgehead atom. These common structural features are attributed to the similar biosynthetic origin of these metabolites, which may be formed by oroidin (1) and its analogues through cyclization and oxidation processes.74 With the amazing biosynthesis capacity, the sponges could produce an array of fused cyclic pyrrole alkaloids with diverse structure frameworks. According to different oroidin atoms involved in the linkage formation, these compounds can be classified into four groups: agelaspongin family (N1/C12 + N7/C12), phakellin family (N1/C12 + N7/C11 or C4/C12 + N7/C11), agelastatin family (N1/C9 + C8/C12) and longamide family (N1/C9) (Scheme 2).
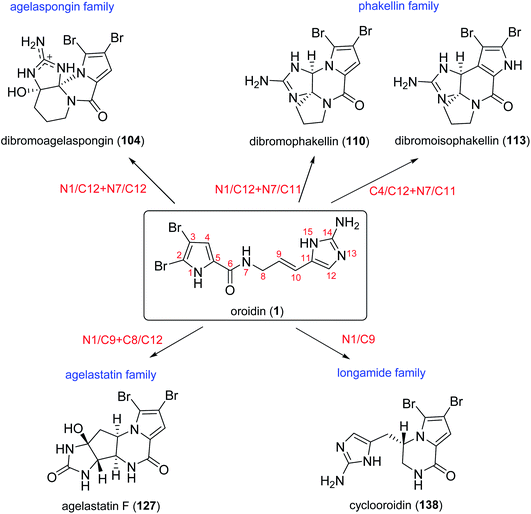 |
| Scheme 2 The four groups of fused cyclic pyrrole alkaloids formed by different cyclization modes of oroidin (1). | |
2.2.1. Agelaspongin family. From Fig. 9—agelaspongins (104–109) are a family of tetracyclic PIAs usually found in Agelas sponges. Dibromoagelaspongin hydrochloride (104), the structure of which has been elucidated by X-ray analysis, was characterized from the Tanzania sea Agelas sp.,75 and was later found to have the ability to stimulate growth of seedling roots of wheat Triticum aestivum L.76 The Caribbean specimen of A. dispar, collected near the Venezuelan island La Blanquilla, could metabolize dibromoagelaspongin methyl ether (105).68 LC-MS based metabolomics study of A. oroides collected from West Mediterranean Sea revealed that this sample contained a racemic monobromo analogue, monobromoagelaspongin (106),77 while a further investigation of the Tel Aviv (East Mediterranean Sea) A. oroides yielded the optically active (−)-monobromoagelaspongin (107), (−)-11-deoxymonobromoagelaspongin (108) and (−)-11-O-methylmonobromoagelaspongin (109).27 From a biosynthetic perspective, these dibromo- and monobromoagelaspongins were thought to be formed through cyclization and oxidation of oroidin/hymenidin.74,77,78
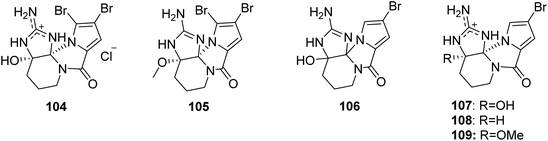 |
| Fig. 9 Chemical structures of 104–109. | |
2.2.2. Phakellin family. From Fig. 10—a series of phakellin family alkaloids (110–122) bearing a similar tetracyclic skeleton to that of agelaspongins, have been identified from Agelas sponges. Dibromophakellin (110), previously described from the sponge Phakellia flabellate,79 was obtained from Fijian A. mauritiana,47 while an examination of the extract of Caribbean Agelas sp. collected off Sweetings Cay resulted in isolation of monobromoisophakellin (111), along with previously reported monobromophakellin (112) and dibromoisophakellin (113).80 An anti-biofilm assay of the four phakellins (110–113) indicated that dibromophakellin and dibromoisophakellin significantly reduced the biofilm formation of E. coli, which was more active than the analogues with monobromo substitution.25 Additionally, results of antifeedant activity of these phakellins against Thalassoma bifasciatum suggested that the isophakelline skeleton showed a higher activity than that of phakelline skeleton, and bromination increases the antifeedant activity.80 Two N-methylated phakellin derivatives, (−)-7-N-methyldibromophakellin (114) and 7-N-methylmonobromophakellin (115) from Papua New Guinean Agelas sp., were obtained using a combination of bioassay- and LC-MS guided fractionation. Compound 114 had moderate inhibitory effect towards 12-human lipoxygenase isozymes (IC50, 10.7 ± 1.3 μM).81 A racemic mixture, dibromohydroxyphakellin (116) purified from Thousand Islands A. linnaei is the first 12-OH analogue of the phakellin family alkaloids.48 The report on an Indonesian sponge Agelas sp. collected in North Sulawesi, included 5-bromophakelline (117), as well as the known cylindradine A (118),60 which was previously isolated from the sponge Axinella cylindratus and was found to display moderate inhibitory activity against the murine leukemia cell line P388.82 Dibromocantharillin (119), firstly described from the sponge Pseudaxinyssa cantharella,83 was later purified from the Kosrae Island A. kosrae.84 Compound 119 not only showed antifungal activity against Cladosporium herbarum,85 but also displayed a significant inhibitory effect towards glycogen synthase kinase 3β (IC50, 3 μM).86 Another two congeners, 9-N-methylcylindradine A (120) and 1-N-methylugibohlin (121), were isolated from Xisha Islands A. nemoechinata, together with the known N-methyldibromoisophakellin (122),46 which was firstly found in the sponge Stylissa caribica.87
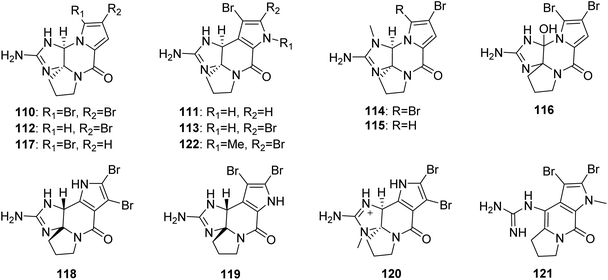 |
| Fig. 10 Chemical structures of 110–122. | |
2.2.3. Agelastatin family. From Fig. 11—as a most unusual member of fused cyclic pyrrole alkaloids, agelastatins A (123), B (124) and D–F (125–127) were discovered from New Caledonian A. dendromorpha, a species that has rarely been studied to date.88–90 These compounds structurally featured by a densely functionalized cyclopentane ring bearing four contiguous stereogenic centers substituted with nitrogen functionalities, and their conformational preference and absolute configuration were determined via combined molecular modelling, nuclear magnetic resonance, and exciton splitting for diamide and hydroxyamide derivatives.88 In addition to powerful cytotoxicity against a wide range of human cancer cell lines,89,91 agelastatin A (123) could also selectively inhibit glycogen synthase kinase-3β92 and was significantly toxic toward brine shrimp, larvae of beet army worm and corn rootworm.93 Comparative bioassays with semisynthetic derivatives showed that for high cytotoxic activity of 123, the unsubstituted OH–C(8a), H–N(5), and H–N(6) moieties are needed in the natural B/D transoid configuration.94 For biogenetic considerations, the agelastatin skeleton could be derived from oroidin/hymenidin through subtle tautomerization and the dual reactivity induced by the 2-aminoimidazole portion of the structure.74
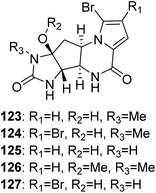 |
| Fig. 11 Chemical structures of 123–127. | |
2.2.4. Longamide family. From Fig. 12—the most common structural class of Agelas-derived fused cyclic pyrrole alkaloids were longamide family alkaloids, which are characterized by a pyrroloketopiperazine nucleus and could be derived from the cyclization of an oxidized oroidin-like fragment. A typical bicyclic pyrrole alkaloid, longamide (128) with moderate antibacterial activity was discovered in A. longissima, collected from Little San Salvador Island.95 Soon afterwards, the same research group found a mixture of enantiomers, longamide B (129), having moderate antibacterial activity against B. subtilis and S. aureus, from another Little San Salvador Island species A. dispar.33 A follow-up antiprotozoal activity screening revealed that 129 was a promising trypanocidal and antileishmanial agent, with narrower therapeutic windows, while 128 was completely inactive against any protozoan parasite.38 The monobrominated mukanadin C (130) and nonbrominated nemoechine B (131), were racemates obtained from the Okinawan A. nakamurai and the Xisha Islands Agelas aff. nemoechinata, respectively.41,56 The Indonesian A. nakamurai collected from the Menjangan Island produced longamide C (132).48 Bioassay-guided fractionation of the extract of the Xisha Islands Agelas sp. afforded another three new longamide family alkaloids, (±)-longamides D–F (133–135), together with the known (±)-hanishin (136) and (±)-longamide B methyl ester (137), previously discovered in the sponge Acanthella carteri collected from Hanish Islands96 and A. ceylonica collected near the Mandapam coast,97 respectively. In the experiment of antimicrobial activity, compound 137 possessed mild antibacterial activity against B. subtitles.97 Furthermore, the individual enantiomers (+)-(9S,10R)-133, (−)-S-134, (+)-R-135, (+)-R-136 and (+)-R-137 exhibited significant antifungal activity against C. albicans, whereas no activity was observed for some linear pyrrole alkaloids, suggesting that the pyrroloketopiperazine core positively affected the antifungal activity. In addition, the conclusion that the absolute configuration at C-9 of the intramolecular cyclized metabolites had an appreciable effect on the antifungal activity was reached, owing to the fact that no antifungal activity was found for individual enantiomers (−)-(9R,10S)-133, (+)-R-134, (−)-S-135, (−)-S-136 and (−)-S-137.43
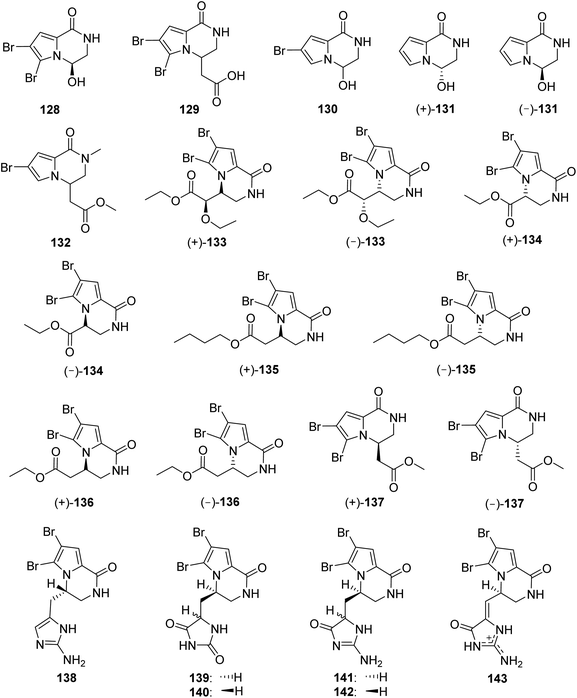 |
| Fig. 12 Chemical structures of 128–143. | |
The longamide family also includes compounds 138–143, featured by a pyrroloketopiperazine nucleus linked with an additional aminoimidazole/aminoimidazolone/hydantoin moiety. Cyclooroidin (138), bearing a methylenaminoimidazole unit was isolated from Mediterranean A. oroides.49 Diffusion-ordered NMR spectroscopy was applied for constituent analysis of crude pyrrole fractions from the Okinawan Agelas sp., resulting in the detection and isolation of two new stereoisomeric pyrroloketopiperazine derivatives with a hydantoin ring, namely agesamides A (139) and B (140).98 The congeners having a methylenaminoimidazolone unit, agesamines A (141) and B (142), were obtained as an inseparable epimeric mixture from Indonesian Agelas sp.,67 while a chemical investigation of the Mediterranean A. oroides yielded agesamine C (143).27
2.3. Dimeric pyrrole alkaloids
The dimeric pyrrole alkaloids are a growing class of alkaloids with exotic connectivity, unique structure, high nitrogen content, and exciting bioactivities. Structurally, some dimers were formed by cyclization of two monomers through a unique cyclic skeleton, such as cyclobutane, cyclohexene and pyrrolidine group, the others in which both monomers were linked by a single chemical bond, including C-8–C-15′, C-10–C-15′, C-11–C-15′ and C-15–C-15′. Although the biosynthesis of the complex pyrrole alkaloid dimers has been a subject of long debates, it is generally agreed that these metabolites could be derived from the three simple monomers (1–3) through oxidation, cyclization and dimerization reactions. For example, sceptrins featured by a cyclobutane core skeleton could be formed by [2 + 2] cycloaddition of the corresponding monomers, while ageliferins with a cyclohexene-based skeleton are formally the [4 + 2] cycloaddition products. Even after more than 40 years of research, the chemical diversity of dimer pyrrole alkaloids is still expanding, which have attracted many researchers to explore their biogenetic origin and structural relations.99
From Fig. 13—the study of A. sceptrum collected at Glover Reef afforded the first natural pyrrole-imidazole dimer, named sceptrin (144), which was generated from two molecules of hymenidin through a cyclobutane frame.100 However, until 2014, the mismatched chirality of sceptrin (144) was corrected by the intramolecular cycloaddition reaction in asymmetric syntheses, which was confirmed by the new crystal structure of sceptrin.101 Several years later, the di- and debromo analogues (145 and 146), and monooxygenated analogues of sceptrin with an aminoimidazolinone moiety (147 and 148), together with three structurally unique dimers, ageliferin (149), bromoageliferin (150) and dibromoageliferin (151), were identified both from Caribbean sponges A. conifera and A. cf. mauritiana.102,103 The ageliferins (149–151) with a rare cyclohexene-based skeleton were considered to be formed by [4 + 2] cycloaddition, although sceptrin-like alkaloids seem to be derived from [2 + 2] cycloaddition. In light of the revision of the absolute stereochemistry of 144, the absolute stereochemistry of 149–151 was also reversed.101 These metabolites were endowed with many valuable bioactivities. Compounds 144–151 were shown to be antiviral and antimicrobial and were active in barnacle settlement and biochemical prophage induction assays.102,104,105 Oxysceptrin (148) and ageliferins (149–151) were a potent actomyosin ATPase activator.106,107 As an anti-biofilm substance, compound 150 had the ability to inhibit the growth of marine bacteria with adhering properties.108 Compounds 150 and 151 could also inhibit voltage-operated, but not store-operated calcium entry in phaeochromocytoma PC12 cells.109 Additionally, Compounds 144, 145, 150 and 151 were revealed to be potent feeding deterrents against fish predation.19 The first sceptrin-type alkaloid without bromine substitution was debromosceptrin (152), isolated from the Caribbean A. conifer collected in Belize.110
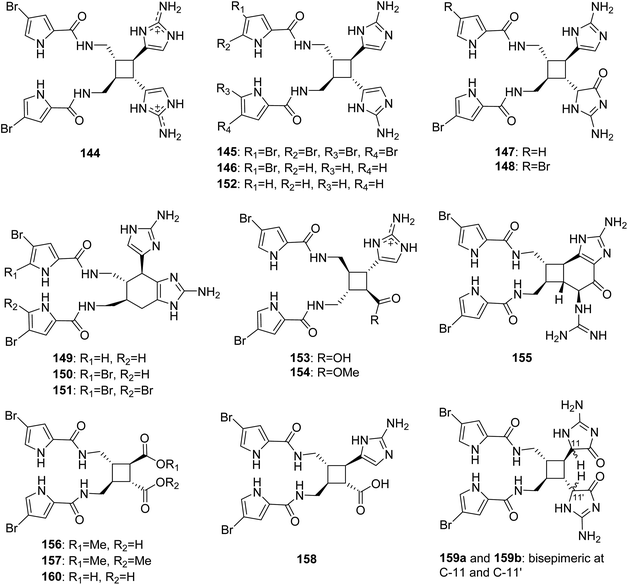 |
| Fig. 13 Chemical structures of 144–160. | |
The sceptrin derivatives with only one aminoimidazole ring in the molecule, nakamuric acid (153) and its corresponding methyl ester (154), without the assignment of absolute configuration, were obtained from A. nakamurai, collected near the island of Ambon, and both the compounds were shown to be active against the bacteria B. subtilis.105 A chemical investigation of Agelas sp. collected from Xisha Islands afforded several dimers, including hexazosceptrin (155) with a rare cyclohexane-fused-cyclobutane skeleton, two sceptrin derivatives without any aminoimidazole substitution, agelestes A (156) and B (157), along with (9S,10R,9′S,10′R)-nakamuric acid (158), of which the absolute configuration was assigned for the first time by comparison of experimental and calculated electronic circular dichroism (ECD). A subsequent bioactivity evaluation revealed that compounds 155 and 158 had moderate antimicrobial activity against S. aureus with the same MIC value of 16 μg mL−1.111 A purple elongated species that has rarely been studied to date, identified as A. kosrae, collected from Kosrae Island, was revealed to produce dioxysceptrin (159) and ageleste C (160). Compound 159, the dioxygenated form of sceptrin with two aminoimidazolinone moieties, was found to exist as a mixture of α-amido epimers and the absolute configuration was determined by ECD calculation. Both compounds 159 and 160 exhibited weak cytotoxicity against several cancer cell lines (including K562, A549, HCT116, MDA-MB-231, SNU 638 and SK-Hep-1). In addition, compounds 159 and 160 displayed moderate antiangiogenic and isocitrate lyase inhibitory activities, respectively.84
From Fig. 14—two unusual benzocyclobutane-containing natural products, benzosceptrin A (161) from Solomon Islands A. cf. mauritiana,112 and its brominated analogue benzosceptrin C (162) from both the Okinawan Agelas sp.113 and New Caledonian A. dendromorpha,90 have been identified. The bioactivities study revealed that compound 162 not only had antimicrobial activity against some pathogenic and environmental bacteria, quorum sensing inhibitory activity against Chromobacterium violaceum, but also inhibited the biofilm formation in Pseudomonas aeruginosa PAO1.27,113 Bioassay-guided isolation of extract from A. mauritiana collected off Hachijo-jima Island, led to the isolation of a new type of oxygenated oroidin dimer, mauritiamine (163), obtained as a racemate. Unlike the dimerization through a cyclobutane group for sceptrin-like alkaloids, compound 163 was constructed by two monomers with a linkage between C-11 in aminoimidazolinone unit and C-15′ in the aminoimidazole unit. Biological studies have identified that compound 163 could inhibit larval metamorphosis of the barnacle Balanus Amphitrite (ED50, 15 μg mL−1), and exhibit moderate antibacterial activity against Flavobacterium marinotypicum.17
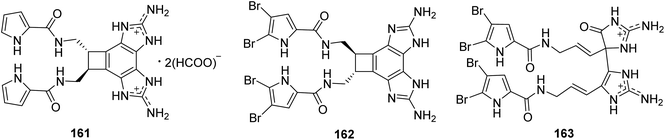 |
| Fig. 14 Chemical structures of 161–163. | |
From Fig. 15—twenty-two dimeric PIAs named nagelamides (164–185) have been identified from Agelas sponges. The Okinawan Agelas sp. was the source of nagelamides A–H (164–171). Of which, nagelamides A–D (164–167) were generated by two oroidin-type subunits through a single bond between C-10 and C-15′, while nagelamide H (171) with a taurine residue has a C-11–C-15′ linkage similar to 163. In the bioactivity assays, compounds 164–171 exhibited antibacterial activity against M. luteus, B. subtilis and E. coli (MIC, 2.08–33.3 μg mL−1). Compounds 164, 170 and 171 also inhibited protein phosphatase type 2A (IC50, 48, 13 and 46 μM, respectively).30 In addition, from the Okinawan collections of Agelas sp., the same research group obtained nagelamides I–L (172–175) and 2,2-didebromonagelamide B (176).114–116 Nagelamide I (172), a symmetric dimer of hymenidin, is the first example of PIAs consisting of two subunits connected with an uncommon connection of single bond between C-15 and C-15′ in the imidazole rings,114 while nagelamide J (173) represents the first pyrrole derivative with a cyclopentane-fused aminoimidazole system,115 nagelamide K (174) possesses a rare piperidinoiminoimidazolone ring with an aminoimidazole ring and a taurine unit, and nagelamide L (175) is a unique dimeric pyrrole alkaloid containing an ester linkage.116 Results of bioactivity tests suggested that compound 173 exhibited antimicrobial activity against S. aureus and C. neoformans (MIC, 8.35 and 16.7 μg mL−1, respectively),115 while compounds 174 and 175 were able to inhibit the growth of M. luteus (MIC, both 16.7 μg mL−1).116 Further studies on the Okinawan Agelas sponges, also by Kobayashi's group, have yielded nagelamides O–R (177–180).42,117 The structure of compound 177 is notable for bearing an uncommon perhydrocyclopenta-imidazo-azolo-imidazole carbon skeleton,42 while 179 contains a rare pyrrolidine ring, and 180 is the first example of bromopyrrole alkaloid having an oxazoline ring.117 Compound 177 was found to have weak antibacterial activity against B. subtilis, M. luteus and S. aureus (MIC, 33.3 μg mL−1, each),42 while compounds 179 and 180 were active against B. subtilis, T. mentagrophytes, C. neoformans, C. albicans and A. niger (MIC, 6–13 μg mL−1).117 Two nonbrominated congeners, nagelamides S (181) and T (182), biogenetically starting from clathrodin were sourced from Solomon Islands collection of A. cf. mauritiana.112 Kobayashi's group carried out a continued study on Okinawan Agelas sp., yielding three new structurally intriguing pyrrole-imidazole dimers, nagelamides X–Z (183–185). The racemates 183 and 184 both possess a rare tricyclic skeleton consisting of spiro-bonded tetrahydrobenzaminoimidazole and aminoimidazolidine moieties and seem to be derived from oroidin and taurodispacamide A by [4 + 2] cycloaddition, while compound 185, with the connectivity of C-8 to C-15′ between each oroidin unit, is the first pyrrole alkaloid involving the C-8 position in dimerization. Compounds 183–185 exhibited antimicrobial activities against some bacteria and fungi, with compound 185 being the most potent (the IC50 of 185 for C. albicans was 0.25 μg mL−1).118
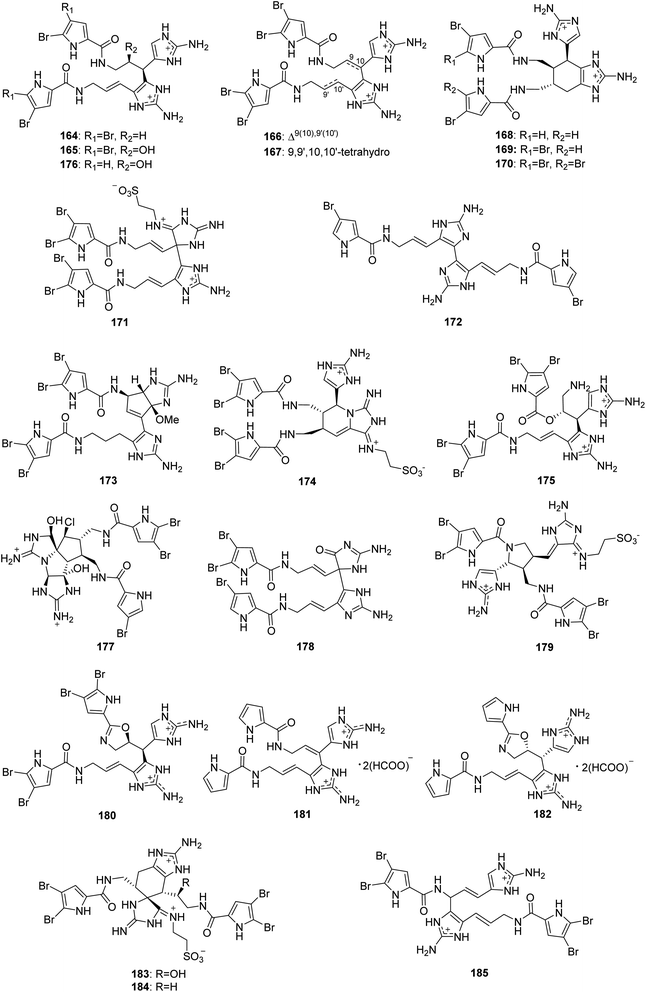 |
| Fig. 15 Chemical structures of 164–185. | |
From Fig. 16—the specimen A. citrina collected at San Salvador in the Bahamas was revealed to metabolize four hymenidin-type dimers that have different linkages between each monomeric unit, namely citrinamines A–D (186–189). Compounds 186 and 187 are closely related to 163 in which the monomeric units are connected by a single bond between C-11 and C-15′. Whereas compounds 188 and 189 show a different connection of the two monomeric hymenidin units (C-10/C-15′ and C-15/C-15′, respectively). In antimicrobial assays, the inhibitory effect against Mycobacterium phlei were observed for 187–189, while there was also an activity of 188 against M. luteus.65 The Mediterranean Sea A. oroides, collected off the Tel Aviv coast, contained another dimeric pyrrole alkaloid with a linkage of C-15 and C-15′ between each monomeric unit, namely dioroidamide A (190), which was constructed by the co-isolated linear monomer oroidin and fused cyclic monomer agesamine C derivative.27
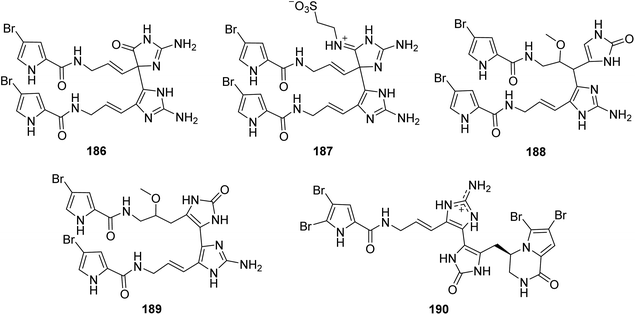 |
| Fig. 16 Chemical structures of 186–190. | |
From Fig. 17—Kobayashi's group also obtained five unique unsymmetrical pyrrole-imidazole dimers, agelamadins A–E (191–195), from the Okinawan Agelas sp.119,120 Of which, the racemates 191 and 192, with an agelastatin-like tetracyclic subunit and an oroidin-like linear subunit in common, exhibited antimicrobial activity against B. subtilis (MIC, 16 μg mL−1, each), M. luteus (MIC, 4.0 and 8.0 μg mL−1, respectively) and C. neoformans (IC50, 8.0 and 4.0 μg mL−1, respectively).119 The stereoisomeric 193–195 possess hybrid structures of oroidin and 3-hydroxykynurenine connected through a dihydro-1,4-oxazine moiety and their structure and absolute configuration were elucidated on the basis of spectroscopic analysis as well as application of a phenylglycine methyl ester method and ECD calculation. Biosynthetically, compounds 193–195 have been emerged as the first example for pyrrole alkaloids comprising oroidin and a tryptophan derivative to be reported. In antimicrobial screening, compounds 193 and 195 showed antimicrobial activity against C. neoformans (IC50, 32 μg mL−1, each).120 A closely related compound, decarboxyagelamadin C (196) has been sourced from Bahamas A. sceptrum, together with 15′-oxoadenosceptrin (197), which is the first pyrrole-imidazole derivative to incorporate adenine.121 Another unusual dimer, agelanemoechine (198), with an unprecedented imidazo [1,5-a] azepin nucleus was isolated from Xisha Islands A. nemoechinata. A possible biogenetic pathway of compound 198 was proposed starting from sceptrin through a key step of ring expansion reaction by rearrangement, which was different from the [2 + 2] and [4 + 2] cycloaddition encountered in the previously reported pyrrole alkaloid dimers. It is also worth noting that compound 198 showed a strong in vivo promoting angiogenesis activity in a zebrafish model.122
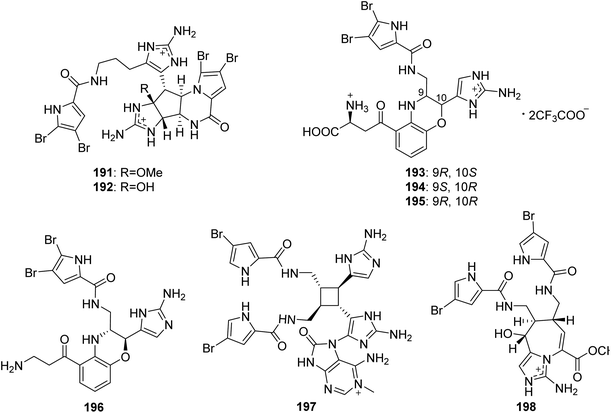 |
| Fig. 17 Chemical structures of 191–198. | |
3. Terpenoid alkaloids
Since Cullen and Devlin reported the isolation of agelasine, a quaternary 9-N-methyladenine derivative of an unidentified diterpene as a constituent of A. dispar in 1975,123 a number of unusual terpenoid alkaloids characterized by a polar functionality attached to a terpenoid moiety, have been found from Agelas sponges. Included among these were 9-N-methyladeninium terpenoids (i.e., agelasines, agelines, nemoechines) and terpenoids of hypotaurocyamine (i.e., agelasidines). The former usually carry a bicyclic diterpenoid skeleton (including clerodane, labdane and halimane) connected to the methyladenine moiety, while the latter featured by a branched diterpene with a hypotaurocyamine group. As the members of diterpenes, the 9-N-methyladeninium terpenoids likely arise from E,E,E-geranylgeranyl diphosphate (GGPP) to form the bicyclic diterpenoid moiety (Scheme 3).124,125 The presence of the unusual adenine in the structure made these compounds attractive targets in biological and chemical research. Recently there has been enormous interest in the bioactivities of 9-N-methyladeninium terpenoids owing to their noteworthy biological activities, including cytotoxic and antimicrobial activity and their action as Na+,K+-ATPase inhibitors.124 In contrast to the structural variety of the 9-N-methyladeninium terpenoid, only 10 terpenoids of hypotaurocyamine have been reported to date.
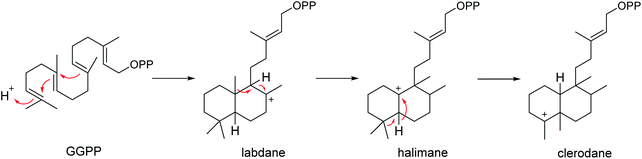 |
| Scheme 3 Origin of labdane, halimane and clerodane diterpenoid skeletons from geranylgeranyl diphosphate (GGPP). | |
3.1. 9-N-Methyladeninium terpenoids
From Fig. 18—agelasines A–F (199–204), possessing inhibitory effects against Na+,K+-ATPase, were isolated from Okinawan A. nakamurai, and their structures were solved by a comprehensive combination of spectral data and chemical conversions to establish the absolute configurations.126–128 Compounds 199 and 200 have a bicyclic clerodane diterpenoid moiety, while 201 and 202 possess a halimane and labdane diterpenoid skeleton, respectively, and 203 and 204 are unusual monocyclic diterpenoids of 9-N-methyladeninium. An additional bioactivity study suggested that compound 203 had antituberculosis activity against Mycobacterium tuberculosis,129 while compound 204 displayed a wide range of bioactivities including antimicrobial effect against S. aureus, B. subtilis and C. albicans, toxicity to goldfish Carassius auratus, antituberculosis activity against drug resistant M. tuberculosis, antitrypanosomal action against Trypanosoma brucei, as well as cytotoxicity against Jurkat cells.130,131 The specimen of Agelas sp. collected at Palau was found to yield agelines A (agelasine F, 204) and B (205). It is interesting to note that the latter compound showed clerodane diterpene merged with a pyrrole ring, not yet encountered in the family of 9-N-methyladeninium terpenoid alkaloids.132 A brominated analogue of 205, named agelasine G (206), exhibiting cytotoxic activity against murine lymphoma L1210 cells (IC50, 3.1 μg mL−1), was obtained from Okinawan Agelas sp.133 Further studies have identified compound 206 as being able to activate the insulin signaling pathway in Huh-7 human hepatoma cells by inhibiting PTP1B activity, while 205 exerted no inhibitory effect against PTP1B, suggesting that a Br atom plays a prominent role in the inhibition of PTP1B activity.134,135 Agelasines H (207) and I (208), both bearing a clerodane skeleton, were discovered from Agelas sp., collected at Yap Island.136 The Solomon Islands specimen of A. cf. mauritiana could metabolize agelasines J–L (209–211). Compound 209 possesses a halimane diterpenoid moiety, while compounds 210 and 211 are clerodane diterpenoid alkaloids. All the three metabolites (209–211) displayed antimalarial activity against Plasmodium falciparum with respective IC50 values of 6.6, 8.3 and 18 μM, and a low cytotoxicity on MCF7 human breast cancer cells.137
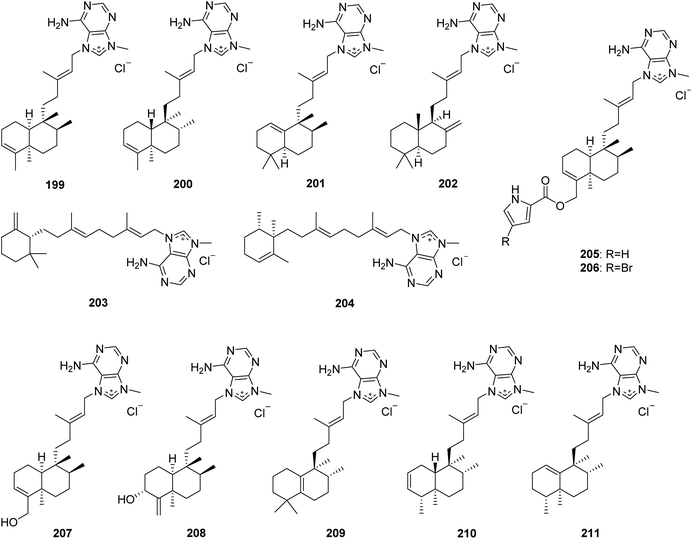 |
| Fig. 18 Chemical structures of 199–211. | |
From Fig. 19—a study on Pohnpei collection of A. mauritiana afforded epi-agelasine C (212), which was an antifouling substance active against macroalgae.138 The stereochemistry and absolute configuration of the halimane diterpenoid portion in compound 212 and the isomer agelasine C (201) were later reversed on the basis of chemical synthesis.139 The Eniwetak species A. mauritiana gave agelasimines A (213) and B (214),140 as well as an unusual purino-diterpene (215), the structure of which was determined by X-ray analysis.141 Compounds 213 and 214 not only had cytotoxic effect on L1210 leukemia cells, but also served as smooth muscle relaxants, Ca2+ channel antagonists and α1 adrenergic blockers.140,142 Chemical investigation of Indonesian A. nakamurai collected from Menjangan Island yielded (−)-agelasine D (216) and its analogue 217.48 Compound 217 was originally proposed as an oxime derivative and revised later as a formamide derivative of 216, thus renamed agelamide.143,144 Both compounds 216 and 217 exhibited cytotoxicity against L5178Y mouse lymphoma cells (IC50, 4.03 and 12.5 μM, respectively), and were toxic to the larvae rather than just inhibiting settlement of Balanus improvisus in an antifouling bioassay.48 Additionally, the therapeutic potential of agelamide (217) as a natural radiosensitizer in hepatocellular carcinoma models has been also revealed.144 The specimen Agelas sp. from Papua New Guinea was the source of another two agelasines with a clerodane diterpenoid moiety, agelasine M (218) and 2-oxo-agelasine B (219), along with the unusual norditerpenoid-agelasines, gelasines A (220) and B (221), among which compound 218 possessed significant inhibitory action against T. brucei (IC50, 3.0 μg mL−1) as well as mild cytotoxicity against Jurkat cells (IC50, 25 μg mL−1).130 An unusual thelepogane diterpene alkaloid (222) and agelasine N (223) with a hydroxy-substituted clerodane diterpenoid scaffold were reported from Papua New Guinean collections of A. nakamurai145 and A. citrina,146 respectively. Subsequently, Kobayashi's group described the isolation of agelasines O–U (224–230) from Okinawan Agelas sp. and the antimicrobial activity of compounds 224–227 and 229. The structures of 224–227 are notable for being 9-N-methyladenine diterpene alkaloids consisting of a bromopyrrole unit. In terms of the diterpenoid part, compound 224 has a halimane skeleton, while compounds 225–227 are clerodane diterpenoids. Compounds 228–230 contain a halimane, labdane, and clerodane skeleton, respectively.147
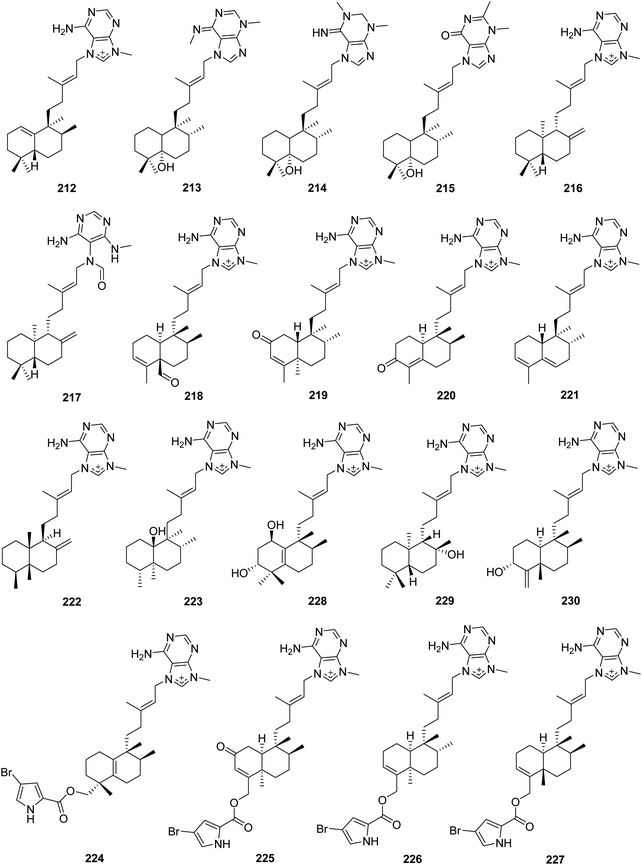 |
| Fig. 19 Chemical structures of 212–230. | |
From Fig. 20—three new agelasine derivatives, 2-oxoagelasine A (231), 2-oxoagelasine F (232) and 10-hydro-9-hydroxyagelasine F (233), together with series of known agelasines were isolated from Okinawan A. nakamurai. Compound 233 was found to inhibit the growth of Mycobacterium smegmatis, while the 2-oxoagelasines (231 and 232) exhibited markedly reduced activity against M. smegmatis, indicating that oxidation at the C-2 position of diterpenoid moiety was unfavorable for antimycobacterial activity of 9-N-methyladenine diterpenes.135 The alkaloid constituents of A. mauritiana collected from Xisha Islands were described and consisted of four 8′-oxo agelasines, namely (−)-8′-oxo-agelasines B–E (234–237), accompanied by (+)-agelasine B (238), agelasine V (239), and an oxime derivative ageloxime B (240). Further biological studies have identified that compound 238 exhibited moderate cytotoxicity toward the cancer cell lines PC9, A549, HepG2, MCF-7 and U937 (IC50, 4.49–14.07 μM), and also inhibited the growth of a panel of methicillin-resistant S. aureus clinical isolates (MIC90, 1–2 μg mL−1), while compound 240 showed antimicrobial activity against Cryptococcus neoformans, S. aureus and methicillin-resistant S. aureus and antileishmanial activity against Leishmania donovani. A subsequent structure–activity relationship analysis for 9-N-methyladenine diterpene alkaloids revealed that the presence of carbonyl at C-8′ in adenine can reduce antibacterial and cytotoxic activities.73,148 The species A. nakamurai, also collected from Xisha Islands could metabolize isoagelasine C (241), showing mild cytotoxic activities against HL-60, K562 and HCT-116 tumor cell lines as well as antimicrobial activities against C. albicans and Proteusbacillus vulgaris (MIC, 4.69 and 18.75 μg mL−1, respectively).44 Another Xisha Islands specimen of A. aff. nemoechinata produced nemoechines D ((−)-8′-oxo-agelasine B, 234), F (242) and G (243), with nemoechines D (234) and G (243) possessing cytotoxicity against HL-60 (IC50, 9.9 μM) and Jurkat cell lines (IC50, 17.1 μM), respectively.56,149 A bioassay-directed separation of the extract of A. axifera, collected from the Republic of Palau, allowed the identification of three unusual diterpenes with a seco-methyladenine moiety, axistatins 1–3 (244–246), which were found to inhibit the growth of a panel of murine and human cancer cells as well as some bacteria and fungi.150 Newly discovered agelasine derivatives included agelamasines A (247) and B (248), isolated from Okinawan Agelas sp. What's interesting is that compound 247, which may be generated from agelasine B (200) via oxidative cleavage followed by aldol condensation, featured by a rearranged (4 → 2)-abeo-clerodane diterpene skeleton that has no precedent in marine derived diterpene alkaloids.151
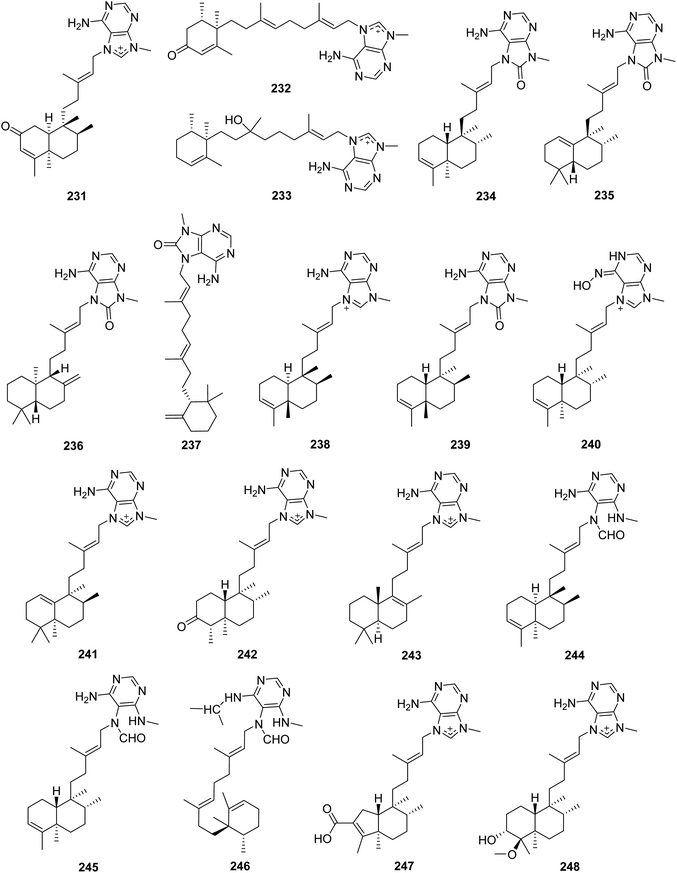 |
| Fig. 20 Chemical structures of 231–248. | |
3.2. Terpenoids of hypotaurocyamine
From Fig. 21—the first hypotaurocyamine terpenoid from natural source was agelasidine A (249) isolated from Okinawan Agelas sp., and the structure of this acyclic sesquiterpene with a guanidinylethysulfone unit was established by spectral analysis and chemical degradation.152 In addition to antispasmodic activity, compound 249 was also revealed to have antimicrobial effect against C. albicans, B. subtilis and S. aureus.132,152 A study on physiologically active metabolites of Okinawan A. nakamurai, by the same research group, resulted in the isolation of agelasidines B (250) and C (251), which were both found to have inhibitory effects on growth of microorganisms, contractile responses of smooth muscle and enzymic reactions of Na+,K+-ATPase.153 The Caribbean A. clathodes has been reported to yield three antibacterial analogues, (−)-agelasidine A (252), (−)-agelasidine C (253) and (−)-agelasidine D (254). The latter two compounds had potent cytotoxicity against CHO-K1 cells (respective ED50, 5.70 and 2.21 μg mL−1).154,155 Agelasidines E (255) and F (256) were discovered in another Caribbean specimen of A. citrina, and their absolute configurations were established by quantitative measurements of molar rotations based on van't Hoff's principle of optical superposition.146 The Xisha Islands A. mauritiana and A. nakamurai were found to produce (+)-2-oxo-agelasidine C (257)73 and isoagelasidine B (258),44 respectively. Compound 258 showed mild cytotoxic activities against HL-60 and K562 cell lines (IC50, 33.0 and 39.2 μM, respectively), as well as potent antifungal activities against C. albicans (MIC, 2.34 μg mL−1).44
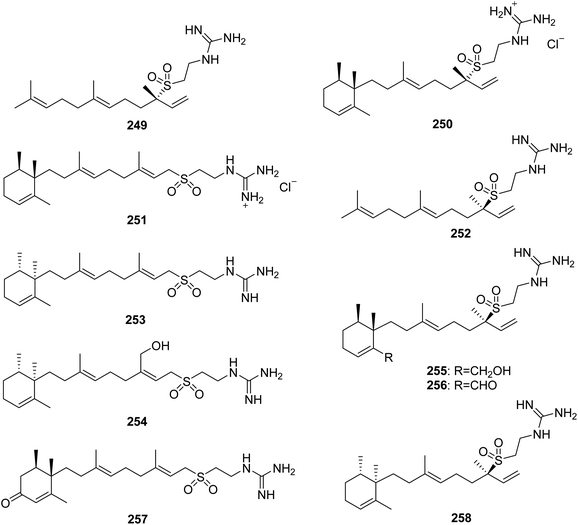 |
| Fig. 21 Chemical structures of 249–258. | |
3.3. Other terpenoid alkaloids
From Fig. 22—the sponge A. nakamurai, collected from Okinawa, metabolized a rare pyrrole-containing diterpene, named nakamurol D (259).156
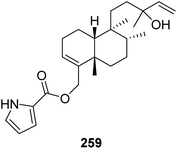 |
| Fig. 22 Chemical structure of 259. | |
4. Glycosphingolipids
From Fig. 23—the sponges belonging to the genus Agelas have the ability to synthesize a variety of unique glycosphingolipids (260–281). Eight agelasphins (260–267), with their absolute configuration elucidated by total synthesis, were isolated from the Okinawan A. mauritiana as active substances prolonging the life span of mice intraperitoneally inoculated with B16 mouse melanoma cells. These glycosphingolipids represent the first example of galactosylceramides containing an α-galactosyl linkage,157,158 and compound 262 has been served as a lead compound for further clinical application.159,160 The Puerto Rico collection of A. conifera was the source of β-galactosphingolipid coniferoside (268)161 and a mixture of homologs 269.162 Investigation of the glycosphingolipid composition of the Caribbean A. clathrodes revealed the presence of 270–277,163–165 of which clarhamnoside (271) is a tetraglycosylated α-galactoglycosphingolipid, containing an unusual L-rhamnose unit in the sugar head,163 while clathrosides A–C (272–274) and isoclathrosides A–C (275–277) are glycosides of a very-long-chain alcohol derived from fatty acids, a new class of glycolipids that appears to be characteristic of marine sponges.164 Additional research described by the same research group involved the congeners 278 and 279 from Caribbean A. longissima,166,167 and an immunomodulating compound 280 from the San Salvador Island A. dispar.168 A human cancer cell line bioassay-directed investigation of the Papua New Guinean Agelas sp. led to isolation of agelagalastatin (281), which is the first example of a natural product containing a digalactofuranosyl unit.169
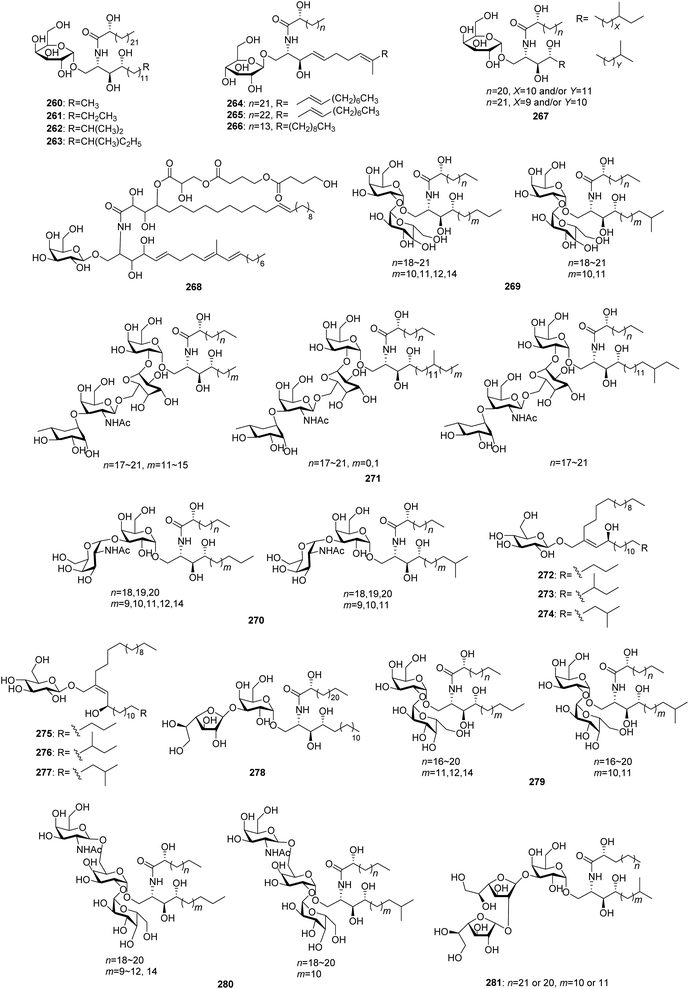 |
| Fig. 23 Chemical structures of 260–281. | |
5. Sterols
From Fig. 24—the species A. oroides was found to produce a group of sterols, including 282–296 from Naples specimen,170 and the Northern Aegean Sea derived 297, which exhibited antiplasmodial, as well as trypanocidal and leishmanicidal activities without cytotoxicity towards mammalian cells.21 The Bahamas A. flabelliformis was shown to contain 298, which was highly active in suppression of the response of murine splenocytes in the two-way mixed lymphocyte reaction with no associated toxicity when tested in a concentration range of 2.0 to 62.5 μg mL−1.59 Another examples of Agelas-derived sterols are 299–301 produced by Caribbean A. schmidtii,171 ecdysterone (302) and ajugasterone C (303) contained in Little San Salvador Island A. dispar,33 and 304–307 derived from Jamaican A. sceptrum.172
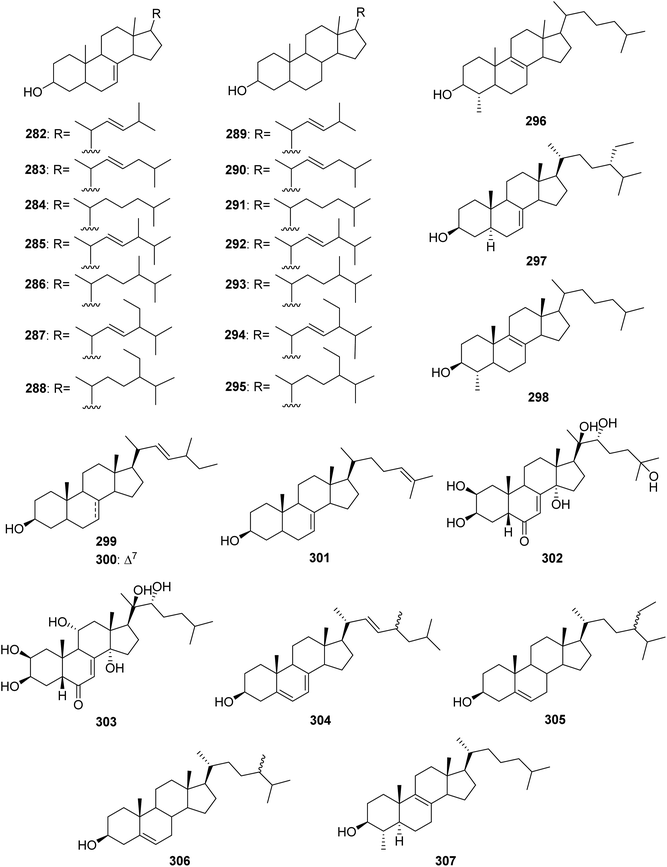 |
| Fig. 24 Chemical structures of 282–307. | |
6. Carotenoids
From Fig. 25—four carotenoids, namely α-carotene (308), isorenieratene (309), trikentriorhodin (310) and zeaxanthin (311), were identified from West Indies A. schmidtii,173 while the Kagoshima A. mauritiana was the source of isotedanin (312) and isoclathriaxanthin (313).174
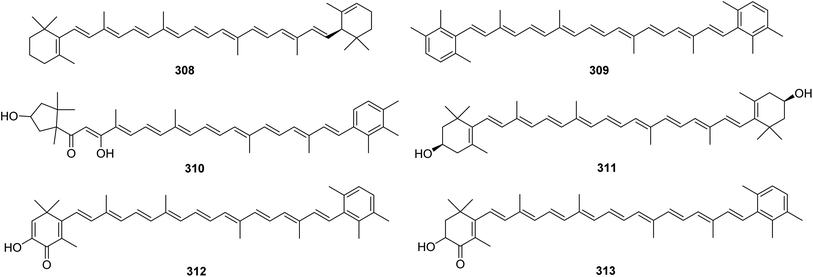 |
| Fig. 25 Chemical structures of 308–313. | |
7. Other types
From Fig. 26—an investigation of the chemistry of Great Barrier Reef A. oroides afforded three antibacterial fistularin-3 derivatives, including agelorins A (314) and B (315), and 11-epi-fistularin-3 (316),175 along with two volatile compounds, 2,4,6,6-tetramethyl-3(6H)-pyridone (317) and 2,2,6,6-tetramethyl-4-piperidone (318),176 while one imidazole derivative (319), taurine (320) and the fatty acids (321–324) were isolated from the Northern Aegean Sea collection of A. oroides,21 and the Mediterranean A. oroides contained several amino-acid derivatives, namely zwitterionic zooanemonin (325), N,N,N-trimetyl-β-alanin (326), 3-(2-aminoethyl)-11-dimethylurea (327), homarine (328), norzooanemonin (329) and (−)-equinobetaine (330).77 Compounds 319–324 showed antiplasmodial, trypanocidal and leishmanicidal activities.21 The Caribbean A. dispar could metabolize three new betaine alkaloids, called aminozooanemonin (331), pyridinebetaine A (332) and pyridinebetaine B (333), along with the known zooanemonin (325), homarine (328), trigonelline (334) and N,N,N-trimethyltaurine (335), with 331 and 332 showing antibacterial activity against B. subtilis and S. aureus (MIC, 2.5–8 μg mL−1).177 A study of A. longissima collected in Little San Salvador Island has resulted in 3,7-dimethylisoguanine (336) being reported.95 The Xisha Islands A. clathrodes was revealed to contain 337–339, with 338 exhibiting moderate cytotoxicity against cancer cell line SGC7901 (IC50, 13.2 μg mL−1).178 The identification of four brominated phospholipid fatty acids 340–343 from the Caribbean Agelas sp.179 and an anti-mycobacterial bisfunctionalized sphingolipid, leucettamol A (344), detected in another unclassified Indonesian Agelas specimen180 have been also achieved. The Okinawan A. nakamurai produced three uncommon diterpenoids, nakamurols A–C (345–347).156 In addition, some Agelas sponges were also found to metabolize polyketides, represented by gracilioethers A–C (348–350) from Amami-oshima Island A. gracilis,181 together with nemoechioxide A (351) and 352–355 from Xisha Islands A. aff. nemoechinata.56 Bioactivity tests indicated that compounds 348–350 showed antimalarial activity against Plasmodium falciparum (IC50, 0.5–10 μg mL−1), whereas 349 also possessed antileishmanial activity.181
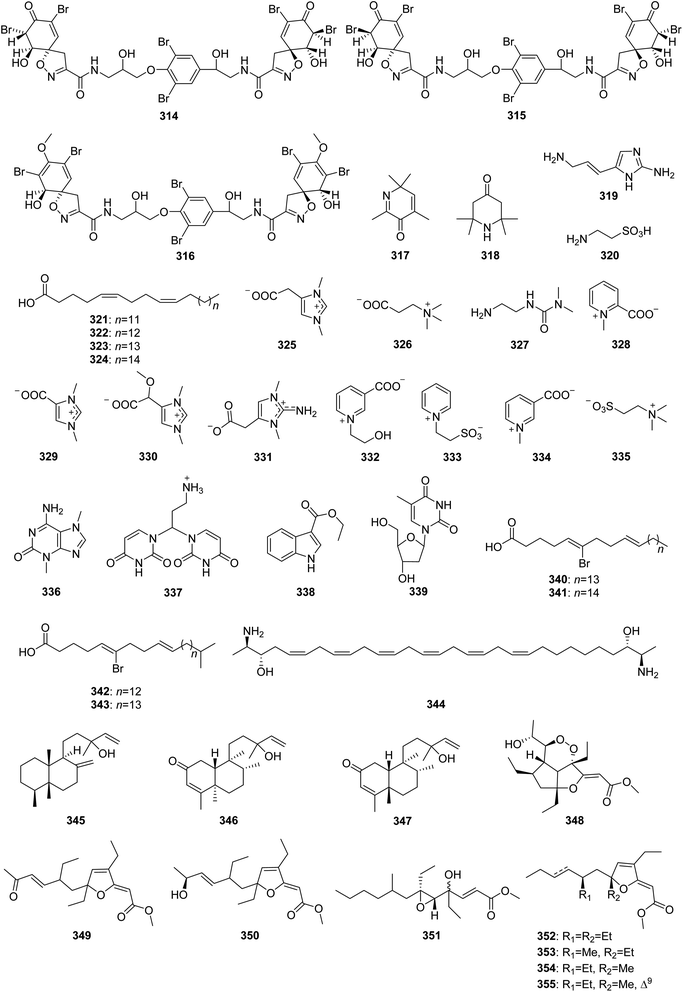 |
| Fig. 26 Chemical structures of 314–355. | |
8. Conclusions
Secondary metabolites isolated from marine sponges of the genus Agelas, from their first discovery to date, present an interesting case which has stimulated research advancements in the field of marine natural products. This review highlights the chemical diversity and biological activities of the 355 compounds isolated from Agelas sponges over the nearly five decades from 1971 to November 2021, covering 20 identified species and unclassified Agelas sp. (Table 1). The species A. oroides (15%), A. nakamurai (13%) and A. mauritiana (11%) are predominant producers of these diverse compounds, while the unclassified Agelas sp. are the source of 28% of the secondary metabolites from this genus (Fig. 27).
Table 1 Secondary metabolites isolated from Agelas sponges and their bioactivities
Compound |
Species |
Local of collection |
Bioactivity |
Ref. |
See main text for the bioactivities. Not reported. |
Pyrrole alkaloids |
1 |
A. oroides |
Naples |
Broad spectruma |
8, 9 and 16–27 |
2 |
A. clathrodes |
Desecheo Island |
Broad spectrum |
10, 23 and 28 |
3 |
A. sventres |
Bahamas |
Inhibitor of potassium channel |
11 and 23 |
4 |
Antipredatory |
11 |
5 |
Agelas sp. |
Okinawa |
Serotonergic receptors antagonist, antibacterial |
29, 31 and 33 |
6–8 |
NRb |
30 and 31 |
9 |
A. cf. mauritiana |
Solomon Islands |
NR |
32 |
10, 11 |
Agelas sp. |
Okinawa |
NR |
34 |
12 |
A. clathrodes, A. dispar, A. longissima, A. conifer |
Little San Salvador Island |
Antihistaminic, antipredatory |
35–37 |
13 |
Antihistaminic, antimalarial |
35, 36 and 38 |
14, 15 |
Antihistaminic |
36 |
16, 17 |
A. mauritiana |
Solomon Islands |
NR |
39 |
18 |
A. oroides |
Israeli Mediterranean |
NR |
27 |
19 |
Agelas sp. |
Okinawa |
NR |
34 |
20, 21 |
A. mauritiana |
Enewetak Atoll |
NR |
40 |
22 |
A. nakamurai |
Okinawa |
NR |
41 |
23 |
A. oroides |
Tel Aviv |
NR |
27 |
24 |
Agelas sp. |
Okinawa |
NR |
42 |
25 |
Agelas sp. |
Xisha Islands |
NR |
43 |
26, 27 |
A. nakamurai |
Xisha Islands |
NR |
44 and 45 |
28 |
A. nemoechinata |
Xisha Islands |
Cytotoxic |
46 |
29 |
A. mauritiana |
Fijian |
NR |
47 |
30, 31 |
A. linnaei |
Indonesia |
NR |
48 |
32 |
A. oroides |
Mediterranean |
Antihistaminic, antimicrobial |
16 and 49 |
33 |
Agelas sp. |
Okinawa |
Inhibitor of tyrosine kinase, antibacterial |
31 and 53 |
34–36 |
Agelas sp. |
Okinawa |
NR |
31 and 50 |
37, 38 |
Agelas sp. |
Okinawa |
Antimicrobial |
51 |
39 |
Agelas sp. |
Okinawa |
Antimicrobial |
52 |
40 |
NR |
41 |
Antimicrobial |
42 |
Agelas sp. |
Okinawa |
Antimicrobial |
31 |
43 |
Agelas sp. |
Okinawa |
NR |
50 |
44, 45 |
A. clathrodes |
Little San Salvador Island |
Antifungal |
54 |
46, 47 |
A. dispar |
Little San Salvador Island |
Antifungal |
33 |
48 |
A. nakamurai |
Okinawa |
NR |
55 |
49, 50 |
Cytotoxic |
51 |
A. oroides |
Tel Aviv |
NR |
27 |
52 |
A. aff. nemoechinata |
Xisha Islands |
NR |
56 |
53 |
A. nakamurai |
Kuchinoerabu-jima Island |
Antiangiogenic, fluorescent pH sensor |
57 and 58 |
54 |
A. linnaei |
Thousand Islands |
NR |
48 |
55 |
A. oroides |
Naples |
Immunosuppressive, antipredatory |
8, 18 and 59 |
56 |
Inhibitor of protein tyrosine phosphatase |
8 and 60 |
57 |
NR |
8 |
58 |
Promotor of ascidian larval metamorphosis, inhibitor of bacterial biofilm |
17 and 27 |
59, 60 |
A. oroides |
Great Barrier Reef |
NR |
61 |
61, 62 |
Agelas sp. |
NR |
NR |
62 |
63, 64 |
A. nakamurai |
Papua New Guinea |
Antimicrobial |
63 |
NR |
65, 66 |
A. nakamurai |
Menjangan Island |
NR |
48 |
67, 68 |
A. cerebrum |
Cuba |
NR |
64 |
69 |
A. mauritiana |
Enewetak Atoll |
NR |
40 |
70 |
A. longissima |
San Salvador Island |
Antiserotonergic |
66 |
71 |
A. citrina |
San Salvador Island |
NR |
65 |
72 |
Agelas sp. |
Likpan |
NR |
67 |
73 |
A. dispar |
Venezuelan island La Blanquilla |
Ligand and antagonist of several receptors |
68–70 |
74–77 |
A. linnaei |
Thousand Islands |
Cytotoxic |
48 |
78–81 |
NR |
82 |
A. wiedenmayeri |
Florida Keys |
NR |
72 |
83 |
Agelas sp. |
Okinawa |
NR |
42 |
84 |
A. oroides |
Tel Aviv |
NR |
27 |
85 |
A. mauritiana |
Xisha Islands |
NR |
73 |
86, 87 |
Agelas sp. |
Xisha Islands |
NR |
43 |
88–98 |
A. nakamurai |
Xisha Islands |
NR |
44 and 45 |
99–101 |
A. aff. nemoechinata |
Xisha Islands |
NR |
56 |
102, 103 |
Agelas sp. |
Okinawa |
NR |
34 |
104 |
Agelas sp. |
Tanzania sea |
Stimulator of wheat roots growth |
75 and 76 |
105 |
A. dispar |
Venezuelan island La Blanquilla |
NR |
68 |
106 |
A. oroides |
West Mediterranean |
NR |
77 |
107–109 |
A. oroides |
Tel Aviv |
NR |
27 |
110 |
A. mauritiana |
Fijian |
Inhibitor of bacterial biofilm, antipredatory |
25, 47 and 79 |
111–113 |
Agelas sp. |
Sweetings Cay |
Inhibitor of bacterial biofilm, antipredatory |
25 and 80 |
114 |
Agelas sp. |
Papua New Guinea |
Inhibitor of lipoxygenase isozymes |
81 |
115 |
NR |
116 |
A. linnaei |
Thousand Islands |
NR |
48 |
117, 118 |
Agelas sp. |
North Sulawesi |
Cytotoxic |
60 and 82 |
119 |
A. kosrae |
Kosrae Island |
Antifungal, inhibitor of glycogen synthase kinase |
84 and 85 |
120–122 |
A. nemoechinata |
Xisha Islands |
NR |
46 |
123 |
A. dendromorpha |
New Caledonia |
Cytotoxic, inhibitor of glycogen synthase kinase, toxic towards arthropods |
88, 89 and 91–93 |
124–127 |
NR |
88 and 90 |
128 |
A. longissima |
Little San Salvador Island |
Antibacterial |
95 |
129 |
A. dispar |
Little San Salvador Island |
Antibacterial, trypanocidal, antileishmanial |
33 and 38 |
130 |
A. nakamurai |
Okinawa |
NR |
41 |
131 |
Agelas aff. nemoechinata |
Xisha Islands |
NR |
56 |
132 |
A. nakamurai |
Menjangan Island |
NR |
48 |
133–136 |
Agelas sp. |
Xisha Islands |
antifungal |
43 |
137 |
Antibacterial, antifungal |
138 |
A. oroides |
Mediterranean |
NR |
49 |
139, 140 |
Agelas sp. |
Okinawa |
NR |
98 |
141, 142 |
Agelas sp. |
Indonesia |
NR |
67 |
143 |
A. oroides |
Mediterranean |
NR |
27 |
144 |
A. sceptrum |
Glover Reef |
Broad spectrum |
19, 100, 101, 104 and 105 |
145–151 |
A. conifera |
Caribbean |
Broad spectrum |
19, 102 and 103 |
152 |
A. conifera |
Belize |
NR |
110 |
153, 154 |
A. nakamurai |
Island of Ambon |
Antibacterial |
105 |
155 |
Agelas sp. |
Xisha Islands |
Antibacterial |
111 |
156, 157 |
NR |
158 |
Antibacterial |
159, 160 |
A. kosrae |
Kosrae Island |
Cytotoxic, antiangiogenic, inhibitor of isocitrate lyase |
84 |
161 |
A. cf. mauritiana |
Solomon Islands |
NR |
112 |
162 |
Agelas sp. |
Okinawa |
Antibacterial, quorum sensing inhibitory, inhibitor of bacterial biofilm |
27 and 113 |
163 |
A. mauritiana |
Hachijo-jima Island |
Antifouling, antibacterial |
17 |
164 |
Agelas sp. |
Okinawa |
Antibacterial, inhibitor of protein phosphatase |
30 |
165–169 |
Antibacterial |
170, 171 |
Antibacterial, inhibitor of protein phosphatase |
172 |
Agelas sp. |
Okinawa |
NR |
114–116 |
173–175 |
Antimicrobial |
176 |
NR |
177 |
Agelas sp. |
Okinawa |
Antibacterial |
42 and 117 |
178 |
NR |
179, 180 |
Antimicrobial |
181, 182 |
Agelas cf. mauritiana |
Solomon Islands |
NR |
112 |
183–185 |
Agelas sp. |
Okinawa |
Antimicrobial |
118 |
186 |
A. citrina |
San Salvador |
NR |
65 |
187–189 |
Antimicrobial |
190 |
A. oroides |
Tel Aviv |
NR |
27 |
191–195 |
Agelas sp. |
Okinawa |
Antimicrobial |
119 and 120 |
196, 197 |
A. sceptrum |
Bahamas |
NR |
121 |
198 |
A. nemoechinata |
Xisha Islands |
Proangiogenic |
122 |
![[thin space (1/6-em)]](https://www.rsc.org/images/entities/char_2009.gif) |
Terpenoid alkaloids |
199–202 |
A. nakamurai |
Okinawa |
Inhibitor of Na+,K+-ATPase |
126–128 |
203 |
Inhibitor of Na+,K+-ATPase, antituberculosis |
126–129 |
204 |
Broad spectrum |
126–128, 130 and 131 |
205 |
Agelas sp. |
Palau |
NR |
132 |
206 |
Agelas sp. |
Okinawa |
Cytotoxic, inhibitor of protein tyrosine phosphatase |
133 and 134 |
207, 208 |
Agelas sp. |
Yap Island |
NR |
136 |
209–211 |
A. cf. mauritiana |
Solomon Islands |
Antimalarial, cytotoxic |
137 |
212 |
A. mauritiana |
Pohnpei |
Antifouling |
138 |
213, 214 |
A. mauritiana |
Eniwetak |
Broad spectrum |
140 and 142 |
215 |
NR |
141 |
216 |
A. nakamurai |
Menjangan Island |
Cytotoxic, antifouling |
48 |
217 |
Cytotoxic, antifouling, radiosensitizer |
48 and 144 |
218 |
Agelas sp. |
Papua New Guinea |
Trypanocidal, cytotoxic |
130 |
219–221 |
NR |
222 |
A. nakamurai |
Papua New Guinea |
NR |
145 |
223 |
A. citrina |
Caribbean Sea |
NR |
146 |
224–227 |
Agelas sp. |
Okinawa |
Antimicrobial |
147 |
228 |
NR |
229, 230 |
Antimicrobial |
231–233 |
A. nakamurai |
Okinawa |
Antibacterial |
135 |
234–237 |
A. mauritiana |
Xisha Islands |
NR |
73 and 148 |
238 |
Cytotoxic, antibacterial |
239 |
NR |
240 |
Antibacterial, antileishmanial |
241 |
A. nakamurai |
Xisha Islands |
Cytotoxic, antimicrobial |
44 |
242 |
A. aff. nemoechinata |
Xisha Islands |
NR |
149 |
243 |
Cytotoxic |
244–246 |
A. axifera |
Republic of Palau |
Cytotoxic, antimicrobial |
150 |
247, 248 |
Agelas sp. |
Okinawa |
NR |
151 |
249 |
Agelas sp. |
Okinawa |
Antispasmodic, antimicrobial |
132 and 152 |
250, 251 |
A. nakamurai |
Okinawa |
Smooth muscle relaxant, inhibitor of Na+,K+-ATPase |
153 |
252 |
A. clathodes |
Caribbean |
NR |
154 and 155 |
253, 254 |
Cytotoxic |
255, 256 |
A. citrina |
Caribbean |
NR |
146 |
257 |
A. mauritiana |
Xisha Islands |
NR |
73 |
258 |
A. nakamurai |
Xisha Islands |
Cytotoxic, antifungal |
44 |
259 |
A. nakamurai |
Okinawa |
NR |
156 |
![[thin space (1/6-em)]](https://www.rsc.org/images/entities/char_2009.gif) |
Glycosphingolipids |
260–267 |
A. mauritiana |
Okinawa |
Cytotoxic |
157 and 158 |
268, 269 |
A. conifera |
Puerto Rico |
NR |
161 and 162 |
270–277 |
A. clathrodes |
Caribbean |
NR |
163–165 |
278, 279 |
A. longissima |
Caribbean |
NR |
166 and 167 |
280 |
A. dispar |
San Salvador Island |
Immunoactivating |
168 |
281 |
Agelas sp. |
Papua New Guinea |
NR |
169 |
![[thin space (1/6-em)]](https://www.rsc.org/images/entities/char_2009.gif) |
Sterols |
282–296 |
A. oroides |
Naples |
NR |
170 |
297 |
A. oroides |
Northern Aegean Sea |
Antiplasmodial, trypanocidal, leishmanicidal |
21 |
298 |
A. flabelliformis |
Bahamas |
Immunosuppressive |
59 |
299–301 |
A. schmidtii |
Caribbean |
NR |
171 |
302, 303 |
A. dispar |
Little San Salvador Island |
NR |
33 |
304–307 |
A. sceptrum |
Jamaica |
NR |
172 |
![[thin space (1/6-em)]](https://www.rsc.org/images/entities/char_2009.gif) |
Carotenoids |
308–311 |
A. schmidtii |
West Indies |
NR |
173 |
312, 313 |
A. mauritiana |
Kagoshima |
NR |
174 |
![[thin space (1/6-em)]](https://www.rsc.org/images/entities/char_2009.gif) |
Other types |
314, 315 |
A. oroides |
Great Barrier Reef |
Antibacterial |
175 |
316 |
Antibacterial, cytotoxic |
317, 318 |
NR |
176 |
319–324 |
A. oroides |
Northern Aegean Sea |
Trypanocidal, antiplasmodial, leishmanicidal |
21 |
325–330 |
A. oroides |
Mediterranean |
NR |
77 |
331, 332 |
A. dispar |
Caribbean |
Antibacterial |
177 |
333–335 |
NR |
336 |
A. longissima |
Little San Salvador Island |
NR |
95 |
337 |
A. clathrodes |
South China Sea |
NR |
178 |
338 |
Cytotoxic |
339 |
NR |
340–343 |
Agelas sp. |
Caribbean |
NR |
179 |
344 |
Agelas sp. |
Indonesia |
Anti-mycobacterial |
180 |
345–347 |
A. nakamurai |
Okinawa |
NR |
156 |
348 |
A. gracilis |
Amami-oshima Island |
Antimalarial |
181 |
349 |
Antimalarial, antileishmanial |
350 |
Antimalarial |
351–355 |
A. aff. nemoechinata |
Xisha Islands |
NR |
56 |
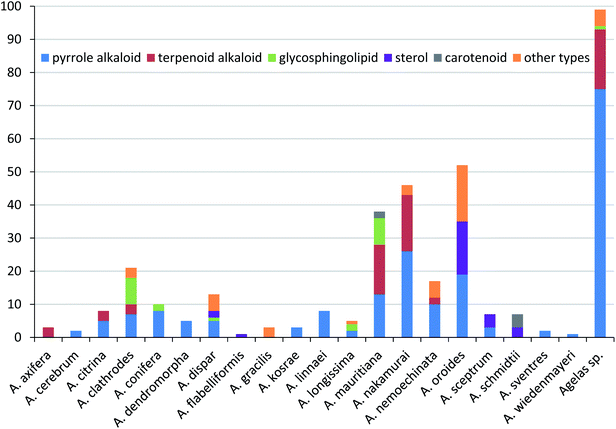 |
| Fig. 27 Agelas species distribution of isolated compounds belonging to the several chemical classes. | |
Pyrrole alkaloids are widely distributed in Agelas sponges and accounted for 56% of Agelas-derived secondary metabolites (198/355). The number of new members discovered still increases. The biogenetic key metabolite oroidin, which could be derived from proline and lysine, functions as basis of structural diversity on the skeleton level by undergoing cyclization, oxidation and dimerization to form an array of structurally complex pyrrole alkaloids. These pyrrole alkaloids can be classified as three structural types: linear pyrrole alkaloids, fused cyclic pyrrole alkaloids and dimeric pyrrole alkaloids, which account for about 52, 20 and 28% of the total pyrrole alkaloids, respectively. Another represented structural class is terpenoid alkaloids, with 17% of Agelas-derived secondary metabolites. In contrast to the structural variety of pyrrole alkaloids, only 61 terpenoid alkaloids have been reported to date, covering the species A. nakamurai (28%), A. mauritiana (25%), A. axifera (5%), A. citrina (5%), A. clathrodes (5%) and A. nemoechinata (3%). The remaining 29% were obtained from unclassified Agelas species (Fig. 27). These unusual terpenoid alkaloids mainly include 9-N-methyladeninium terpenoids and terpenoids of hypotaurocyamine, which account for about 82 and 16% of the total terpenoid alkaloids, respectively. It should be noted that, no terpenoid alkaloids have been found from the most prolific species A. oroides, which mainly metabolized pyrrole alkaloids, sterol and other types. In addition, the species A. clathrodes and A. mauritiana were also the source of nearly 73% of glycosphingolipids. The reasons for the biosynthesis of such characteristic metabolites within the multiple sponge species of the genus Agelas remains puzzling and deserves further study.
This review also involves the biological activities of 355 compounds. Bioactivity distribution of the pyrrole alkaloids and terpenoid alkaloids, which are the most important and representative secondary metabolites from Agelas sponges, revealed that the two families of alkaloids have different emphases on activity (Fig. 28). In terms of pyrrole alkaloids, the most prevalent activity was antimicrobial activity, which have been expressed by about 24% of the compounds. There were also pyrrole alkaloids endowed with various activities, including cytotoxic, enzyme inhibitory, antifouling, antiviral, bacterial biofilm inhibitory, and other activities (e.g., antihistaminic, antimalarial, antiangiogenic, proangiogenic, trypanocidal, antileishmanial and immunosuppressive effects). As for terpenoid alkaloids, about 21% were reported for cytotoxic activity, about 20% for antimicrobial activity, about 10% for enzyme inhibitory activity, and 22% for other activities, such as trypanocidal, antimalarial, antileishmanial and antispasmodic activities.
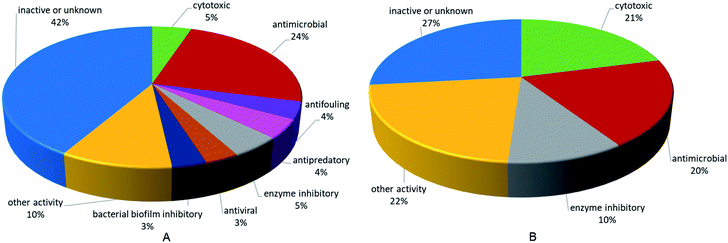 |
| Fig. 28 Bioactivity distribution of Agelas-derived pyrrole alkaloids (A) and terpenoid alkaloids (B). | |
Although these Agelas-derived secondary metabolites, especially pyrrole alkaloids and terpenoid alkaloids, are of historic importance in marine natural products chemistry, the unavailability of large amounts of natural alkaloids, challenges for purification due to numerous similar analogues, and structural and configurational complexity restricting efficient chemical synthesis have hindered further application in drug research and development. In recent years, with the increasing development of oceanographic science and spectroscopy technology, new intriguing Agelas-derived alkaloids are reported every year, making these marine sponges of this genus some of the most attractive targets for lead compounds discovery, worthy of further exploration. These findings, in conjunction with synthetic chemistry and biology, offer the potential to discover valuable molecules for future development in medicinal applications.
Conflicts of interest
There are no conflicts to declare.
Acknowledgements
The preparation of this review was supported by High-level Talents Scientific Research Foundation of Qingdao Agricultural University (grant number 663-1120014).
References
- D. J. Newman and G. M. Cragg, J. Nat. Prod., 2020, 83, 770–803 CrossRef CAS PubMed.
- A. R. Carroll, B. R. Copp, R. A. Davis, R. A. Keyzers and M. R. Prinsep, Nat. Prod. Rep., 2021, 38, 362–413 RSC.
- A. R. Carroll, B. R. Copp, R. A. Davis, R. A. Keyzers and M. R. Prinsep, Nat. Prod. Rep., 2020, 37, 175–223 RSC.
- R. Manconi, E. Perino and R. Pronzato, ZooKeys, 2016, 553, 1–31 CrossRef PubMed.
- H. Zhang, M. Dong, J. Chen, H. Wang, K. Tenney and P. Crews, Mar. Drugs, 2017, 15, 351 CrossRef PubMed.
- A. Al-Mourabit, M. A. Zancanella, S. Tilvi and D. Romo, Nat. Prod. Rep., 2011, 28, 1229–1260 RSC.
- E. Hao, F. Jane, J. Daniel and P. Karuso, Molecules, 2001, 6, 130–141 CrossRef CAS.
- S. Forenza, L. Minale, R. Riccio and F. Fattorusso, Chem. Commun., 1971, 18, 1129–1130 RSC.
- E. E. Garcia, L. E. Benjamin and R. I. Fryer, J. Chem. Soc., Chem. Commun., 1973, 3, 78–79 RSC.
- J. J. Morales and A. D. Rodríguez, J. Nat. Prod., 1991, 54, 629–631 CrossRef CAS.
- M. Assmann, S. Zea and M. Köck, J. Nat. Prod., 2001, 64, 1593–1595 CrossRef CAS PubMed.
- G. Genta-Jouve, N. Cachet, S. Holderith, F. Oberhänsli, J. L. Teyssié, R. Jeffree, A. Al Mourabit and O. P. Thomas, ChemBioChem, 2011, 12, 2298–2301 CrossRef CAS PubMed.
- I. Mohanty, S. G. Moore, D. Yi, J. S. Biggs, D. A. Gaul, N. Garg and V. Agarwal, ACS Chem. Biol., 2020, 15, 2185–2194 CrossRef CAS PubMed.
- E. P. Stout, B. I. Morinaka, Y. G. Wang, D. Romo and T. F. Molinski, J. Nat. Prod., 2012, 75, 527–530 CrossRef CAS PubMed.
- E. P. Stout, Y. G. Wang, D. Romo and T. F. Molinski, Angew. Chem., Int. Ed., 2012, 51, 4877–4881 CrossRef CAS PubMed.
- H. Zhang, Z. Khalil, M. M. Conte, F. Plisson and R. J. Capon, Tetrahedron Lett., 2012, 53, 3784–3787 CrossRef CAS.
- S. Tsukamoto, H. Kato, H. Hirota and N. Fusetani, J. Nat. Prod., 1996, 59, 501–503 CrossRef CAS.
- B. Chanas, J. R. Pawlik, T. Lindel and W. Fenical, J. Exp. Mar. Biol. Ecol., 1996, 208, 185–196 CrossRef.
- M. Assmann, E. Lichte, J. R. Pawlik and M. Köck, Mar. Ecol.: Prog. Ser., 2000, 207, 255–262 CrossRef CAS.
- E. Richelle-Maurer, M. J. De Kluijver, S. Feio, S. Gandencio, H. Gaspar, R. Gomez, R. Tavares, G. Van de Vyver and R. W. M. Van Soest, Biochem. Syst. Ecol., 2003, 31, 1073–1091 CrossRef CAS.
- D. Tasdemir, B. L. Topaloglu, R. Perozzo, R. Brun, R. O'Neill, N. M. Carballeira, X. Zhang, P. J. Tonge, A. Lindeng and P. Rüedi, Bioorg. Med. Chem., 2007, 15, 6834–6845 CrossRef CAS PubMed.
- I. E. Orhan, B. Ozcelik, B. Konuklugil, P. Orhan, K. Annika, G. Ulkua and P. Proksch, Rec. Nat. Prod., 2012, 6, 356–367 Search PubMed.
- N. Zidar, A. Žula, T. Tomašič, M. Rogers, R. W. Kirby, J. Tytgat, S. Peigneur, D. Kikelj, I. Janez and L. P. Mašič, Eur. J. Med. Chem., 2017, 139, 232–241 CrossRef CAS PubMed.
- J. J. Richards, T. E. Ballard, R. W. Huigens and C. Melander, ChemBioChem, 2008, 8, 1267–1279 CrossRef PubMed.
- R. J. Melander, H. Liu, M. D. Stephens, C. A. Bewley and C. Melander, Bioorg. Med. Chem. Lett., 2016, 26, 5863–5866 CrossRef CAS PubMed.
- J. Y. Sun, J. R. Wu, B. An, N. J. de Voogd, W. Cheng and W. H. Lin, Mar. Drugs, 2018, 16, 9 CrossRef PubMed.
- D. Kovalerchik, R. P. Singh, P. Schlesinger, A. Mahajni, S. Shefer, M. Fridman, M. Ilan and S. Carmeli, J. Nat. Prod., 2020, 83, 374–384 CrossRef CAS PubMed.
- A. L. Rentas, R. Rosa, A. D. Rodríguez and G. E. de Motta, Toxicon, 1995, 33, 491–497 CrossRef CAS PubMed.
- H. Nakamura, Y. Ohizumi and J. Kobayashi, Tetrahedron Lett., 1984, 25, 2475–2478 CrossRef CAS.
- T. Endo, M. Tsuda, T. Okada, S. Mitsuhashi, H. Shima, K. Kikuchi, Y. Mikami, J. Fromont and J. Kobayashi, J. Nat. Prod., 2004, 67, 1262–1267 CrossRef CAS PubMed.
- T. Kusama, N. Tanaka, A. Takahashi-Nakaguchi, T. Gonoi, J. Fromont and J. Kobayashi, Chem. Pharm. Bull., 2014, 62, 499–503 CrossRef CAS PubMed.
- C. Schroif-Gregoire, J. O. Appenzeller, C. Debitus, A. Zaparucha and A. Al-Mourabit, Tetrahedron, 2015, 71, 3609–3613 CrossRef CAS.
- F. Cafieri, E. Fattorusso and O. Taglialatela-Scafati, J. Nat. Prod., 1998, 61, 122–125 CrossRef CAS PubMed.
- S. Lee, N. Tanaka, S. Takahashi, D. Tsuji, S. Y. Kim, M. Kojoma, K. Itoh, J. Kobayashi and Y. Kashiwada, Mar. Drugs, 2020, 18, 455 CrossRef CAS PubMed.
- F. Cafieri, E. Fattorusso, A. Mangoni and O. Taglialatela-Scafati, Tetrahedron Lett., 1996, 37, 3587–3590 CrossRef CAS.
- F. Cafieri, R. Carnuccio, E. Fattorusso, O. Taglialatela-Scafati and T. Vallefuoco, Bioorg. Med. Chem. Lett., 1997, 7, 2283–2288 CrossRef CAS.
- T. Lindel, H. Hoffmann, M. Hochgürtel and J. R. Pawlik, J. Chem. Ecol., 2000, 26, 1477–1496 CrossRef CAS.
- F. Scala, E. Fattorusso, M. Menna, O. Taglialatela-Scafati, M. Tierney, M. Kaiser and D. Tasdemir, Mar. Drugs, 2010, 8, 2162–2174 CrossRef CAS PubMed.
- C. Vergne, J. Appenzeller, C. Ratinaud, M. T. Martin, C. Debitus, A. Zaparucha and A. Al-Mourabit, Org. Lett., 2008, 10, 493–496 CrossRef CAS PubMed.
- R. Fathi-Afshar and T. M. Allen, Can. J. Chem., 1988, 66, 45–50 CrossRef CAS.
- H. Uemoto, M. Tsuda and J. Kobayashi, J. Nat. Prod., 1999, 62, 1581–1583 CrossRef CAS PubMed.
- T. Yasuda, A. Araki, T. Kubota, J. Ito, Y. Mikami, J. Fromont and J. Kobayashi, J. Nat. Prod., 2009, 72, 488–491 CrossRef CAS PubMed.
- Y. Zhu, Y. Wang, B. B. Gu, F. Yang, W. H. Jiao, G. H. Hu, H. B. Yu, B. N. Han, W. Zhang, Y. Shen and H. W. Lin, Tetrahedron, 2016, 72, 2964–2971 CrossRef CAS.
- M. J. Chu, X. L. Tang, G. F. Qin, Y. T. Sun, L. Li, N. J. de Voogd, P. L. Li and G. Q. Li, Chem. Biodiversity, 2017, 14, e1600446 CrossRef PubMed.
- M. J. Chu, X. L. Tang, G. F. Qin, N. J. de Voogd, P. L. Li and G. Q. Li, Chin. Chem. Lett., 2017, 28, 1210–1213 CrossRef CAS.
- T. Li, P. L. Li, X. C. Luo, X. L. Tang and G. Q. Li, Tetrahedron Lett., 2019, 60, 1996–1998 CrossRef CAS.
- C. Jiménez and P. Crews, Tetrahedron Lett., 1994, 35, 1375–1378 CrossRef.
- T. Hertiani, R. Edrada-Ebel, S. Ortlepp, R. W. M. van Soest, N. J. de Voogd, V. Wray, U. Hentschel, S. Kozytska, W. E. G. Müller and P. Proksch, Bioorg. Med. Chem., 2010, 18, 1297–1311 CrossRef CAS PubMed.
- E. Fattorusso and O. Taglialatela-Scafati, Tetrahedron Lett., 2000, 41, 9917–9922 CrossRef CAS.
- T. Kusama, N. Tanaka, Y. Kashiwada and J. Kobayashi, Tetrahedron Lett., 2015, 56, 4502–4504 CrossRef CAS.
- T. Kubota, A. Araki, J. Ito, Y. Mikami, J. Fromont and J. Kobayashi, Tetrahedron, 2008, 64, 10810–10813 CrossRef CAS.
- N. Tanaka, T. Kusama, A. Takahashi-Nakaguchi, T. Gonoi, J. Fromont and J. Kobayashi, Tetrahedron Lett., 2013, 54, 3794–3796 CrossRef CAS.
- J. Kobayashi, K. Lnaba and M. Tsuda, Tetrahedron, 1997, 53, 16679–16682 CrossRef CAS.
- F. Cafieri, E. Fattorusso, A. Mangoni and O. Taglialatela-Scafafi, Tetrahedron, 1996, 52, 13713–13720 CrossRef CAS.
- M. Tsuda, H. Uemoto and J. Kobayashi, Tetrahedron Lett., 1999, 40, 5709–5712 CrossRef CAS.
- L. An, W. J. Song, X. L. Tang, N. J. de Voogd, Q. Wang, M. J. Chu, P. L. Li and G. Q. Li, RSC Adv., 2017, 7, 14323–14329 RSC.
- M. Fujita, Y. Nakao, S. Matsunaga, M. Seiki, Y. Itoh, J. Yamashita, R. W. M. van Soest and N. Fusetani, J. Am. Chem. Soc., 2003, 125, 15700–15701 CrossRef CAS PubMed.
- U. Bickmeyer, A. Grube, K. Klings and M. Köck, Biochem. Biophys. Res. Commun., 2008, 373, 419–422 CrossRef CAS PubMed.
- S. P. Gunasekera, S. Cranick and R. E. Longley, J. Nat. Prod., 1989, 52, 757–761 CrossRef CAS PubMed.
- D. B. Abdjul, H. Yamazaki, S. Kanno, A. Tomizawa, H. Rotinsulu, D. S. Wewengkang, D. A. Sumilat, K. Ukai, M. M. Kapojos and M. Namikoshi, J. Nat. Med., 2017, 71, 531–536 CrossRef CAS PubMed.
- G. M. König and A. D. Wright, Nat. Prod. Lett., 1994, 5, 141–146 CrossRef.
- H. Tada and T. Tozyo, Chem. Lett., 1988, 117, 803–804 CrossRef.
- T. Iwagawa, M. Kaneko, H. Okamura, M. Nakatani and R. W. M. van Soest, J. Nat. Prod., 1998, 61, 1310–1312 CrossRef CAS PubMed.
- E. L. Regalado, A. Laguna, J. Mendiola, O. P. Thomas and C. Nogueiras, Quim. Nova, 2011, 34, 289–291 CrossRef CAS.
- C. Cychon, E. Lichte and M. Köck, J. Org. Chem., 2015, 11, 2029–2037 Search PubMed.
- F. Cafieri, A. Mangoni, O. Taglialatela-Scafati and R. Carnuccio, Bioorg. Med. Chem. Lett., 1995, 5, 799–804 CrossRef CAS.
- A. Katsuki, H. Kato, Y. Ise, F. Losung, R. E. P. Mangindaan and S. Tsukamoto, Heterocycles, 2019, 98, 558–563 CrossRef CAS.
- I. C. Piña, K. N. White, G. Cabrera, E. Rivero and P. Crews, J. Nat. Prod., 2007, 70, 613–617 CrossRef PubMed.
- J. P. Kennedy, J. T. Brogan and C. W. Lindsley, J. Nat. Prod., 2008, 71, 1783–1786 CrossRef CAS PubMed.
- J. P. Kennedy, P. J. Conn and C. W. Lindsley, Bioorg. Med. Chem. Lett., 2009, 19, 3204–3208 CrossRef PubMed.
- J. Zeng and J. Zhan, Isr. J. Chem., 2019, 59, 387–402 CrossRef CAS.
- M. Assmann, E. Lichte, R. W. M. van Soest and M. Köck, Org. Lett., 1999, 1, 455–457 CrossRef CAS.
- F. Yang, M. T. Hamann, Y. Zou, M. Y. Zhang, X. B. Gong, J. R. Xiao, W. S. Chen and H. W. Lin, J. Nat. Prod., 2012, 75, 774–778 CrossRef CAS PubMed.
- A. A. Mourabit and P. Potier, Eur. J. Org. Chem., 2001, 2, 237–243 CrossRef.
- S. A. Fedoreyev, S. G. Ilyin, N. K. Utkina, O. B. Maximov, M. V. Reshetnyak, M. Y. Antipin and Y. T. Struchkov, Tetrahedron, 1989, 45, 3487–3492 CrossRef.
- M. M. Anisimov, E. L. Chaikina and N. K. Utkina, Nat. Prod. Commun., 2014, 9, 459–460 CAS.
- P. Sauleau, C. Moriou and A. Al Mourabit, Nat. Prod. Res., 2017, 31, 1625–1632 CrossRef CAS PubMed.
- S. Picon, E. T. H. Dau, M. T. Martin, P. Retailleau, A. Zaparucha and A. Al-Mourabit, Org. Lett., 2009, 12, 2523–2526 CrossRef PubMed.
- G. M. Sharm and P. R. Burkhold, Chem. Commun., 1971, 3, 151–152 RSC.
- M. Assmann and M. Köck, Z. Naturforsch., C: J. Biosci., 2002, 57, 153–156 CrossRef CAS PubMed.
- J. T. Gautschi, S. Whitman, T. R. Holman and P. Crew, J. Nat. Prod., 2004, 67, 1256–1261 CrossRef CAS PubMed.
- M. Kuramoto, N. Miyake, Y. Ishimaru, N. Ono and H. Uno, Org. Lett., 2008, 23, 5465–5468 CrossRef PubMed.
- G. De Nanteuil, A. Ahond, J. Guilhem, C. Poupat, E. T. H. Dau, P. Potier, M. Pusset, J. Pusset and P. Laboute, Tetrahedron, 1985, 4, 6019–6033 CrossRef.
- O. S. Kwon, D. Kim, H. Kim, Y. J. Lee, H. S. Lee, C. J. Sim, D. C. Oh, S. K. Lee, K. B. Oh and J. Shin, Mar. Drugs, 2018, 16, 513 CrossRef CAS PubMed.
- W. Hassan, E. Elkhayata, R. A. Edrada, R. Ebel and P. Proksch, Nat. Prod. Commun., 2007, 2, 1149–1154 CrossRef CAS.
- N. Zhang, R. Zhong, H. Yan and Y. Jiang, Chem. Biol. Drug Des., 2011, 77, 199–205 CrossRef CAS PubMed.
- M. Assmann, R. W. M. van Soest and M. Köck, J. Nat. Prod., 2001, 64, 1345–1347 CrossRef CAS PubMed.
- M. D’Ambrosio, A. Guerriero, G. Chiasera and F. Pietra, Anal. Chim. Acta, 1994, 77, 1895–1902 Search PubMed.
- M. D'Ambrosio, A. Guerriero, C. Debitus, O. Ribes, J. Pusset, S. Leroy and F. Pietra, J. Chem. Soc., Chem. Commun., 1993, 16, 1305–1306 RSC.
- S. Tilvi, C. Moriou, M. T. Martin, J. F. Gallard, J. Sorres, K. Patel, S. Petek, C. Debitus, L. Ermolenko and A. Al-Mourabit, J. Nat. Prod., 2010, 73, 720–723 CrossRef CAS PubMed.
- C. K. Mason, S. McFarlane, P. G. Johnston, P. Crowe, P. J. Erwin, M. M. Domostoj, F. C. Campbell, S. Manaviazar, K. J. Hale and M. El-Tanani, Mol. Cancer Ther., 2008, 7, 548–558 CrossRef CAS PubMed.
- L. Meijer, A. M. Thunnissen, A. W. White, M. Garnier, M. Nikolic, L. H. Tsai, J. Walter, K. E. Cleverlry, P. C. Salinas, Y. Z. Wu, J. Biernat, E. M. Mandelkow, S. H. Kim and G. R. Pettit, Chem. Biol., 2000, 7, 51–63 CrossRef CAS PubMed.
- T. W. Hong, D. R. Jímenez and T. F. Molinski, J. Nat. Prod., 1998, 61, 158–161 CrossRef CAS.
- M. D'Ambrosio, A. Guerriero, M. Ripamontib, C. Debitus, J. Waikedre and P. Francesco, Helv. Chim. Acta, 1996, 79, 727–735 CrossRef.
- F. Cafieri, E. Fattorusso, A. Mangoni and O. Taglialatela-Scafati, Tetrahedron Lett., 1995, 36, 7893–7896 CrossRef CAS.
- I. Mancini, G. Guella, P. Amade, C. Roussakis and F. Pietra, Tetrahedron Lett., 1997, 38, 6271–6274 CrossRef CAS.
- N. S. Reddy and Y. Venkateswarlu, Biochem. Syst. Ecol., 2000, 28, 1035–1037 CrossRef CAS.
- M. Tsuda, T. Yasuda, E. Fukushi, J. Kawabata, M. Sekiguchi, J. Fromont and J. Kobayashi, Org. Lett., 2006, 8, 4235–4238 CrossRef CAS PubMed.
- X. Wang, Z. Ma, X. Wang, S. De, Y. Ma and C. Chen, Chem. Commun., 2014, 50, 8628–8639 RSC.
- R. P. Walker, D. J. Faulkner, D. Van Engen and J. Clardy, J. Am. Chem. Soc., 1981, 103, 6772–6773 CrossRef CAS.
- Z. Ma, X. Wang, X. Wang, R. A. Rodriguez, C. E. Moore, S. Gao, X. Tan, Y. Ma, A. L. Rheingold, P. S. Baran and C. Chen, Science, 2014, 346, 219–224 CrossRef CAS PubMed.
- P. A. Keifer, R. E. Schwartz, M. E. S. Koker, R. G. Hughes Jr, D. Rittschof and K. L. Rinehart, J. Org. Chem., 1991, 56, 2965–2975 CrossRef CAS.
- K. L. Rinehart, Pure Appl. Chem., 1989, 61, 525–528 CrossRef CAS.
- R. P. Walker and D. J. Faulkner, J. Am. Chem. Soc., 1981, 103, 6773–6775 CrossRef.
- C. Eder, P. Proksch, V. Wray, R. W. M. van Soest, E. Ferdinandus, L. A. Pattisina and S. Sudarsono, J. Nat. Prod., 1999, 62, 1295–1297 CrossRef CAS PubMed.
- J. Kobayashi, M. Tsuda, T. Murayama, H. Nakamura, Y. Ohizumi, M. Ishibashi, M. Iwamura, T. Ohta and S. Nozoe, Tetrahedron, 1990, 46, 5579–5586 CrossRef CAS.
- J. Kobayashi, M. Tsuda and Y. Ohizumi, Experientia, 1991, 47, 301–304 CrossRef CAS PubMed.
- A. Yamada, H. Kitamura, K. Yamaguchi, S. Fukuzawa, C. Kamijima, K. Yazawa, M. Kuramoto, G. Y. S. Wang, Y. Fujitani and D. Uemura, Bull. Chem. Soc. Jpn., 1997, 70, 3061–3069 CrossRef CAS.
- U. Bickmeyer, Toxicon, 2005, 45, 627–632 CrossRef CAS PubMed.
- X. Shen, T. L. Perry, C. D. Dunbar, M. Kelly-Borges and M. T. Hamann, J. Nat. Prod., 1998, 61, 1302–1303 CrossRef CAS.
- Y. T. Sun, B. Lin, S. G. Li, M. Liu, Y. J. Zhou, Y. Xu, H. M. Hua and H. W. Lin, Tetrahedron, 2017, 73, 2786–2792 CrossRef CAS.
- J. R. Appenzeller, S. Tilvi, M. T. Martin, J. F. Gallard, H. El-bitar, E. T. H. Dau, C. Debitus, D. Laurent, C. Moriou and A. Al-Mourabit, Org. Lett., 2009, 11, 4874–4877 CrossRef CAS PubMed.
- T. Kubota, A. Araki, T. Yasuda, M. Tsuda, J. Fromont, K. Aoyama, Y. Mikami, M. R. Wälchli and J. Kobayashi, Tetrahedron Lett., 2009, 50, 7268–7270 CrossRef CAS.
- T. Iwai, T. Kubota, J. Fromont and J. Kobayashi, Chem. Pharm. Bull., 2014, 62, 213–216 CrossRef CAS PubMed.
- A. Araki, M. Tsuda, T. Kubota, Y. Mikami, J. Fromont and J. Kobayashi, Org. Lett., 2007, 9, 2369–2371 CrossRef CAS PubMed.
- A. Araki, T. Kubota, M. Tsuda, Y. Mikami, J. Fromont and J. Kobayashi, Org. Lett., 2008, 10, 2099–2102 CrossRef CAS PubMed.
- A. Araki, T. Kubota, K. Aoyama, Y. Mikami, J. Fromont and J. Kobayashi, Org.
Lett., 2009, 11, 1785–1788 CrossRef CAS PubMed.
- N. Tanaka, T. Kusama, A. Takahashi-Nakaguchi, T. Gonoi, J. Fromont and J. Kobayashi, Org. Lett., 2013, 15, 3262–3265 CrossRef CAS PubMed.
- T. Kusama, N. Tanaka, K. Sakai, T. Gonoi, J. Fromont, Y. Kashiwada and J. Kobayashi, Org. Lett., 2014, 16, 3916–3918 CrossRef CAS PubMed.
- T. Kusama, N. Tanaka, K. Sakai, T. Gonoi, J. Fromont, Y. Kashiwada and J. Kobayashi, Org. Lett., 2014, 16, 5176–5179 CrossRef CAS PubMed.
- J. Muñoz and M. Köck, J. Nat. Prod., 2016, 79, 434–437 CrossRef PubMed.
- T. Li, X. L. Tang, X. C. Luo, Q. Wang, K. C. Liu, Y. Zhang, N. J. de Voogd, J. J. Yang, P. L. Li and G. Q. Li, Org. Lett., 2019, 21, 9483–9486 CrossRef CAS PubMed.
- E. Cullen and J. P. Devlin, Can. J. Chem., 1975, 53, 1690–1691 CrossRef CAS.
- A. M. Roncero, I. E. Tobal, R. F. Moro, D. Díez and I. S. Marcos, Nat. Prod. Rep., 2018, 35, 955–991 RSC.
- R. Li, S. L. Morris-Natschke and K. H. Lee, Nat. Prod. Rep., 2016, 33, 1166–1226 RSC.
- H. Nakamura, H. Wu, Y. Ohizumia and Y. Hirata, Tetrahedron Lett., 1984, 25, 2989–2992 CrossRef CAS.
- H. Wu, H. Nakamura, J. Kobayashi and Y. Ohizumi, Tetrahedron Lett., 1984, 25, 3719–3722 CrossRef CAS.
- H. Wu, H. Nakamura, J. Kobayashi, M. Kobayashi, Y. Ohizumi and Y. Hirata, Bull. Chem. Soc. Jpn., 1986, 59, 2495–2504 CrossRef CAS.
- A. K. Bakkestuen, L. Gundersen, D. Petersen, B. T. Utenova and A. Vik, Org. Biomol. Chem., 2005, 3, 1025–1033 RSC.
- L. Calcul, K. Tenney, J. Ratnam, J. H. McKerrow and P. Crews, Aust. J. Chem., 2010, 63, 915–921 CrossRef CAS.
- G. C. Manglindan, M. T. Talaue, L. J. Cruz, S. G. Franzblau, L. B. Adams, A. D. Richardson, C. M. Ireland and G. P. Concepcion, Planta Med., 2000, 66, 363–365 Search PubMed.
- R. J. Capon and D. J. Faulkner, J. Am. Chem. Soc., 1984, 106, 1819–1822 CrossRef CAS.
- K. Ishida, M. Ishibashi, H. Shigemori, T. Sasaki and J. Kobayashi, Chem. Pharm. Bull., 1992, 40, 766–767 CrossRef CAS PubMed.
- H. Yamazaki, S. Kanno, D. B. Abdjul and M. Namikoshi, Bioorg. Med. Chem. Lett., 2017, 27, 2207–2209 CrossRef CAS PubMed.
- D. B. Abdjul, H. Yamazaki, S. Kanno, O. Takahashi, R. Kirikoshi, K. Ukai and M. Namikoshi, J. Nat. Prod., 2015, 78, 1428–1433 CrossRef CAS PubMed.
- X. Fu, F. J. Schmitz, R. S. Tanner and M. Kelly-Borges, J. Nat. Prod., 1998, 61, 548–550 CrossRef CAS PubMed.
- J. Appenzeller, G. Mihci, M. T. Martin, J. F. Gallard, J. L. Menou, N. Boury-Esnault, J. Hooper, S. Petek, S. Chevalley, A. Valentin, A. Zaparucha, A. Al-Mourabit and C. Debitus, J. Nat. Prod., 2008, 71, 1451–1454 CrossRef CAS PubMed.
- T. Hattori, K. Adachi and Y. Shizuri, J. Nat. Prod., 1997, 60, 411–413 CrossRef CAS.
- I. S. Marcos, N. García, M. J. Sexmero, P. Basabe, D. Díez and J. G. Urones, Tetrahedron, 2005, 61, 11672–11678 CrossRef CAS.
- R. Fathi-Afshar and T. M. Allen, Can. J. Chem., 1988, 66, 45–50 CrossRef CAS.
- T. Nakatsu and D. J. Faulkner, Tetrahedron Lett., 1984, 25, 935–938 CrossRef CAS.
- R. Fathi-Afshar, T. M. Allen, C. A. Krueger, D. A. Cook, A. S. Clanachan, R. Vriend, H. P. Baer and C. E. Cass, Can. J. Physiol. Pharmacol., 1989, 67, 276–281 CrossRef CAS PubMed.
- B. Paulsen, K. A. Fredriksen, D. Petersen, L. Maes, A. Matheeussen, A. O. Naemi, A. A. Scheie, R. Simm, R. Mad, B. Wand, S. Franzblaud and L. L. Gundersen, Bioorg. Med. Chem., 2019, 27, 620–629 CrossRef CAS PubMed.
- C. Choi, Y. Cho, A. Son, S. W. Shin, Y. J. Lee and H. C. Park, Mar. Drugs, 2020, 18, 500 CrossRef CAS PubMed.
- T. Iwagawa, M. Kaneko, H. Okamura, M. Nakatani and R. W. M. van Soest, J. Nat. Prod., 1998, 61, 1310–1312 CrossRef CAS PubMed.
- E. P. Stout, L. C. Yu and T. F. Molinski, Eur. J. Org. Chem., 2012, 27, 5131–5135 CrossRef PubMed.
- T. Kubota, T. Iwai, A. Takahashi-Nakaguchi, J. Fromont, T. Gonoi and J. Kobayashi, Tetrahedron, 2012, 68, 9738–9744 CrossRef CAS.
- L. L. Hong, J. B. Sun, F. Yang, M. Liu, J. Tang, F. Sun, W. H. Jiao, S. P. Wang, W. Zhang and H. W. Lin, RSC Adv., 2017, 7, 23970–23976 RSC.
- T. Li, B. Wang, N. J. de Voogd, X. L. Tang, Q. Wang, M. J. Chu, P. L. Li and G. Q. Li, Chin. Chem. Lett., 2016, 27, 1048–1051 CrossRef CAS.
- G. R. Pettit, Y. Tang, Q. Zhang, G. T. Bourne, C. A. Arm, J. E. Leet, J. C. Knight, R. K. Pettit, J. C. Chapuis, D. L. Doubek, F. J. Ward, C. Weber and J. N. A. Hooper, J. Nat. Prod., 2013, 76, 420–424 CrossRef CAS PubMed.
- S. Lee, N. Tanaka, J. Kobayashi and Y. Kashiwada, J. Nat. Med., 2018, 72, 364–368 CrossRef CAS PubMed.
- H. Nakamura, H. Wu, J. Kobayashi and Y. Ohizumi, Tetrahedron Lett., 1983, 24, 4105–4108 CrossRef CAS.
- H. Nakamura, H. Wu and J. Kobayashi, J. Org. Chem., 1985, 50, 2494–2497 CrossRef CAS.
- J. J. Morales and A. D. Rodriguez, J. Nat. Prod., 1992, 55, 389–394 CrossRef CAS PubMed.
- M. A. Medeiros, A. Lourenço, M. R. Tavares, M. J. M. Curtoa, S. S. Feioc and J. C. Roseiroc, Z. Naturforsch., C: J. Biosci., 2006, 61, 472–476 CrossRef CAS PubMed.
- N. Shoji, A. Umeyama, M. Teranaka and S. Arihara, J. Nat. Prod., 1996, 59, 448–450 CrossRef CAS.
- T. Natori, Y. Koezuka and T. Higa, Tetrahedron Lett., 1993, 34, 5591–5592 CrossRef CAS.
- T. Natori, M. Morita, K. Akimoto and Y. Koezuka, Tetrahedron, 1994, 50, 2771–2784 CrossRef CAS.
- M. Morita, K. Motoki, K. Akimoto, T. Natori, T. Sakai, E. Sawa, K. Yamaji, Y. Koezuka, E. Kobayashi and H. Fukushima, J. Med. Chem., 1995, 38, 2176–2187 CrossRef CAS PubMed.
- F. L. Schneiders, R. J. Scheper, B. M. E. von Blomberg, A. M. Woltman, H. L. A. Janssen, A. J. M. van den Eertwegh, H. M. W. Verheul, T. D. de Gruijl and H. J. van der Vliet, Clin. Immunol., 2011, 140, 130–141 CrossRef CAS PubMed.
- J. J. La Clair and A. D. Rodriguez, Bioorg. Med. Chem., 2011, 19, 6645–6653 CrossRef CAS PubMed.
- V. Costantino, E. Fattorusso and A. Mangoni, Liebigs Ann., 1995, 12, 2133–2136 CrossRef.
- V. Costantino, E. Fattorusso, C. Imperatore and A. Mangoni, J. Org. Chem., 2004, 69, 1174–1179 CrossRef CAS PubMed.
- V. Costantino, E. Fattorusso, C. Imperatore and A. Mangoni, J. Nat. Prod., 2006, 69, 73–78 CrossRef CAS PubMed.
- V. Costantino, E. Fattorusso and A. Mangoni, Liebigs Ann., 1995, 8, 1471–1475 CrossRef.
- F. Cafieri, E. Fattorusso, Y. Mahajnah and A. Mangoni, Liebigs Ann. Chem., 1994, 2, 1187–1189 CrossRef.
- F. Cafieri, E. Fattorusso, A. Mangoni and O. Taglialatela-scafati, Liebigs Ann., 1995, 8, 1477–1481 CrossRef.
- V. Costantino, E. Fattorusso, A. Mangoni, M. Di Rosa, A. Ianaro and P. Maffia, Tetrahedron, 1996, 52, 1573–1578 CrossRef CAS.
- G. R. Pettit, J. Xu, D. E. Gingrich, M. D. Williams, D. L. Doubek, J. C. Chapuis and J. M. Schmidt, Chem. Commun., 1999, 10, 915–916 RSC.
- G. D. Giacomo, A. Dini, B. Falco, A. Marino and D. Sica, Comp. Biochem. Physiol. B, 1983, 74, 499–501 CrossRef.
- C. Duque, G. Castillo, S. Buitrago, O. Osorno and S. Zea, Rev. Colomb. Quim., 1994, 23, 63–72 CAS.
- J. Hu, M. Kelly and M. T. Hamann, Steroids, 2002, 67, 743–747 CrossRef CAS PubMed.
- R. Buchecker, C. H. Eugster and C. Litchfield, Helv. Chim. Acta, 1977, 60, 2780–2788 CrossRef CAS.
- Y. Tanaka and T. Katayama, Bull. Jpn. Soc. Sci. Fish., 1982, 48, 531–533 CrossRef CAS.
- G. M. König and A. D. Wright, Heterocycles, 1993, 36, 1351–1358 CrossRef.
- G. M. König and A. D. Wright, Planta Med., 1998, 64, 88–89 CrossRef PubMed.
- F. Cafieri, E. Fattorusso and O. Taglialatela-Scafati, J. Nat. Prod., 1998, 61, 1171–1173 CrossRef CAS PubMed.
- F. Yang, R. H. Ji, J. Li, J. H. Gan and H. W. Lin, Nat. Prod. Commun., 2013, 8, 1713–1714 CAS.
- N. M. Carballeira and A. Emiliano, Lipids, 1993, 28, 763–766 CrossRef CAS PubMed.
- D. B. Abdjul, H. Yamazaki, S. Kanno, A. Tomizawa, H. Rotinsulu, D. S. Wewengkang, D. A. Sumilat, K. Ukai, M. M. Kapojos and M. Namikoshi, J. Nat. Med., 2017, 71, 531–536 CrossRef CAS PubMed.
- R. Ueoka, Y. Nakao, S. Kawatsu, J. Yaegashi, Y. Matsumoto, S. Matsunaga, K. Furihata, R. W. M. van Soest and N. Fusetani, J. Org. Chem., 2009, 74, 4203–4207 CrossRef CAS PubMed.
|
This journal is © The Royal Society of Chemistry 2022 |