DOI:
10.1039/D1RA08633B
(Review Article)
RSC Adv., 2022,
12, 6099-6113
Advances in pretreatment and analysis methods of aromatic hydrocarbons in soil†
Received
25th November 2021
, Accepted 27th January 2022
First published on 21st February 2022
Abstract
Benzene compounds that are prevalent in the soil as organic pollutants mainly include BTEX (benzene, toluene, ethylbenzene, and three xylene isomers) and PAHs (polycyclic aromatic hydrocarbons). These pose a severe threat to many aspects of human health. Therefore, the accurate measurement of BTEX and PAHs concentrations in the soil is of great importance. The samples for analysis of BTEX and PAHs need to be suitable for the various detection methods after pretreatment, which include Soxhlet extraction, ultrasonic extraction, solid-phase microextraction, supercritical extraction, and needle trap. The detection techniques mainly consist of gas chromatography (GC), mass spectrometry (MS), and online sensors, and provide comprehensive information on contaminants in the soil. Their performance is evaluated in terms of sensitivity, selectivity, and recovery. Recently, there has been rapid progress in the pretreatment and analysis methods for the quantitative and qualitative analyses of BTEX and PAHs. Therefore, it is necessary to produce a timely and in-depth review of the emerging pretreatment and analysis methods, which is unfortunately absent from the recent literature. In this work, state-of-art extraction techniques and analytical methods have been summarized for the determination of BTEX and PAHs in soil, with a particular focus on the potential and limitations of the respective methods for different aromatic hydrocarbons. Accordingly, the paper will describe the basic methodological knowledge, as well as the recent advancement of pretreatment and analysis methods for samples containing BTEX and PAHs.
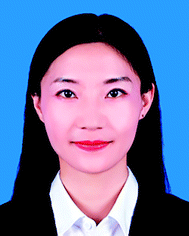 Na Song | Na Song received her B.Eng. degree in chemical engineering and technology from school of Chemistry & Materials Science, Huaibei Normal University in 2017. She is a graduate student at Northwest University, and is a master student supervised by Dr Yi-xiang Duan. She current research interests focus on online monitoring of organic pollution (BTEX and PAHs) in soil. The aim is to develop a method for online monitoring of organic pollutants in the soil, so as to achieve fast and simple analysis. |
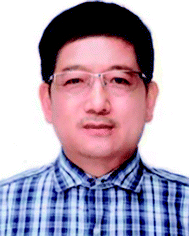 Yixiang Duan | Yixiang Duan is currently a National Special Professor, Northwest University, China. His current research interests include novel analytical instrumentation, noninvasive medical diagnostics, new ionization source for mass spectrum, molecule spectrometry, new biosensors design and development, and various applications, as well as the design and development of sensors and instrument for online detection of environmental pollutants on site, and various applications. He founded the Analytical Instrument Research Center, focusing on the research of new technologies, new methods and new instruments in analytical chemistry. |
1. Introduction
One of the main sources of soil pollution, benzene compounds, are a set of small molecules that contain one or more benzene rings. These substances are primarily generated during the incomplete combustion of organic materials such as coal, oil, petrol, and wood; they also are predominant in emissions from anthropogenic activities, particularly residential heating, petrochemicals, coal-chemicals, and coke and aluminum production. Nevertheless, they are also generated by natural processes, such as volcanic activities, forest fires, and seepage of petroleum or coal. Thus, PAHs and BTEX are commonly detected in the air, soil, and water. Benzene compounds accumulate through automobile exhaust and coal burning, and then slowly infiltrate into the soil environment.1 Among the many benzene compounds, PAHs and BTEX are the major persistent organic pollutants that accumulate in soil; their variety of structures results in a range of toxicities. The mass transfer of PAHs and BTEX in horizontal and vertical directions can be divided into three types of pollution: low (<200 μg kg−1), medium, and high pollution (>200 μg kg−1) areas.2 At present, there are insufficient data to show the extent of contamination of these compounds in soils in different regions of the world. Manmi et al.3 collected soil samples from the vicinity and surrounding area of petrol stations and oil refinery companies in the city of Sulaymaniyah in north-eastern Iraq for contamination assessment. The results showed very high-risk intensity in areas near the petrol and refinery stations, whereas in the area away from the station, the impact of PAHs and BTEX decreased gradually. Later, Wang et al.4 investigated the relationship between the level of urbanization and the accumulation of PAHs in soil. Their study demonstrated that the average level of PAHs in the soil from Nanjing (high urbanization) was higher than that from Dingshu (low urbanization). Thus, if the urbanization is higher and the human activities are more intense, there are more pollution sources to produce soil pollution of PAHs and BTEX.
The International Agency for Research on Cancer (IARC) classifies some PAHs and BTEX as known (Group 1), possible (Group 2A), probable (Group 2B), or uncertain (Group 3) carcinogenic to humans. Table S1† presents the physicochemical properties, toxicity, and pollution risk guidance values of common monocyclic aromatic hydrocarbons, as well as their derivatives. Moreover, 16 types of PAHs are listed as priority pollutants by the United States Environmental Protection Agency (USEPA). These substances are prevalent in the human environment through the volatilization of organic pollutants from soil and the exhaust emissions from transportation;5 they are highly toxic to human health, especially to the blood,6 nerves,7 and reproductive system.8 The assessment of exposure to BTEX and PAHs in soil is significant due to their harm and widespread presence in the environment. Therefore, the pretreatment and detection technology of organic pollutants in soil is the key to prevent and control soil pollution. To avoid adverse toxicity and reduce emissions, the accurate detection of the existence and relative content of BTEX and PAHs is of great importance. Notably, owing to their high lipophilicity, hydrophobicity and perdurability, PAHs and BTEX are easily adsorbed by organic matter in soil. Generally, PAHs are enriched in the topsoil layer, whereas BTEX is distributed more deeply.9 As such, the properties of these pollutants have a major impact on the choice of sample pretreatment method and analysis techniques. Although BTEX and PAHs in high-pollution areas can be easily detected, it is difficult to directly and sensitively analyze the organic pollutants in the low- and medium-pollution areas.10 As it is known that most pollution areas are slightly or moderately contaminated, therefore the low concentration and in situ online monitoring of BTEX and PAHs have emerged as challenges that require the development of pretreatment and detection techniques.
Classic extraction methods, such as Soxhlet extraction and liquid–solid extraction, have been applied to the extraction of BTEX and PAHs from soil.11,12 However, in recent years, researchers have gravitated to automated extraction techniques and solvent-free analysis methods, such as a combination of solid-phase microextraction (SPME) and gas chromatography–mass spectrometry (GC–MS).13,14 Supercritical fluid extraction (SFE) and ultrasonic extraction are also emerging as techniques applied for the extraction of volatile organic pollutants in soil owing to the high-extraction efficiency and short processing time.15,16 In addition to pretreatment methods, the technologies for the detection of BTEX and PAHs in the soil include GC,17 GC–MS,18 MS,19 liquid chromatography–mass spectrometry (LC–MS),20 and online sensors.19,21,22 In GC, a flame ionization detector (FID) is commonly used for the determination of BTEX and PAHs. However, with the progress in analytical technology, the qualitative and quantitative abilities of GC–MS methods have greatly improved, in addition to enhanced sensitivity. Therefore, GC–MS has gradually emerged as a common method for the detection of BTEX and PAHs in soil. LC–MS methods can provide more useful information for the analysis of PAHs because of their high sensitivity, selectivity, and capacity for identification. In addition, some chemical and biological sensors have been applied for the online monitoring of pollutants in soil.23,24 When integrated with suitable pretreatment methods, the detection technologies are promising analytical tools to monitor the pollution of BTEX and PAHs in soil.
Over the past few years, extraction methods and detection techniques for BTEX and PAHs in the environment have been extensively reviewed in the literature. Most of them are periodic summaries of this field, such as the analysis of PAHs in sediment,25 analytical research methods of PAHs,26,27 pollution status and research progress of PAHs in water,28 or sampling and pretreatment techniques of PAHs in air, water and soil,29,30 or marine environmental behavior and metabolites of PAHs.31 On the other hand, only a few studies discussed and summarized the pretreatment and detection techniques of BTEX and PAHs in soil.32 In this review, works published within the past 5 years on the pretreatment and detection technologies for BTEX and PAHs in soil are systematically summarized. The review discusses the principles, main functions, process plans, advantages, and disadvantages of various sample treatments. The methods are also compared to show the limitations and potentials of each method. Secondly, it discusses the limitations of detection technology and related analysis methods used to analyze BTEX and PAHs in soil, and proposes some rapid detection technologies and analytical methods for BTEX and PAHs in soil. The common procedures for analysis of BTEX and PAHs in soil samples are tabulated in Fig. 1. This work aims to help readers fully understand the pretreatment technologies, detection technologies, and analysis methods for BTEX and PAHs in the soil matrix, and will guide readers in the correct choice and development of new research methods to improve sensitivity, specificity, stability, and productivity.
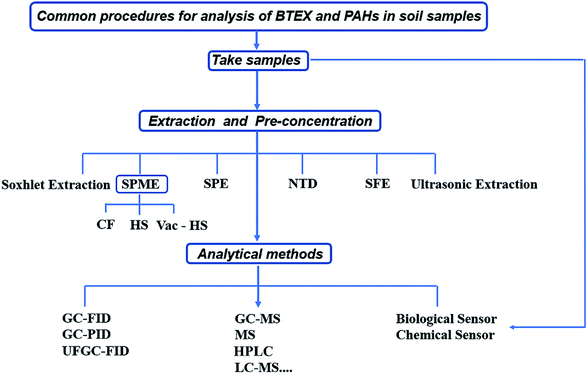 |
| Fig. 1 Common procedures for analysis of BTEX and PAHs in soil samples. | |
2. Pretreatment methods for BTEX and PAHs in soil samples
The measurement of BTEX and PAHs in soil samples is influenced by soil matrix, concentrations and volatility of analytes. Therefore, appropriate pretreatment methods are the prerequisite and basis to determine the specific objects in the environmental matrix accurately. The advantages and disadvantages of common pre-treatment methods for aromatic compounds in soil are summarized in Table 1.
Table 1 Advantages and disadvantages of different extraction techniques for the pre-concentration of aromatic hydrocarbon compounds in soil
Order |
Technique |
Advantages |
Disadvantages |
1 |
Needle-trap device (NTD) |
Suited for the extraction and preconcentration of (VOCs), more reliable, lower-cost, higher capacity and robust, the exhaustive extractions by appropriate selection of the experimental variables |
Cumbersome process, low reproducibility |
2 |
Soxhlet extraction |
High extraction effectiveness, better selectivity, simple equipment |
Consume time, cumbersome operation, large volumes of solvent |
3 |
Ultrasonic extraction |
Short extraction time, wide adaptability, more economical |
Low recoveries particularly for lower molecular weight PAHs |
4 |
Mechanical agitation |
Simple, low-cost, smaller volumes of extraction solvent, minimal glassware |
Lower extraction efficiency, lower selectivity, not wide adaptability, unsatisfactory quantitative results |
5 |
Supercritical fluid extraction (SFE) |
Cleaner extracts, better selectivity |
More difficult to optimize |
6 |
Solid phase extraction (SPE) |
Complete the sample enrichment and purification at the same time, greatly improving the detection sensitivity, faster than liquid–liquid extraction, more solvent saving, automatic batch processing, better reproducibility |
Needs a lot of organic solvents, which leads to high cost and environmental pollution, it is difficult to extract high water soluble substances from water, weak purification effect |
6 |
Solid-phase microextraction (SPME) |
No need for extraction solvent, suitable for the analysis of volatile and non-volatile substance, convenient to carry, lower cost |
Limited types of coatings, lower selectivity |
7 |
Cold-fiber solid-phase microextraction (CF-SPME) |
Improve the extraction of compounds with different volatilities, the sample can be heated to a high temperature, the coating can be simultaneously cooled, no need for sample pretreatment |
Unable to monitor in real time, its construction is difficult, its use is troublesome |
8 |
Vacuum-assisted headspace solid phase microextraction (Vac-HSSPME) |
Good sensitivities short sampling times, high extraction efficiencies |
The thermogreen septumwas replaced daily |
2.1 Soxhlet extraction
Soxhlet extraction was reported for the first time in 1879, representing a milestone moment in the history of solvent extraction methods.33 Soxhlet extraction is commonly used for semi-volatile compounds such as PAHs and PCBs.34,35 Soxhlet extraction is recommended by the USEPA for extraction of PAHs from soil samples owing to its high extraction efficiency and small standard deviation.36–39 However, it has drawbacks, such as time and solvent consumption (10 g of soil sample requires 150 mL of solvent).40–42 Tackling the shortcomings of traditional Soxhlet extraction has highlighted the starting areas for further development of Soxhlet apparatus, particularly automated Soxhlet extraction and microwave-assisted Soxhlet extraction.43 Improved Soxhlet pretreatment methods provide substantial savings in time and extractants. In this method, the sample is extracted with pure solvent during each cycle via the principle of solvent reflux and siphon. Notably, the choice of extraction solvent depends on whether the sample is dry or not, and whether the surface is wettable. If it is not dry and there are traces of water on the sample surface, then extraction with an apolar solvent is typically unsuccessful owing to miscibility and wettability issues. In this case, a water-miscible solvent or solvent mixture (such as acetone/hexane) must be used.44–46 Moreover, the addition of a neutral salt, such as sodium sulfate, that assists in drying the water in the extraction solvent is needed. Szolar et al.47 used 1
:
1 (v/v) n-hexane/acetone as the extraction solvent, and the addition of sodium sulfate led to a significant increase in the extraction efficiency of all PAHs in industrially contaminated soil.
2.2 Ultrasonic extraction
In the early 1950s, ultrasonic waves were used to extract picrin from peanut oil and grease from fish tissues. Ultrasonic extraction is currently a promising pretreatment technology for the detection and analysis of PAHs in soil.38,46,48,49 Compared with Soxhlet extraction, ultrasonic methods are more economical, environmentally friendly, effective, and easier to operate.50 The sonication procedure shows that individual PAHs can be extracted at different rates according to the number of fused rings in the molecules,51 whereas a loss of volatile PAH components is observed after 1–4 h in Soxhlet extraction. Besides, ultrasonic extraction is suitable for the extraction of non-heat-resistant target composition, which avoids the problems of limited material amount and longer operation time for the Soxhlet method.
In ultrasonic extraction, ultrasonic-assisted solvent is used to extract PAHs. The sample mixture is disintegrated by sound energy transmitted through a liquid phase. Common solvents are acetone, cyclohexane, 2-propanol, methanol, dichloromethane, acetonitrile, and tetrahydrofuran.52 The sample in the solvent can be placed directly into an ultrasonic bath or an ultrasonic sensor can be directly immersed in the sample/solvent mixture. Ultrasonic waves can induce strong cavitation and stirring effects, which facilitates the target analytes to be assimilated into the solvent. The following two features should be noted when ultrasound extraction is used: (1) the extraction efficiency is closely related to ultrasonic power and duration; (2) under the action of ultrasound, samples containing volatile degradation compounds are not suitable for ultrasonic extraction. In other words, to achieve the high efficiency of ultrasonic separation and extraction, the relationship between ultrasound and the subjects should be figured out in advance. Higher amplitudes of vibrations lead to an increase in the analyte extraction from solid samples.53 Moreover, increasing the sonication time at the beginning of the process will result in the desorption of the analyte from the solid sample, whereas excess sonication time will reduce the extraction efficiency. In Heydari's study, ultrasound combined with salt-assisted liquid–liquid extractors for the effective extraction of PAHs from soil samples. The factors influencing the extraction efficiency of analytes were investigated and optimized, including the extraction solvent (tetrahydrofuran) and its volume, ultrasonic time, ultrasonic amplitude, and pulse.54
With regard to organic substances, ultrasonic extraction technology has displayed advantages. The extraction rate of BTEX from soil was increased and the volume of organic solvent was reduced by using a combined strategy of ultrasonic treatment with further separation techniques (such as solid-phase extraction, centrifugation, and filtration).37 Shahram et al. discussed the possibility of coupling ultrasound with other analytical techniques.55 In addition, ionic liquids or surfactants were also used as green solvents in ultrasound methods to improve the scope of its application.56
2.3 Supercritical fluid extraction (SFE)
Supercritical fluid extraction (SFE) has increased in use for soils and has been widely accepted as a replacement for classical extraction methods, especially for the extraction of volatile organic compounds (VOCs) and persistent organic pollutants (POPs).57,58 Compared with Soxhlet extraction, SFE has the advantages of shorter extraction times and smaller amounts of extraction solvent. SFE is based on the principle that the solubility of a supercritical fluid is closely related to its density, which varies with a change in the pressure or temperature. Selectivity is another advantage of SFE. Changing the pressure or temperature affects the solubilizing potency of the fluid. This makes it possible to extract complex compounds.59 In the majority of analytical studies using SFE, PAH extraction efficiencies were generally observed to increase with an increase in temperatures.60–62 Other researchers applied a variety of operational parameters, such as fluid pressure, fluid temperature, extraction time, cosolvent type and amount, and modifiers, to optimize the recovery of PAHs.63 When the temperature and pressure of supercritical fluids are controlled, researchers can use the penetrating characteristics of gases and the solvating properties of the liquid to obtain satisfactory experimental results. Carbon dioxide (31 °C, 74 bar) is widely employed in SFE as an environmentally friendly solvent because of its low activity, low price, high purity, and easy preparation.64 Moreover, a trace amount of polar solvent in the supercritical fluid can further expand the application of this technology.65 When SFE is used, the extract is usually recovered in a liquid solvent or a solid trap. Direct real-time monitoring of the extracts is conducted by coupling the supercritical fluid extractor with the analytical detector to improve recovery rate and reduce operation error.66 SFE devices have already been applied owing to their online connections to different analytical apparatus.67,68
However, as CO2 is the only choice as a green extractant in SFE, it is only suitable for the extraction of non-polar substances within a narrow range. If we can find a substance that can be used as an extractant instead of CO2, this problem can be solved. Water is also used as the extracting fluid. Subcritical water extraction (SWE), also known as pressurized hot-water extraction, is used instead. SWE is more selective for more polar analytes, therefore providing a higher extraction efficiency for PAHs.69 Notably, the properties of sub- and supercritical fluids are quite different. In the subcritical state, the temperature of a given substance is above its boiling point but below the critical temperature and the pressure is below the critical pressure.
2.4 Solid-phase extraction (SPE)
As a sample pretreatment method, solid-phase extraction (SPE) has rapidly evolved in recent years.70 Currently, SPE is an indispensable pretreatment method for the purification of solid sample extracts. It is mainly used for the separation, purification, and concentration of the samples. SPE is a type of physical extraction process that is based on the separation principle of liquid chromatography under selective adsorption and elution.71 This method has the advantages of low sample matrix interference, high separation efficiency of analyte from interfering components, simple operation procedure, fast pretreatment process, and the range of sorbents available. The sorbents include reversed-phase hydrophobic–hydrophilic balanced (HLB) polymeric sorbents, molecularly imprinted sorbents, alkyl-modified silica (C-18 non-polar phase), and ionic liquid-coated Fe3O4 magnetic nanoparticles, among others.72,73 The HLB-based sorbents are the preferred solid-phase materials owing to the improved recoveries and good extraction efficiency for pollutants.74 Notably, SPE is not a direct extraction technique for PAHs from soil; it may only be used with soil extracts. As such, it may be used as a sample clean-up technique.75 In 2016, Zhang et al.76 used an SPE method to purify soil samples. The samples were first extracted by a mixed solution of n-hexane and acetone (4
:
1, v/v), then purified by a SPE column (6 mL silica gel column). A mixture of n-hexane and dichloromethane (9
:
1, v/v) was used as the eluent. This method can be used for a highly accurate quantitative analysis of PAHs in soil samples.
2.5 Solid-phase microextraction (SPME)
Solid-phase microextraction (SPME) has also been employed as a new preparation method for the extraction of PAHs and BTEX from soil. SPME is a reliable and effective alternative method to SPE.77 Specifically, SPME is easy to operate, convenient to carry, and has a lower cost and short operating time. It overcomes the disadvantages of low recovery and blockage in adsorbent channels. The method, which has good reproducibility, has been applied to the analysis of volatile and non-volatile substances. In addition, the different extraction modes are available depending on the types of analytes: low-volatile compounds and liquids are extracted in direct mode, whereas high-volatile compounds are extracted in headspace mode.78 It is worth noting that the direct mode is used only for the extraction of analytes from a suspension of solid samples. During the extraction of BTEX and PAHs, the headspace extraction method is favored because it can improve the extraction rate of different volatile compounds.
The core part of SPME is fiber coating.79 Various types of fiber coatings have been used to fabricate SPME, such as carbon fibers (CFs), metal–organic frameworks (MOFs), polydimethylsiloxane (PDMS) and hollow carbon nanobubbles.80–82 Among them, PDMS coatings83 have been widely used in the extraction of BTEX and PAHs in various environments. When the fiber is placed in the samples, the target analyte can be adsorbed and concentrated on the coating of the SPME fine needle; then, they are separated from the fiber by thermal desorption or solvent desorption. Xu et al.84 developed a novel SPME fiber based on hollow carbon nanobubbles for the analysis of BTEX and PAHs. This architecture enhanced the enrichment capacity of the target analytes and improved extraction efficiency. In addition, the lifespan of such fiber was estimated to be over 100 times required for successful analysis of environmental samples.
To improve the extraction abilities of SPME, a cold fiber solid-phase microextraction (CF-SPME) technique was developed to extract PAHs from soil samples.78 In CF-SPME, the samples are heated to high temperatures, while the coating can be cooled, which causes a significant improvement in the analyte distribution coefficient between the coating and the sample. Ghiasvand et al.85 applied successfully CF-HS-SPME-GC-FID to PAHs, and achieved reproducible, precise, and high-throughput extraction, monitoring, and quantification of PAHs as a result of the temperature gap between the cold-fiber (CF) coating and hot headspace. The temperature gap can improve the efficiency of the release of analytes from the matrix and facilitate the mass transfer into the headspace. However, owing to the complex structure and complicated operation of the CF-SPME device, research into and application of CF-SPME are greatly restricted. To address the challenge of the complicated construction of CF-SPME, Xu et al.86 designed a facile device for cooling-assisted solid-phase microextraction (CA-SPME). Additionally, Ghiasvand et al. proposed a cooling/heating-assisted headspace solid-phase microextraction device (CHA-HS-SPME) coupled with gas chromatography-flame ionization detector (GC-FID), which was successfully applied for the extraction and determination of PAHs in soil.87 The proposed CHA-HS-SPME device is shown in Fig. 2. Good consistency was observed between the results obtained by the proposed method and those achieved by a validated method.
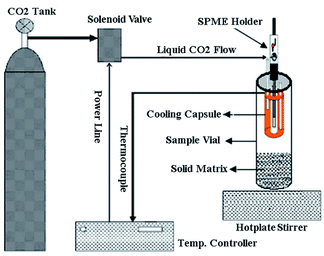 |
| Fig. 2 The proposed a cooling/heating-assisted headspace solid-phase microextraction (CHA-HS-SPME) device (adapted from ref. 87). This figure has been adapted from reference “Cooling/heating-assisted headspace solid-phase microextraction of polycyclic aromatic hydrocarbons from contaminated soils” with permission from ELSEVIER, copyright 2015. | |
The vacuum-assisted headspace solid-phase microextraction (Vac-HS-SPME) method is another new pretreatment method.88 A schematic diagram of Vac-HS-SPME is shown in Fig. 3. Psillakis's group reported the use of Vac-HS-SPME for the extraction of PAHs from solid matrices, where sand was chosen as a simple matrix for the extraction of low-molecular-weight PAHs. The effect of different experimental parameters on Vac-HS-SPME was investigated. Compared with the routine headspace solid-phase microextraction (HS-SPME), Vac-HS-SPME produced higher extraction efficiency and better sensitivity with a shorter time and lower temperature (such as room temperature).89,90
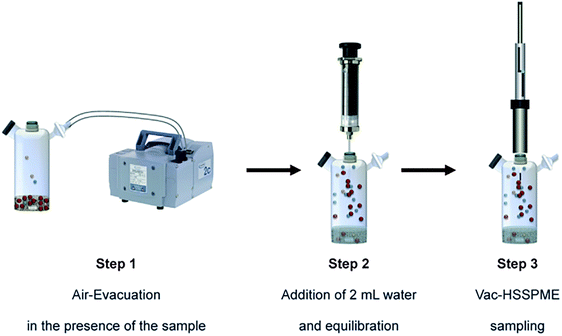 |
| Fig. 3 The schematic diagram of vacuum-assisted headspace solid phase microextraction (adapted from ref. 88). This figure has been reproduced from reference “Vacuum-assisted headspace solid phase microextraction of polycyclic aromatic hydrocarbons in solid samples” with permission from ELSEVIER, copyright 2015. | |
2.6 Needle-trap device (NTD)
A new dynamic needle-trap device (NTD) was recently developed to actively extract and enrich organic pollutants of BTEX and PAHs.17,91 NTD is an active sampling method owing to its non-equilibrium adsorption process and is a promising extraction method for PAHs in soil, owing to the associated advantages of rapid, solvent-free, and sample-lossless analysis.92 The NTD technique consists of sorbents packed inside a stainless-steel needle, and a gas-tight syringe or a pump through which volatile analytes are drawn into the needle and introduced actively to the trap.93 Target analytes can be adsorbed in the needle and removed at the inlet of the analytical instrument for qualitative and quantitative fractionation. A schematic representation of NTD device is shown in Fig. 4. To achieve quantitative extraction of the analytes in the shortest time, various parameters that potentially affect the extraction process should be optimized, such as extraction temperature, time, and desorption conditions. In general, higher temperatures can enhance the desorption process with less of a carryover effect, but attention should be paid to the thermal stability of the adsorbent and the target analyte. In contrast, longer times can lead to high response values for analytes, but may reduce the lifetime of the sorbent. It is important to note that the extraction conditions should be chosen based on the characteristics of the sorbent and the target analyte. According to Poormohammadi's works,94 an NTD packed with Carbotrap B provides a highly sensitive procedure for the sampling and analysis of BTEX in the concentration range of 0.03–25 ng mL−1.
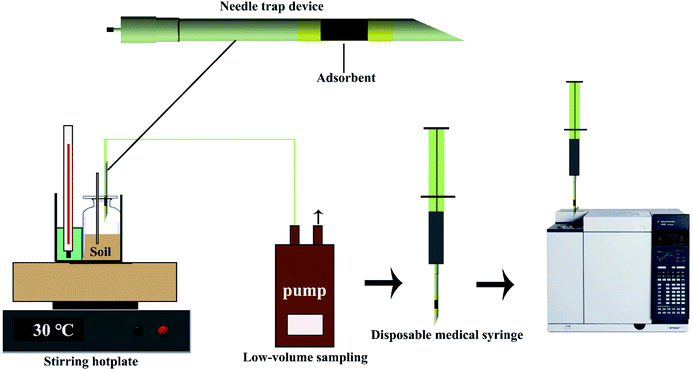 |
| Fig. 4 The schematic representation of NTD device. This figure has been adapted from reference “A needle trap device packed with MIL-100(Fe) metal organic frameworks for efficient headspace sampling and analysis of urinary BTEXs” with permission from John Wiley and Sons, copyright 2020. | |
2.7 Comparison of different pretreatment methods for BTEX and PAHs in soil
Soxhlet extraction, as one of the classic conventional extraction techniques, has been successfully applied for the extraction of the 16 types of PAH in soil listed by the EPA.11 Despite the relatively high extraction duration compared to other newly developed techniques such as ultrasonic extraction and SFE, its simple setup and unlimited extraction capacity present an ideal extraction method for non-targeted soil analysis. Soxhlet extraction is mainly recommended for analyses of wet soil samples and sample quantities of less than three grams.95 Semi-volatile compounds (especially PAHs with five or six aromatic rings) are usually extracted by Soxhlet or sonication. However, SFE has been shown to provide a higher yield in a shorter time, which translates into a better economic investment. Therefore, SFE has been widely accepted as an alternative to classical extraction methods, especially for low solubility compounds. Generally, the SPE method is used to purify soil extract. NTD is most appropriate for the extraction of volatile organic compounds (VOCs) and is mainly applied in the headspace mode for the trapping of volatile and semi-volatile analytes. At present, there are two recommended methods for extraction of PAHs and BTEX in soil: the headspace technique and SPME. The automation of the extraction method is crucial to achieve the required repeatability and accuracy for the determination of PAHs and BTEX in soil.96 The connection of both techniques proved to be adequate for the analysis of PAHs and BTEX with good precision, linearity, and no noticeable impact of water content in soil samples. Essentially, the headspace is applied for soil samples containing low concentrations of pollutants, whereas the solvent extraction method is used for more contaminated soil samples. Nonetheless, further innovations are still needed to improve the efficiency of the extraction methods and to address the problems associated with these analysis techniques for BTEX and PAHs in soil. Therefore, future research studies should consider the development of combined extraction techniques to increase their merits, increasing the “green” nature of the sample pretreatment stage, shortening the analysis time, using greener media (e.g., supercritical liquids, ionic liquids, and super-heated water) instead of toxic organic solvents, using new sorbents such as nanoparticles and metal organic frameworks in solid phase-based extraction methods, facilitating automation, and increasing the selectivity of the pretreatment methods.
3. Analysis techniques for BTEX and PAHs in soil
Due to the difficulty of analyzing BTEX and PAHs in complex matrices, it is necessary to develop efficient, inexpensive, rapid and environmentally friendly assay methods. At present, since the rapid development of analytical methods, the detection of BTEX and PAHs is readily realized. The detection technologies used for BTEX and PAHs in the soil are mainly gas chromatography (GC), gas chromatography-mass spectrometry (GC–MS), liquid chromatography–mass spectrometry (LC–MS), sensors and other methods. The detection methods for BTEX and PAHs in soil are summarized in Table 2.
Table 2 Sample pretreatment methods and the detection technique for BTEX and PAHs in soila
Order |
Analytes |
Method |
Analytical instrument |
Matrix |
LOD |
LDR |
RSD (%) |
Recovery (%) |
Ref. |
NTD: needle trap device; CF-SPME: cold fiber-SPME; PC-HS-SPME: pressure controlled headspace-SPME; HS: head space; VA-HS-SPME: vacuum-assisted headspace solid phase microextraction; CA-HS-HF-LPME: cooling-assisted headspace fiber liquid phase microextraction; CA-SPME: cooling assisted solid phase microextraction; MSPD: matric solid phase dispersion; QuEChERS: quick, easy, cheap, effective, rugged and safe; PTV: programmable temperature vaporizer; Vac-HSSPME: vacuum-assisted headspace solid phase microextraction; RTILs-HS: room temperature ionic liquid co-solvents; DSPE: dispersive solid phase extraction; DLLE: dispersive liquid–liquid extraction; HS-SPME: head space-SPME. |
(A) GC |
1 |
PAHs |
NTD |
GC-FID |
Soil(sand) samples |
0.001–0.02 ng g−1 |
0.01–2000 ng g−1 |
8.3–13.2 |
— |
17 |
BTEX |
0.02–0.1 ng g−1 |
0.1–2000 ng g−1 |
7.3–10.3 |
— |
2 |
BTEX |
Sonication methods |
UFGC-FID |
Soil samples |
— |
— |
<1 |
70–130 |
96 |
3 |
PAHs |
CF-SPME |
GC-FID |
Sand or soil samples |
200–2000 ng g−1 |
— |
4–19 |
— |
78 |
4 |
BTEX |
PC-HS-SPME |
GC-FID |
Soil samples |
0.001–0.08 ng g−1 |
0.1–20 000 ng g−1 |
5.7–12.3 |
— |
104 |
5 |
BTEX |
HS-GC-PID |
GC-PID |
Soil samples |
0.1–0.8 μg kg−1 |
— |
5.3–7.8 |
87.2–105.1 |
101 |
6 |
PAHs |
CA-HS-HF-LPME |
GC-FID |
Soil and plant samples |
0.01–0.1 ng g−1 |
1–10 000 ng g−1 |
4.7–10.1 |
— |
105 |
7 |
PAHs |
CA-SPME/MSPD |
GC-FID |
Soil samples |
4.2–8.5 ng g−1 |
— |
8.1–13.4 |
— |
86 |
![[thin space (1/6-em)]](https://www.rsc.org/images/entities/char_2009.gif) |
(B) LC |
8 |
PAHs |
MHLLE |
HPLC-FL |
Sediment |
— |
0.003–0.04 ng g−1 |
— |
81–92 |
106 |
9 |
PAHs |
Ultrasonic probe |
HPLC |
Soil samples |
0.07–0.3 ng g−1 |
0.7–200 ng g−1 |
<7.5 |
80–100 |
38 |
10 |
BPCA |
Internal standards |
LC–MS |
Soil samples |
— |
— |
<5 |
— |
20 |
Deuterated phthalic acid |
![[thin space (1/6-em)]](https://www.rsc.org/images/entities/char_2009.gif) |
(C) GC–MS |
11 |
BTEX |
QuEChERS |
GC–MS |
Soil samples |
0.3–15 ng g−1 |
— |
0.7–2.8 |
65–76 |
29 |
PTV |
12 |
PAHs |
Vac-HSSPME |
GC–MS |
Sand samples |
0.003–0.233 ng g−1 |
1–400 ng g−1 |
4.3–10 |
— |
107 |
Soil samples |
0.003–0.795 ng g−1 |
13 |
BTEX |
RTILs-HS |
GC–MS |
Sand samples |
Sub-to mid pg g−1 |
— |
2–18 |
15–103 |
108 |
Clay samples |
Loam samples |
14 |
BTEX |
DSPE |
GC–MS |
Soil samples |
0.12–0.75 ng g−1 |
4–100 ng g−1 |
0.25–2.3 |
— |
109 |
DLLE |
15 |
Naphthalene |
HS-SPME |
GC–MS |
Soil samples |
0.001 ng g−1 |
0.01–0.1 ng g−1 |
— |
105–119 |
110 |
Standard addition method |
3.1 Gas chromatography (GC)
GC is a valuable method for the analysis of PAHs and BTEX in soil. It is of a particular interest for this family of compounds because of its ability to separate structural isomers according to their difference in boiling point. In GC, a flame ionization detector (FID) has the merits of high sensitivity, precision, and reproducibility for most hydrocarbon compounds.97–99 Remarkably, in complex matrices such as soil or atmospheric PM, to prevent all organic molecules from giving a response (such as aliphatic compounds and lipids), a very intensive clean-up is required before the sample is analyzed. Moreover, some researchers have attempted to perform BTEX and PAHs determinations using a barrier ionization discharge detector (BID) and a photoionization detector (PID). The PID is suitable for volatile and semi-volatile organic compounds with ionization energies lower than the energy of emitted photons (from 8 to 12 eV).100 A method based on headspace sampling, and integrated with a portable GC with PID was proposed for the rapid determination of BTEX in soil by Zhou et al.101 This method was suitable for the rapid determination of BTEX in soil on site.102 Pascale et al.103 established a simple, low-cost, and accurate new method to quantify BTEX based on static headspace extraction-GC-BID. However, owing to its high sensitivity, FID has become a common detector for the determination of BTEX and PAHs by GC.
Ultra-fast gas chromatography (UFGC) has emerged with the development of the direct resistively heated column.111 In 2001, Robbat et al. directly extracted PAHs from soil using a heat-extracting cone penetration instrument (TECP) for UFGC–MS detection.112 Later, an ultra-fast chromatography-flame ionization detector (UFGC-FID) combined with the sonication methods was applied to detect BTEX in the soil, where the issues of the longer analysis time of volatile compounds were addressed.113 According to Nespeca's study,113 the time of the UFGC analyses was eight times shorter than that of conventional GC. Combining ultrasonic extraction methods with UFGC-FID makes the detection process faster and cheaper. However, this method still requires organic solvents, which causes secondary pollution to the environment.
In recent years, solvent-free analysis methods in the principles of green chemistry have been advocated by researchers.114 A new pressure-controlled headspace solid-phase microextraction (PC-HS-SPME) device combined with GC-FID enabled the direct analysis of benzene, toluene, ethylbenzene, and m-xylene (BTEX) in soil, in which the extraction is completed without complex sample preparation steps104 because the gradient pressure allows the analyte to be effectively released from the sample matrix to vacuum. This simple, low cost, and efficient method is capable of the efficient extraction of the analyte with high concentration. In another solid-phase microextraction study, temperature-controlled HS-SPME and PC-HS-SPME were intercoupled to extract PAHs from the soil.115 Xu et al. proposed a new study on a solvent-free analysis for PAHs in soil.86 In his study of a facile cooling-assisted solid-phase microextraction (CA-SPME) device for solvent-free sampling of PAHs from soil based on matrix solid-phase dispersion (MSPD) techniques, the MSPD promoted the release of PAHs, which lead to the efficient extraction of PAHs by the CA-SPME device. These examples demonstrate that satisfactory results can be achieved by the solvation-free method for PAHs in soil.
3.2 Gas chromatography–mass spectrometry (GC–MS)
The three key factors affecting the determination results of BTEX and PAHs in soil are complexity, heterogeneity, and the interaction between analytes and soil components.116 GC-MS in the determination of organic compounds is highly appreciated by analysts for its better sensitivity and selectivity. Besides, GC-MS can be used for the determination of non-fluorescent PAHs, such as such as acenaphthylene (Acy) and alkylated PAHs.
At present, a variety of pretreatment methods, such as static headspace,117 dynamic headspace, SPME, dispersive solid-phase and dispersive liquid–liquid extraction,109 have been used to establish GCMS-based analysis methods for quantification of BTEX and PAHs in soil. The addition of water as a modifier or preparing a mud mixture can increase the extraction amount of analyte to solve the problem of low extraction of PAHs.107 In GC–MS, internal standards or surrogates were also used to improve the accuracy and precision of the analytical method. At a specific stage of the analytical procedure, internal standards can be used to track and compensate for analyte losses and make the results more accurate, as well as further improve the recovery rate of the target analyte.118 Further, the determination of BTEX in soil using room-temperature ionic liquid cosolvents (RTILs) can reduce the matrix dependency of soil and analysis time, as well as increase the precision and accuracy for the quantification of BTEX in various soil matrices. This method can improve the low extraction efficiency of high-molecular-weight volatile organic compounds in soil systems.108
The growing demand in trace analysis for lower detection limits has led to the development of innovative ways of introducing larger sample volumes into GC and GC–MS systems. According to reports, a programmable temperature vaporizer (PTV) enables the injection of larger volumes when the expected levels are very low, and protects thermolabile compounds by progressively heating and vaporizing them.119,120 Compared with traditional GC method, the recovery of GC–MS with PTV and an automatic sampler was significantly improved; this was due to the reduced analyte loss during the sample preparation step.121
3.3 Liquid chromatography–mass spectrometry (LC/LC–MS)
Liquid chromatography coupled with mass spectrometry (LC–MS) has emerged as an invaluable technique for tracing pollutants in the environment. MS detection has been widely used for soil because of its good selectivity and high sensitivity. Electrospray ionization (ESI), atmospheric-pressure chemical ionization source (APCI), and atmospheric pressure photoionization (APPI) have generally used to ionize PAHs at the LC–MS interface.122,123 These ionization sources can effectively ionize high-molecular-weight PAHs.124 One study showed that APPI offers at least 3–4 orders of dynamic linear range for PAH analysis under the test conditions.125 In addition, internal standards are widely used in LC–MS for the quantitative analysis of PAHs and BTEX, which can compensate for the loss or change in sample preparation. Structural analogs and stable isotope labeling (SIL) are recognized as ideal internal standards because they possess similar physical and chemical properties to the analytes.20,126 For PAHs analysis, a variety of isotopes have been reported, including deuterated phthalic acid,20 naphthalene-d8,127 benzo[a]pyrene-d12,128 and perylene-d12.129 However, some researchers believe that the prevention and treatment of environmental pollution are hampered by a lack of adequate field-testing procedures rather than sending samples to centralized analytical facilities. Thus, Stelios et al.130 reported a hand-portable system based on high-performance liquid chromatography incorporating a wide-spectrum absorption detector. The system is capable of fingerprinting PAHs based on their characteristic spectral absorption profiles. Its detector system is robust enough to detect and classify co-eluting and hidden peaks.
In recent years, the emergence of high-performance liquid chromatography (HPLC) has provided a rapid and sensitive method to analyze BTEX and PAHs in soil. In HPLC, the columns used for separation were generally octadecylsilane (C18: 250 × 4.6 mm, 5 μm), and the detectors used for detection were ultraviolet (UV) or fluorescence detectors.131,132 There are also some reversed phases based on cross-linked C18 that have shown a particular specificity for PAHs separation.133 The characteristic spectra of PAHs can be quantified and identified using a fluorescence detector and UV-DAD. A gradient elution procedure is usually required for more efficient separation. The mobile phases for separating PAHs are usually acetonitrile (ACN) or methanol (MeOH) and water.134 Unlike GC, HPLC is not limited by sample volatility and thermal stability. However, the resolution of LC methods is not as good as that of GC methods for isomer detection.135
3.4 Biological and chemical sensor
Biological and chemical sensors provide rapid response for the online monitoring of contamination in soil. Online monitoring methods with biological and chemical sensors are summarized in Table 3 for in situ and real-time detection of the changes in the concentration of pollutants in soil.136 The sensor method possesses the advantages of speed, portability, and low cost. As early as 2003, a whole-cell microbial sensor on a silicon chip was constructed to perform efficient and low-cost online monitoring of environmental pollutants outside the laboratory.137,138 In 2016, Hernandez et al. constructed a sensor based on the bioreporter system to monitor BTEX in the soil environment. The bioreporter system was based on the two-component regulatory system TodS-TodT of P. putida DOT-T1E, and the Ptodx promoter fused to the green fluorescent protein (GFP) as the reporter protein.21 However, this method has certain limitations, such as weak mechanical strength, making it suitable only for low-concentration pollutants. Therefore, Bae and co-workers23 proposed an optical detection biosensor module for evaluating toluene-contaminated soil. The sensor used bioluminescent bacterial bioreporters encapsulated in poly-dopamine (PD)-coated alginate microbeads to enhance mechanical strength and stability. Therefore, the biosensor opens up new avenues to low cost, rapid determination, and higher sensitivity of analytical methods. Furthermore, the successful implementation of biosensors may help to monitor pollution conditions for prevention and regulation.
Table 3 Advantages and disadvantages of different pretreatment methods and detection techniques for aromatic compounds in soil samplesa
Order |
Method |
Analytical instrument |
Advantages |
Disadvantages |
NTD: needle trap device; BBD: box-Behnken design; CF-SPME: cold fiber-SPME; CA-HS-HF-LPME: cooling-assisted headspace fiber liquid phase microextraction; MHS-SPME: multiple headspace-SPME; HS: head space; Vac-HS-SPME: vacuum-assisted headspace solid phase microextraction; QuEChERS: quick, easy, cheap, effective, rugged and safe; RTILs: room temperature ionic liquid co-solvents; DSPE: dispersive solid phase extraction; DLLE: dispersive liquid–liquid extraction; HS-SPME: head space-SPME; HMOFs: microporous heterometal–organic framework. |
(A) GC |
1 |
NTD BBD |
GC-FID |
No sample pretreatment, no obvious matrix effect, wide range of LDR, lower LOD, smaller RSD |
Long analysis time, it can't be monitored online, taditional NTD methods have limitations |
2 |
Sonication methods |
UFGC-FID |
It avoids the disadvantage of long analysis time, good linearity and repeatability, fast detection process, lower cost, environmentally friendly |
Less research on aromatic hydrocarbons in soil |
3 |
CF-SPME |
GC-FID |
Improved extraction speed and efficiency, easy to automate |
Unable to monitor in real time, the use of organic solvents is not environmentally friendly |
4 |
Static headspace |
GC-BID |
Simple and accurate, low cost; high sensitivity |
|
5 |
CA-HS-HF-LPME |
GC-FID |
Simple, low cost and effective, good linear range |
Unable to commercialize, poor experiment reproducibility |
6 |
Static headspace |
GC-BID |
Simple, low cost, high sensitivity |
|
7 |
MHS-SPME |
GC-FID |
Avoid matrix effects, it can be extracted continuously many times |
Narrow detection range |
8 |
HS |
GC-PID |
Good linearity and repeatability, low detection limit, it can be monitored online |
Long detection period |
9 |
Vac-HS-SPME |
GC-FID |
Simpler, lower cost and more reliable method, very sensitive |
|
10 |
PC-HS-SPME |
GC-FID |
No sample preparation steps required, super sensitive method with good repeatability |
|
![[thin space (1/6-em)]](https://www.rsc.org/images/entities/char_2009.gif) |
(B) GC–MS |
11 |
QuEChERS programmable temperature vaporizer (PTV) |
GC–MS |
High sensitivity, good linear range, good reproducibility and repeatability, fast |
High cost |
12 |
Vac-HSSPME |
GC–MS |
Fast extraction of target, low detection limit |
Long balancing time |
13 |
RTILs |
GC–MS |
Reduce the matrix effect of soil, lower detection limit |
|
14 |
DSPE DLLE |
GC–MS |
Easy extraction of trace analytes from matrix, simple and fast operation, lower cost, improved separation and enrichment efficiency |
|
15 |
Vac-HSSPME |
GC–MS |
Lower detection limit |
Low content of extracted target analyte |
16 |
Standard addition method HS-SPME |
GC–MS |
Improve recovery |
|
17 |
RTILs static headspace |
GC–MS |
Improve sensitivity and measurement accuracy, reduce matrix effects during analysis |
|
![[thin space (1/6-em)]](https://www.rsc.org/images/entities/char_2009.gif) |
(C) LC |
18 |
Partial least squares (PLS) chemometrics method |
HS-MS |
Without sample pre-treatment, no chromatographic separation required, rapid identification and prediction of samples |
|
19 |
Miniaturized homogeneous liquid–liquid extraction (MHLLE) |
HPLC |
Without sample pre-treatment, fast, simple, and sensitive |
Complex and expensive equipment |
20 |
APPI |
UPLC-APPI-MS/MS |
High sensitivity and high throughput, fast and selective |
|
![[thin space (1/6-em)]](https://www.rsc.org/images/entities/char_2009.gif) |
(D) Sensor method |
21 |
Bioreporter system |
|
Efficient, easy-to-use, low-cost |
Weak mechanical strength, only suitable for low concentration pollution |
22 |
Optical detection biosensor |
|
High mechanical strength, it has stability |
|
23 |
HMOFs |
|
With high selectivity and high acidity and alkalinity, it shows the luminescence quenching effect on BTEX |
|
Apart from the biological sensors, chemical sensors have also been constructed for BTEX and PAHs in recent years. A typical example is a metal–organic framework (MOF)-based sensor. Luminescent MOFs possess various advantages: low cost, simple techniques, low solvent volumes, and obvious changes in phenomenon. MOFs have been increasingly used as a qualitative research method for organic pollutants.139 Myers et al.140 constructed MOFs with high selectivity for the larger methylated aromatic compounds. In addition, heterometal–organic framework (HMOFs) as luminescent sensors started to attract more research interest.22 Zhang et al. proposed a chemical sensor based on 3d–4f microporous HMOFs that were constructed with polydentate carboxylate ligands.24 This sensor (HMOFs) had high selectivity and high acid/base stability, but also exhibited a significant luminescence quenching effect on BTEX, making it a candidate instrument for environmental monitoring. It is worth noting that the sensor method requires further improvement in terms of stability, mechanical strength, and the type of substances to be detected. With optimization, we believe that sensor technology will become a powerful tool for monitoring soil organic pollutants and assessing their toxicity.
3.5 Other methods
In addition to the methods described above, ion mobility spectrometry (IMS) and mass spectrometry (MS) have been used in this field. IMS is an outstanding analytical method owing to its ability to detect and monitor trace levels of volatile and even semi-volatile chemical compounds. It has the advantages of rapid analysis, high sensitivity, portability, and easy operation, making it appropriate for in situ detection.141 It has been reported that BTEX in soil can be studied by introducing online linking between SFE and IMS through a Tenax TA adsorption trap/sampling tube.58 The adsorption/desorption process for Tenax TA is shown in Fig. 5. The use of this solid trap not only collects the target analyte, but also improves the selectivity of the IMS method. Until now, multiple strategies have been designed to introduce volatile compounds to IMS.142,143 If a suitable sampling method144 can be developed, IMS could be used as a sensor for the onsite detection of volatile organic compounds in soil.
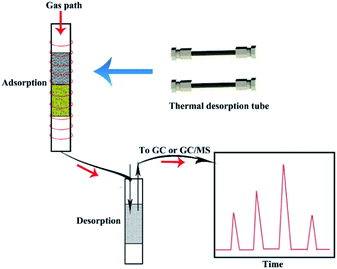 |
| Fig. 5 The adsorption/desorption process of Tenax TA. | |
MS is the preferred detection method after GC separation because it is universal and provides a combination of selectivity and sensitivity that other GC detectors cannot compete with.100 At present, several MS-based analytical methods for detecting BTEX and PAHs in soil have been reported. Examples include headspace mass spectrometry (HS-MS) and ultrahigh-resolution mass spectrometry (UHRMS). The results obtained using HS-MS for the chemometric analysis of BTEX in soil samples were similar to those obtained by the HS-GC–MS method.19 Additionally, Luo and co-workers145 proposed a concept that used multidimensional ionization for UHRMS. With ultrahigh-quality resolution and high-quality accuracy, MS ensures the clear distribution of signals, thus providing a safe and comprehensive view of pollutants in soil at the molecular level. Therefore, MS provides valuable composition information and rapid analysis of PAHs and BTEX in soil, and has great potential for research. Table 3 summarizes the advantages and disadvantages of different pretreatment methods and detection techniques for BTEX and PAHs in soil.
3.6 Comparison of different analysis techniques for BTEX and PAHs in soil
Among the analytical techniques for PAHs and BTEX, GC-FID is the most common technique in laboratories owing to the sensitivity to hydrocarbons and capacity to cover a large range of hydrocarbons. GC can effectively separate and detect PAHs in soil, while MS provides their accurate identification. Therefore, GC–MS is the standard method for the targeted analysis of the 16 PAHs listed by the EPA. According to the USEPA, both HPLC and GC–MS methods are considered equally valid methods for the analysis of PAHs. However, State Environmental Protection Administration (SEPA) recommends the use of HPLC for the determination of PAHs in water. In addition, the separation of non-volatile and trace polar compounds is usually achieved by LC. On the other hand, the successful implementation of sensor may help to monitor pollution conditions for prevention and regulation. In addition, IMS allows high selectivity, high sensitivity, rapid analysis, and small size, making it a candidate instrument for on-site monitoring. In conclusion, GC and GC–MS are the best techniques for the detection of thermally stable organic matter in soils with a boiling point not exceeding 500 °C in the laboratory. Nonetheless, further innovations are still needed to improve the merits of detection techniques, as the detection of PAHs in soil is developed towards simple, rapid, and on-site detection methods.
4 Conclusion and outlook
As an important source of pollution in soil environment, benzene compounds are a complex mixture from a wide range of sources, including PAHs, PCBs, PCNs, BTEX. Increasing attention has been paid to develop novel analytical methods for detecting benzene compounds pollutants. Despite many advances in the environmental analysis of BTEX and PAHs in soil, pretreatment methods still have critical limitations. The traditional Soxhlet extraction is time-consuming, solvent consuming, non-environmentally friendly and non-economical. Compared with Soxhlet extraction, ultrasonic treatment146 is more rapid and practical in extracting hydrocarbons from soil. In recent years, research attention has been attracted to solvent-free extraction techniques, such as static headspace,108 purge and trap, solid-phase microextraction118 for extraction of BTEX and PAHs from soil. However, the disadvantages of these methods are expensive instruments and complex sample preparation. In addition, to overcome drawbacks in SPME, such as the fragility of fibers, high cost of fibers, and passive-sampling, two kinds of new techniques, liquid-phase microextraction (LPME) and needle-trap device (NTD), were developed accordingly.147 The new trend in analytical chemistry refers to the development of “green” sample pretreatment technology that has plenty of merits, including solvent-free or small amounts of solvents, fast analysis, and high selectivity. In addition, in order to select the appropriate extraction method, other factors should be considered, such as time, cost, compatibility with the analytical method and simplicity. In this review, some analytical methods and instruments for extraction and purification of BTEX and PAHs in soil have been described. Consequently, more efforts should be made in future studies to develop diversity and automation of extraction techniques and to increase the advantage of the “green” nature of each pre-concentration.
In recent years, various efficient, rapid, and convenient detection techniques have been developed for the analysis of PAHs in soil. The most commonly used separation and detection techniques are GC, MS, GC–MS, HPLC, LC–MS/MS, and sensor methods. The development of chromatography and mass spectrometry provides invaluable compositional and quantification information of aromatic hydrocarbons in soil. They are extensively employed for the separation and detection of analytes. These detection technologies have the characteristics of high measurement accuracy, good stability, low detection limit and fast detection speed, but they have the disadvantages of complex sample pretreatment, long analysis time and harsh environmental requirements. In addition, disadvantages also include large volume, complex structure and high maintenance cost. As a result, these techniques are limited to laboratory and difficult to be applied for onsite analysis. Furthermore, some sensor methods can provide toxicity information of pollutants, which is beneficial to soil pollution assessment. Biosensor and chemical sensor detections of PAHs have opened up new avenues for low cost, rapid determination, and higher sensitivity. This paper summarizes relevant research work, including recent studies and current research hotspots in this field. Finally, future procedure of pretreatments should provide more innovations and convenience in analytical methods and detection technology. With the application of new processes, new materials, and new technologies, detection of soil organic pollutants will become simple, rapid, low-cost, predictable, and online monitoring.
Conflicts of interest
The authors declare no conflicts of interest.
Acknowledgements
This work was supported by National Key Research and Development Project (2018YFC1800903), Key Research and Development Program in Shaanxi Province (2019ZDLSF01-03), the Natural Science Foundation of Shaanxi Province, China (No. 2020JQ-573), and the Startup Funding of Northwest University of China for setting up the Research Center of Analytical Instrumentation.
References
- C. Peng, W. Chen, X. Liao, M. Wang, Z. Ouyang, W. Jiao and Y. Bai, Environ. Pollut., 2011, 159(3), 802–808 CrossRef CAS PubMed.
- Z. Tang, Y. L. Li, Z. Yang, D. Q. Liu, M. Tang, S. Yang and Y. Tang, Environ. Sci. Pollut. Res., 2019, 26(20), 20277–20285 CrossRef CAS PubMed.
- D. Al Manmi, T.-O. Abdullah, P.-M. Al-Jaf and N. Al-Ansari, Water, 2019, 11(10), 2158 CrossRef CAS.
- C.-H. Wang, S.-L. Zhou, J. Song, J.-H. Tang and S.-H. Wu, Geoderma, 2020, 367, 114271 CrossRef CAS.
- A.-L. Bolden, C.-F. Kwiatkowski and T. Colborn, Environ. Sci. Technol., 2015, 49(19), 11984–11989 CrossRef CAS PubMed.
- Q. Chen, H. Sun, J. Zhang, Y. Xu and Z. Ding, Environ. Sci. Pollut. Res., 2019, 26(11), 10552–10561 CrossRef CAS PubMed.
- E.-J. Werder, L.-S. Engel, A. Blair, R.-K. Kwok, J.-A. McGrath and D.-P. Sandler, Environ. Res., 2019, 175, 100–107 CrossRef CAS PubMed.
- S.-M. Arnold, J. Angerer, P.-J. Boogaard, M.-F. Hughes, R.-B. O'Lone, S.-H. Robison and A.-R. Schnatter, Crit. Rev. Toxicol., 2013, 43(2), 119–153 CrossRef CAS PubMed.
- R.-H. Zhang, L. D.-D. Jiang, S.-J. Wang, D. Zhang, M.-S. Zhong, T.-X. Xia and Q.-K. Fu, Chemosphere, 2020, 255, 126957 CrossRef CAS PubMed.
- M. Farré, S. Pérez, C. Gonçalves, M.-F. Alpendurada and D.-J. Barceló, TrAC, Trends Anal. Chem., 2010, 29(11), 1347–1362 CrossRef.
- R.-J. Luo and W. Schrader, J. Hazard. Mater., 2021, 418, 126352 CrossRef CAS PubMed.
- Y. Xi, D.-A. Duford and E.-D. Salin, Talanta, 2010, 82(3), 1072–1076 CrossRef CAS PubMed.
- N. Zhang, L.-S. Su, S.-N. Man, X.-Y. Lei, T. Huang, C.-L. Zhu, L. Zhang and X.-P. Wu, J. Chromatogr. A, 2019, 1598, 49–57 CrossRef CAS PubMed.
- F. Rasolzadeh and P. Hashemi, Microchim. Acta, 2019, 186(7), 432 CrossRef PubMed.
- S. Seidi and Y. Yamini, Cent. Eur. J. Chem., 2012, 10(4), 938–976 Search PubMed.
- M. Meskar, M. Sartaj and J. Sedano, Environ. Technol., 2019, 40(23), 3040–3053 CrossRef CAS PubMed.
- K. Dalvand and A. Ghiasvand, Anal. Chim. Acta, 2019, 1083, 119–129 CrossRef CAS PubMed.
- V.-U. Okechukwu, D.-O. Omokpariola, V.-I. Onwukeme, E.-N. Nweke and P.-L. Omokpariola, Environ. Anal. Health Toxicol., 2021, 36(4), e2021023 CrossRef PubMed.
- F.-A. Esteve-Turrillas, S. Armenta, S. Garrigues, A. Pastor and M. de la Guardia, Anal. Chim. Acta, 2007, 587(1), 89–96 CrossRef CAS PubMed.
- B. Hindersmann and C. Achten, J. Chromatogr. A, 2017, 1510, 57–65 CrossRef CAS PubMed.
- V. Hernandez-Sanchez, L. Molina, J. Luis Ramos and A. Segura, Microb. Biotechnol., 2016, 9(6), 858–867 CrossRef CAS PubMed.
- S.-R. Zhang, D.-Y. Du, K. Tan, J.-S. Qin, H.-Q. Dong, S.-L. Li, W.-W. He, Y.-Q. Lan, P. Shen and Z.-M. Su, Chem.–Eur. J., 2013, 19(34), 11279–11286 CrossRef CAS PubMed.
- J.-W. Bae, H.-B. Seo, S. Belkin and M.-B. Gu, Anal. Bioanal. Chem., 2020, 412(14), 3373–3381 CrossRef CAS PubMed.
- H. Zhang, J. Ma, D. Chen, J. Zhou, S. Zhang, W. Shi and P. Cheng, J. Mater. Chem. A, 2014, 2(48), 20450–20453 RSC.
- L. Wu, R. Sun, Y. Li and C. Sun, Trends Environ. Anal. Chem., 2019, 24, e00074 CrossRef CAS.
- Q. Zhang, P. Liu, S. Li, X. Zhang and M. Chen, J. Liq. Chromatogr. Relat. Technol., 2020, 43(13–14), 425–444 CrossRef CAS.
- Y. Li, Y. Liu, X. Sun and W. Lu, Huanjing Huaxue-Environmental Chemistry, 2015, 34(8), 1460–1469 CAS.
- Z. Zheng and H. Li, The Administration and Technique of Environmental Monitoring, 2017, 29(5), 1–6 Search PubMed.
- N. Raza, B. Hashemi, K.-H. Kim, S.-H. Lee and A. Deep, TrAC, Trends Anal. Chem., 2018, 103, 56–73 CrossRef CAS.
- E.-V. Lau, S. Gan and H.-K. Ng, Int. J. Anal. Chem., 2010, 2010, 398381 CAS.
- L.-I. Xian-Guo, Z. Qing-Hong, L.-I. Si-Min and D. Chang-Xu, J. Ocean Univ. China, 2009, 39(3), 467–475 Search PubMed.
- Z. Khan, J. Troquet and C. Vachelard, Int. J. Environ. Sci. Technol., 2005, 2(3), 275–286 CrossRef CAS.
- D.-E. Raynie, LCGC North Am., 2019, 37(8), 510–513 CAS.
- M. Klees, C. Bogatzki and E. Hiester, J. Chromatogr. A, 2016, 1468, 10–16 CrossRef CAS PubMed.
- W.-Z. Zhou, H. Zhang, C. Deng, Y. Chen, J. Liao, Z.-M. Chen and J.-M. Xu, J. Chromatogr. A, 2019, 1604, 460473 CrossRef CAS PubMed.
- S. Ncube, G. Lekoto, E. Cukrowska and L. Chimuka, J. Sep. Sci., 2018, 41(4), 918–928 CrossRef CAS PubMed.
- N.-B. Hlengwa and P.-N. Mahlambi, Bull. Environ. Contam. Toxicol., 2020, 104(4), 464–470 CrossRef CAS PubMed.
- R. Heydari, A. Shakarami and M. Kaykhaii, J. Chil. Chem. Soc., 2019, 64(1), 4332–4336 CrossRef CAS.
- S.-A. Mokhtari, M. Farzadkia, A. Esrafili, R.-R. Kalantari and M. Gholami, Desalin. Water Treat., 2017, 66, 176–183 CrossRef CAS.
- T.-F. Guerin, J. Environ. Monit., 1999, 1, 63–67 RSC.
- J.-R. Dean and G.-J. Xiong, TrAC, Trends Anal. Chem., 2000, 19(9), 553–564 CrossRef CAS.
- M.-D. Castro and A.-A. Garc, Anal. Chim. Acta, 1998, 369(1–2), 1–10 CrossRef.
- M.-D. Luque de Castro and F. Priego-Capote, J. Chromatogr. A, 2010, 1217(16), 2383–2389 CrossRef CAS PubMed.
- F. Portet-Koltalo, Y. Tian, I. Berger-Brito, A. Benamar, C. Boulange-Lecomte and N. Machour, Talanta, 2021, 221, 121601 CrossRef CAS PubMed.
- C. Li, X. Zhang, Y. Shi, H. Jia, W. Shi, W. Wang and Z. Yang, Huanjing Huaxue-Environmental Chemistry, 2017, 36(1), 190–197 CAS.
- V.-S. Jovanovic, V. Mitic, S. Ciric, M. Ilic, J. Nikolic, M. Dimitrijevic and G. Stojanovic, Anal. Lett., 2017, 50(15), 2491–2504 CrossRef.
- O.-H. Szolar, H. Rost, R. Braun and A.-P. Loibner, Anal. Chem., 2002, 74(10), 2379–2385 CrossRef CAS PubMed.
- Y.-T. Zhang, G.-X. Zhao, J.-S. Liu, L. Zhang, X.-Y. Li, J.-Y. Gui and C.-L. Zhang, J. Groundwater Sci. Eng., 2017, 5(3), 249–253 Search PubMed.
- E. Alsbou, M.-A. Zaitoun, A.-M. Alasoufi and A. Al Shra'ah, Arch. Environ. Contam. Toxicol., 2019, 77(4), 619–630 CrossRef CAS PubMed.
- P.-N. Kunene and P.-N. Mahlambi, J. Environ. Chem. Eng., 2020, 8, 103665 CrossRef CAS.
- T. Oluseyi, K. Olayinka, B. Alo and R.-M. Smith, Int. J. Environ. Res., 2011, 5(3), 681–690 CAS.
- F. Sun, D. Littlejohn and M.-D. Gibson, Anal. Chim. Acta, 1998, 364(1), 1–11 CrossRef CAS.
- R. Heydari, A. Shakarami and M. Kaykhaii, J. Chil. Chem. Soc., 2019, 64(1), 4332–4336 CrossRef CAS.
- R. Heydari, A. Shakarami and M. Kaykhaii, J. Chil. Chem. Soc., 2019, 64(1), 4332–4336 CrossRef CAS.
- S. Seidi and Y. Yamini, Cent. Eur. J. Chem., 2012, 10(4), 938–976 Search PubMed.
- B. Albero, J.-L. Tadeo and R.-A. Perez, TrAC, Trends Anal. Chem., 2019, 118, 739–750 CrossRef CAS.
- G. Madras, C. Erkey, A. Akgerman and S. Energy, Environ. Prog. Sustainable Energy, 2010, 13, 45–50 Search PubMed.
- N. Jurado-Campos, A. Carpio, M. Zougagh, L. Arce and N. Arroyo-Manzanares, Talanta, 2018, 188, 637–643 CrossRef CAS PubMed.
- S.-M. Pourmortazavi, Z. Saghafi, A. Ehsani and M. Yousefi, J. Food Sci. Technol., 2018, 55(8), 2813–2823 CrossRef CAS PubMed.
- C. Miege, J. Dugay and M.-C. Hennion, J. Chromatogr. A, 1998, 823(1–2), 219–230 CrossRef CAS PubMed.
- M. Elektorowicz, H. El-Sadi, J. Lin and T. Ayadat, J. Colloid Interface Sci., 2007, 309(2), 445–452 CrossRef CAS PubMed.
- S. Motamedimehr and S. Gitipour, Pollution, 2019, 5(4), 913–922 CAS.
- H. Larque-Garcia, L.-W. Torres-Tapia, E. Del Olmo-Fernandez, E. Sanchez-Arreola and S.-R. Peraza-Sanchez, J. Supercrit. Fluids, 2020, 163, 104859 CrossRef CAS.
- I.-D. Valente, T.-C. Confortin, L. Luft, G.-A. Ugalde, G.-L. Zabot, M.-A. Mazutti and L.-D. Terra, J. Supercrit. Fluids, 2018, 136, 52–59 CrossRef CAS.
- M. Schnitzer and C.-M. Preston, Soil Sci. Soc. Am. J., 1987, 51, 639–646 CrossRef CAS.
- M. Zougagh, TrAC, Trends Anal. Chem., 2004, 23(5), 399–405 CrossRef CAS.
- M. Zougagh and A. Rios, Electrophoresis, 2008, 29(15), 3213–3219 CAS.
- A.-D. Sanchez-Camargo, F. Parada-Alfonso, E. Ibanez and A. Cifuentes, J. Sep. Sci., 2017, 40(1), 213–227 CrossRef CAS PubMed.
- A.-E. Latawiec and B.-J. Reid, Chemosphere, 2010, 78(8), 1042–1048 CrossRef CAS PubMed.
- C. Erger and T.-C. Schmidt, TrAC, Trends Anal. Chem., 2014, 61, 74–82 CrossRef CAS.
- O. Zuloaga, P. Navarro, E. Bizkarguenaga, A. Iparraguirre, A. Vallejo, M. Olivares and A. Prieto, Anal. Chim. Acta, 2012, 736, 7–29 CrossRef CAS PubMed.
- A. Speltini, M. Sturini, F. Maraschi, L. Consoli, A. Zeffiro and A. Profumo, J. Chromatogr. A, 2015, 1379, 9–15 CrossRef CAS PubMed.
- Y.-P. Duan, C.-M. Dai, Y.-L. Zhang and L.-C. Acta, Anal. Chim. Acta, 2013, 758(758), 93–100 CrossRef CAS PubMed.
- S. Babic and D.-M. Pavlovic, Compr. Anal. Chem., 2013, 62, 129–167 CAS.
- D. Kluk and T. Steliga, Nafta Gaz., 2017, 73(7), 488–495 CrossRef.
- Y.-N. Zhang, X.-L. Yang, Y.-R. Bian, C.-G. Gu, D.-Z. Wang and X. Jiang, Chin. J. Anal. Chem., 2016, 44(10), 1514–1519 CAS.
- M.-J. Yoon and T. Gradworks, Analytical method development for measurement of unregulated organic contaminants in aqueous samples using liquid chromatography – tandem mass spectrometry, dissertations & theses – gradworks, Rutgers, The State University of New, Jersey, 2010.
- E. Martendal and E. Carasek, J. Chromatogr. A, 2011, 1218(13), 1707–1714 CrossRef CAS PubMed.
- H.-M. Liu, F.-P. Ran, X.-Q. Wang, N.-R. He and Y. Guo, Microchim. Acta, 2018, 185(1), 82 CrossRef PubMed.
- J. Feng, M. Sun, Y. Bu and C. Luo, Talanta, 2016, 148, 313–320 CrossRef CAS PubMed.
- A. Gutierrez-Serpa, I. Pacheco-Fernandez, J. Pasan and V. Pino, Separations, 2019, 6(4), 47 CrossRef CAS.
- E. Akbari, A. Ghiasvand and K. Dalvand, Microchem. J., 2020, 158, 105201 CrossRef CAS.
- Z. Wang, M. He, B.-B. Chen and B. Hu, J. Chromatogr. A, 2020, 1616, 460793 CrossRef CAS PubMed.
- L.-Y. Xu, S.-M. Huang, Y. Liu, S.-B. Wei, G.-S. Chen, Z.-J. Gong and G.-F. Ouyang, Anal. Chim. Acta, 2020, 1097, 85–93 CrossRef CAS PubMed.
- A.-R. Ghiasvand, S. Hosseinzadeh and J. Pawliszyn, J. Chromatogr. A, 2006, 1124(1–2), 35–42 CrossRef CAS PubMed.
- S. Xu, H. Li, H. Wu, L. Xiao, P. Dong, S. Feng and J. Fan, Anal. Chim. Acta, 2020, 1115, 7–15 CrossRef CAS PubMed.
- A.-R. Ghiasvand and M. Pirdadeh-Beiranvand, Anal. Chim. Acta, 2015, 900, 56–66 CrossRef CAS PubMed.
- E. Yiantzi, N. Kalogerakis and E. Psillakis, Anal. Chim. Acta, 2015, 890, 108–116 CrossRef CAS PubMed.
- E. Psillakis, E. Yiantzi, L. Sanchez-Prado and N. Kalogerakis, Anal. Chim. Acta, 2012, 742, 30–36 CrossRef CAS PubMed.
- E. Psillakis, A. Mousouraki, E. Yiantzi and N. Kalogeraki, J. Chromatogr. A, 2012, 1244, 55–60 CrossRef CAS PubMed.
- J. De Crom, S. Claeys, A. Godayol, M. Alonso, E. Antico and J.-M. Sanchez, J. Sep. Sci., 2010, 33(17–18), 2833–2840 CrossRef CAS PubMed.
- H.-L. Lord, W.-Q. Zhan and J. Pawliszyn, Anal. Chim. Acta, 2010, 677(1), 3–18 CrossRef CAS PubMed.
- Y. Qin, Y. Pang and Z. Cheng, Phytochem. Anal., 2016, 27(6), 364–374 CrossRef CAS PubMed.
- A. Poormohammadi, A. Bahrami, M. Farhadian, F. Ghorbani Shahna and A. Ghiasvand, J. Chromatogr. A, 2017, 1527, 33–42 CrossRef CAS PubMed.
- A.-P. Schwab, J. Su, S. Wetzel, S. Pekarek and M.-K. Banks, Environ. Sci. Technol., 1999, 33, 1940–1945 CrossRef CAS.
- M.-G. Nespeca, R. Sequinel and J.-E. de Oliveira, Microchem. J., 2019, 150, 104163 CrossRef CAS.
- Y.-I. Pikovskii, L.-A. Korotkov, M.-A. Smirnova and R.-G. Kovach, Eurasian Soil Sci., 2017, 50, 1125–1137 CrossRef CAS.
- R.-N. Okparanma and A.-M. Mouazen, Appl. Spectrosc. Rev., 2013, 48, 458–486 CrossRef CAS.
- A. Horta, B. Malone, U. Stockmann, B. Minasny, T.-F. Bishop, A.-B. McBratney, R. Pallasser and L. Pozza, Geoderma, 2015, 241, 180–209 CrossRef.
- M. Galmiche, O. Delhomme, Y.-N. Francois and M. Millet, TrAC, Trends Anal. Chem., 2021, 134, 116146 CrossRef CAS.
- Y.-Y. Zhou, J.-F. Yu, Z.-G. Yan, C.-Y. Zhang, Y.-B. Xie, L.-Q. Ma, Q.-B. Gu and F.-S. Li, Environ. Monit. Assess., 2013, 185(4), 3037–3048 CrossRef CAS PubMed.
- K. Wojtowicz, Nafta Gaz., 2018, 74(3), 201–207 CrossRef.
- R. Pascale, G. Bianco, S. Calace, S. Masi, I.-M. Mancini, G. Mazzone and D. Caniani, J. Chromatogr. A, 2018, 1548, 10–18 CrossRef CAS PubMed.
- A. Ghiasvand, F. Zarghami and M. Beiranvand, J. Iran. Chem. Soc., 2018, 15(5), 1051–1059 CrossRef CAS.
- A.-R. Ghiasvand and N. Heidari, Chromatographia, 2016, 79(17–18), 1187–1195 CrossRef CAS.
- M. Shamsipur and J. Hassan, J. Chromatogr. A, 2010, 1217(30), 4877–4882 CrossRef CAS PubMed.
- E. Yiantzi, N. Kalogerakis and E. Psillakis, Anal. Chim. Acta, 2015, 890, 108–116 CrossRef CAS PubMed.
- E. Varona-Torres, D.-D. Carlton, Z.-L. Hildenbrand and K.-A. Schug, Anal. Chim. Acta, 2018, 1021, 41–50 CrossRef CAS PubMed.
- M. Khajeh, A.-A. Moosavi-Movahedi, M. Shakeri, F.-M. Zadeh, A. Khajeh and M. Bohlooli, J. Chemom., 2015, 29(4), 245–252 CrossRef CAS.
- D. Orazbayeva, B. Kenessov and J.-A. Koziel, Int. J. Biol. Chem., 2018, 11, 4–11 CrossRef CAS.
- C. Bicchi, C. Brunelli, C. Cordero, P. Rubiolo, M. Galli and A. Sironi, J. Chromatogr. A, 2005, 1071(1–2), 3–12 CrossRef CAS PubMed.
- A. Robbat, Field Anal. Chem. Technol., 2001, 5(1–2), 60–68 CrossRef CAS.
- M.-G. Nespeca, R. Sequinel and J.-E. de Oliveira, Microchem. J., 2019, 150, 104163 CrossRef CAS.
- N. Rombaut, A.-S. Tixier, A. Bily and F. Chemat, Biofuels, Bioprod. Biorefin., 2014, 8(4), 530–544 CrossRef CAS.
- S. Xu, Q. Shuai and J. Pawliszyn, Anal. Chem., 2016, 88(18), 8936–8941 CrossRef CAS PubMed.
- N.-A. Qambrani, J.-H. Hwang and S.-E. Oh, Chemosphere, 2016, 160, 342–348 CrossRef CAS PubMed.
- S. Gorska, M. Delis and P. Chaber, Przem. Chem., 2012, 91(4), 569–573 CAS.
- D. Orazbayeva, B. Kenessov, J.-A. Koziel, D. Nassyrova and N.-V. Lyabukhova, Chromatographia, 2017, 80(8), 1249–1256 CrossRef CAS.
- M. Galmiche, O. Delhomme, Y.-N. Francois and M. Millet, TrAC, Trends Anal. Chem., 2021, 134, 116146 CrossRef CAS.
- M. Blessing, M.-A. Jochmann, S.-B. Haderlein and T.-C. Schmidt, Rapid Commun. Mass Spectrom., 2015, 29(24), 2349–2360 CrossRef CAS PubMed.
- C.-G. Pinto, S.-H. Martin, J.-L. Pavon and B.-M. Cordero, Anal. Chim. Acta, 2011, 689(1), 129–136 CrossRef PubMed.
- S.-S. Cai, J. Stevens and J.-A. Syage, J. Chromatogr. A, 2012, 1227, 138–144 CrossRef CAS PubMed.
- C. Gonzalez-Pinuela, R.-M. Alonso-Salces, A. Andres, I. Ortiz and J.-R. Viguri, J. Chromatogr. A, 2006, 1129(2), 189–200 CrossRef CAS PubMed.
- S.-C. Lung and C.-H. Liu, Sci. Rep., 2015, 5, 12992 CrossRef CAS PubMed.
- S.-S. Cai, J.-A. Syage, K.-A. Hanold and M.-P. Balogh, Anal. Chem., 2009, 81(6), 2123–2128 CrossRef CAS PubMed.
- B. Gill, A. Mell, M. Shanmuganathan, K. Jobst, X. Zhang, D. Kinniburgh, N. Cherry and P. Britz-McKibbin, Anal. Bioanal. Chem., 2019, 411(7), 1397–1407 CrossRef CAS PubMed.
- T. Miyawaki, K. Tobiishi, S. Takenaka and K. Kadokami, Soil Sediment Contam., 2018, 27(1), 31–45 CrossRef CAS.
- B. Gevao, E.-A. Boyle, G.-G. Carrasco, A.-N. Ghadban, J. Zafar and M. Bahloul, Mar. Pollut. Bull., 2016, 112(1–2), 218–224 CrossRef CAS PubMed.
- F. Ben Salem, O. Ben Said, R. Duran and M. Monperrus, Bull. Environ. Contam. Toxicol., 2016, 96(5), 678–684 CrossRef CAS PubMed.
- S. Chatzimichail, F. Rahimi, A. Saifuddin, A.-J. Surman, S.-D. Taylor-Robinson and A. Salehi-Reyhani, Commun. Chem., 2021, 4(1), 17 CrossRef CAS.
- D. Pan, J. Wang, C. Chen, C.-A. Huang, Q.-Y. Cai and S.-Z. Yao, Talanta, 2013, 108, 117–122 CrossRef CAS PubMed.
- S. Garcia-Alonso, A.-I. Barrado-Olmedo and R.-M. Perez-Pastor, Polycyclic Aromat. Compd., 2012, 32(5), 669–682 CrossRef CAS.
- S. Tomaz, P. Shahpoury, J.-L. Jaffrezo, G. Lammel, E. Perraudin, E. Villenave and A. Albinet, Sci. Total Environ., 2016, 565, 1071–1083 CrossRef CAS PubMed.
- N.-N. Xi, W. Guo, J.-L. Feng, S.-H. Liu and J.-H. Sun, Environ. Forensics, 2019, 20(2), 171–181 CrossRef CAS.
- O. Delhomme and M. Millet, Polycyclic Aromat. Compd., 2008, 28(4–5), 518–532 CrossRef CAS.
- S. Krol, B. Zabiegala and J. Namiesnik, TrAC, Trends Anal. Chem., 2010, 29(9), 1092–1100 CrossRef CAS.
- Y. Paitan, I. Biran, N. Shechter, D. Biran, J. Rishpon and E.-Z. Ron, Anal. Biochem., 2004, 335(2), 175–183 CrossRef CAS PubMed.
- S. Belkin, Curr. Opin. Microbiol., 2003, 6(3), 206–212 CrossRef CAS PubMed.
- Q. Tang, S.-X. Liu, Y.-W. Liu, J. Miao, S.-J. Li, L. Zhang, Z. Shi and Z.-P. Zheng, Inorg. Chem., 2013, 52(6), 2799–2801 CrossRef CAS PubMed.
- M. Myers, A. Podolska, C. Heath, M.-V. Baker and B. Pejcic, Anal. Chim. Acta, 2014, 819, 78–81 CrossRef CAS PubMed.
- S. Hajialigol, S.-A. Ghorashi, A.-H. Alinoori, A. Torabpour and M. Azimi, J. Chromatogr. A, 2012, 1268, 123–129 CrossRef CAS PubMed.
- L. Arce, M. Menéndez, R. Garrido-Delgado and M. Valcárcel, TrAC, Trends Anal. Chem., 2008, 27(2), 139–150 CrossRef CAS.
- L. Criado-Garcia, N. Almofti and L. Arce, Sens. Actuators, B, 2016, 235, 370–377 CrossRef CAS.
- A. Galuszka, Z.-M. Migaszewski and J. Namiesnik, Environ. Res., 2015, 140, 593–603 CrossRef CAS PubMed.
- R.-J. Luo and W. Schrader, Polycyclic Aromat. Compd., 2020, 42(2), 643–658 CrossRef.
- P. Zhao, W. Gong, G. Mao, J. Li, F. Xu and J. Shi, Chin. J. Oceanol. Limnol., 2016, 34, 539–548 CrossRef CAS.
- C.-M. Santana, Z.-S. Ferrera, M.-E. Padrón and J.-J. Rodríguez, TrAC, Trends Anal. Chem., 2011, 30, 731–748 CrossRef.
Footnote |
† Electronic supplementary information (ESI) available. See DOI: 10.1039/d1ra08633b |
|
This journal is © The Royal Society of Chemistry 2022 |