DOI:
10.1039/D1RA08487A
(Review Article)
RSC Adv., 2022,
12, 5749-5764
Bio-electrochemical frameworks governing microbial fuel cell performance: technical bottlenecks and proposed solutions
Received
19th November 2021
, Accepted 10th February 2022
First published on 16th February 2022
Abstract
Microbial fuel cells (MFCs) are recognized as a future technology with a unique ability to exploit metabolic activities of living microorganisms for simultaneous conversion of chemical energy into electrical energy. This technology holds the promise to offer sustained innovations and continuous development towards many different applications and value-added production that extends beyond electricity generation, such as water desalination, wastewater treatment, heavy metal removal, bio-hydrogen production, volatile fatty acid production and biosensors. Despite these advantages, MFCs still face technical challenges in terms of low power and current density, limiting their use to powering only small-scale devices. Description of some of these challenges and their proposed solutions is demanded if MFCs are applied on a large or commercial scale. On the other hand, the slow oxygen reduction process (ORR) in the cathodic compartment is a major roadblock in the commercialization of fuel cells for energy conversion. Thus, the scope of this review article addresses the main technical challenges of MFC operation and provides different practical approaches based on different attempts reported over the years.
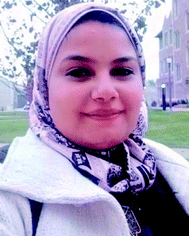 Rehab H. Mahmoud | Rehab H. Mahmoud is a Senior Researcher in the Water Pollution Research Department, National Research Centre (NRC), Egypt. She received her Ph.D. degree in Environmental Microbiology from Ain Shams University, Egypt in 2018. She is currently a visiting scholar at Washington University in St. Louis, USA. Her research work focuses on environmental biotechnology, microbial fuel cells, bioenergy, and biosensors. |
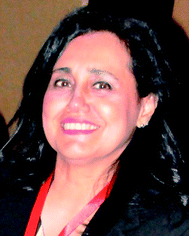 Ola M. Gomaa | Ola M. Gomaa is a Professor of Microbial Biotechnology at the Egyptian Atomic Energy Authority. Her main focus is biofilm engineering and studying biofilm properties to enhance the performance of MFCs specifically and bioremediation in general. Her research on enhancing cell adhesion to electrode surfaces for wastewater treatment has been the main priority in her lab for the past 10 years. She is a Newton-Mosharafa Alumnus, UK Academy of Medical Sciences Alumnus, Daniel Turnberg Alumnus, and a Fulbright Alumnus. She started her role as an Ambassador for ISMET Africa chapter in 2021. |
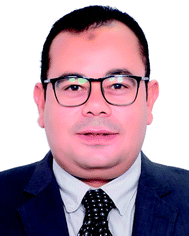 Rabeay Y. A. Hassan | Rabeay Y. A. Hassan is an Associate Professor in the Nanoscience Program, Zewail City of Science and Technology (Cairo, Egypt). In 2011, he received a PhD in Bioanalytical Chemistry from Braunschweig University of Technology (Germany). In 2013, he joined the group of Biosensors and Bioelectronics, University of Technology Potsdam, Germany, as a postdoctoral research fellow. In 2014, he joined the group of Proteomics and Microbiology, UMONS in Mons, Belgium. In 2015, he was a visiting scholar at University of California, USA. He has been awarded several scientific fellowships such as DAAD, Erasmus-Munds and American National Academy of Sciences. His major research interests are microbial electrochemistry, electrochemical nanosensors and biosensors. |
1. Introduction
Microbial fuel cells (MFCs) are bio-electrochemical systems that exploit living-microorganism metabolites to generate an electrical current through the oxidation of a variety of degradable organic substances.1 In addition, anaerobic microbes can obtain electrons from the oxidation of inorganic electron donors such as sulfide.2 This concept of producing electricity using microbes was first published by M. C. Potter in 1911 (ref. 3) using Escherichia coli. After a series of developments, especially from 1990 onwards, the power output of MFCs improved with a reduction in the component and operating costs.4,5 Recently, MFCs have been used as power sources for miniature and lab scale electronic apparatus.6 Recognizable improvement has also been achieved for wastewater treatment, water desalination, biohydrogen production, and biosensors by MFCs.7–10 However, to date scale-up and commercialization of MFCs aren't economically feasible, mainly owing to their low stability, high operating cost and low current generation.11 This review article is dedicated to providing the readers, both students and professionals, with an overall view and thorough discussion of the main limiting factors of MFC operation and suggestions are made to improve performance.
2. Functioning of the microbial fuel cell (MFC)
An MFC is a bioelectrochemical system that produces electricity by converting chemical energy stored in the organic substrates to useful electrical energy by utilizing the catalytic activities of microorganisms.12 Mainly, the function of an MFC can be visualized from the schematic of a basic configuration of MFC presented in Fig. 1. Several other novel and potentially effective configurations of MFCs have been developed, regardless, the main function, as described below, remains constant for any given MFC configuration. The basic MFC design consists of an anode and a cathode separated by a proton exchange membrane (PEM). For the anodic reaction, living exoelectrogenic or electroactive organisms catalyze the breakdown of organic degradable matter (electron donors) that are transferred from the bulk solution via the cellular metabolism to the anodic surface with the generation of electrons.13 Convenient electrocatalysts of biological origin complete the reduction reactions at the cathode, where the protons transported through the PEM are combined with electrons and oxygen to form water molecules. It is confirmed that electrons could be liberated from any biodegradable substrate, varying from pure fuels including acetate, glucose, cysteine, ethanol, and bovine serum albumin to complex mixtures of organic substances such as domestic, animal, meat-packing, and food-processing wastewaters.14
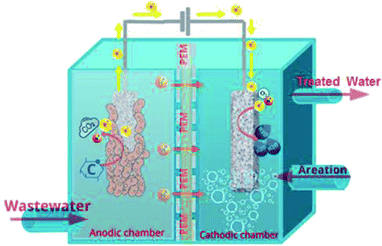 |
| Fig. 1 A schematic representation of a double-chamber microbial fuel cell (MFC) device showing the cathodic and anodic compartments separated by a proton exchange membrane (PEM). | |
3. Microbe-electrode synergy
One of the most important key aspects of MFC technology lies in the mechanism by which microorganisms adhere to the electrode surface and the subsequent electron transfer that takes place. Since electrodes are surfaces that cannot enter the bacterial cells, the main requisite is that electrons are to be transferred from the inside living microbial cell membrane to the outer membrane either through the physical transfer of reduced compounds or via electron bouncing across the membrane using membrane-bound redox enzymes.15 Regardless of the mechanism, the extracellular electron transfer must result in a redox-active species that can link the bacterial cell to the electrode electronically. This species may be a soluble redox shuttle, a reduced primary metabolite, or an outer membrane redox protein.15 To this date, several extracellular electron transfer (EET) mechanisms between living microorganisms and electrodes have been proposed. As shown in Fig. 2, two different mechanisms can be used for connecting the living microbial cells with the electrode surface through direct electron transfer (DET) or mediated electron transfer (MET). The full description of each mechanism is given in the next section.
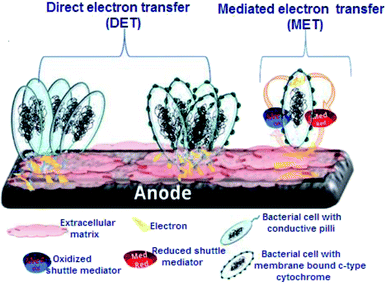 |
| Fig. 2 Illustration of the extracellular electron transfer in the microbial electrochemical systems. The direct microbial electron transfer (DET) is enabled through the growing of conductive pili, or by membrane-bound c-type cytochrome on the microbial cell wall. The mediated electron transfer (MET) was conducted either via the reduced shuttle mediators or secretion of electroactive secondary metabolite(s). | |
3.1. Direct electron transfer (DET)
The DET mechanism is dependent on the ability of electroactive bacteria, also known as exoelectrogens to transfer electrons extracellularly to conductive materials directly without mediators via anodic respiration.16,17 DET which occurs through the physical contact between the bacterial cell membrane or a membrane organelle and the electrode surface18 can be carried out by bacterial membrane-redox-active proteins (c-type cytochromes).19–22 Likewise, some microbes can produce conductive nanowires, which represent a modified form of DET.23,24
For example, Shewanella oneidensis MR-1 produces conductive filaments that are extensions of the outer membrane, thus they allow electrons flow from the cell membrane to a terminal electron acceptor.25–28 Geobacter sulfurreducens also produces a type-IV pili which is capable of electrical conductivity via overlapping pi–pi orbitals that exist in aromatic amino acids.29 DET is not limited to bacteria, a wide range of microbes were also reported as potential exoelectrogenic due to evidence of DET mechanism. This has been explained by a mediator-less bioelectrochemical approach for studying the intracellular level of Candida albicans.30,31 Lately, several studies started screening various algal species for their exoelectrogenic activity and the probability of direct electron transfer. One of the most interesting findings was that after achieving suitable growth conditions with the absence of light and inorganic nutrients which resulted in a significant decrease in the photosynthetic oxygen production, the blue-green alga Oscillatoria agardhii was able to transfer electrons directly without adding artificial redox mediators.32
3.2. Mediated-MFCs (MMFCs) challenges
Some microbes are electrochemically inactive and require soluble chemical redox mediators to transfer their electrons to the electrode surfaces.33,34 In order to enhance the rate of the electron transfer, many attempts were made to overcome the kinetic, and thermodynamic obstacles. Several synthetic electron mediators have been commonly used to transfer the electrons by replacing the oxygen to accept the electrons during the microbial respiration chain between the microbe and electrode, which is called mediated electron transfer.35 There are two types of artificial mediators that are widely used, i.e., hydrophilic mediators (such as potassium ferricyanide) and lipophilic mediators such as benzoquinone, menadione, dichloroindophenol, 2,3,5,6-tetramethylphenylenediamine. Synthetic dyes can also act as mediators in MFC, in that essence neutral red was reported to enhance mediated electron transfer and increased the longevity of MFC performance as compared to MFC without neutral red.36 Hydrophilic mediators are unable to move across the cell membrane and are limited to reacting with the proteins located on the periplasm, however, they have the advantages of high water solubility and high diffusion coefficient in the aquatic systems, which enhance microbial fuel cells operation.37,38 On the other hand, lipophilic mediators such as menadione are able to permeate across the cell membrane, interacting with the intracellular redox centers in cytoplasm and mitochondria, being decreased by the intracellular enzymes, and diffusing outside the cell to transfer electrons to the electrode surface. Nonetheless, using a lipophilic mediator as a sole mediator in the MFC may not be a good choice, because their poor aqueous solubility can significantly affect their concentration in the detection system and hence impact the amplitude of the current signals. To resolve the aforementioned issue, a double-mediator system composed of a hydrophilic and a lipophilic mediator will compensate for their shortcomings and allows the intracellular redox processes, expressing intracellular cell metabolism activities of target cells and high current signal intensity.38 The potassium ferricyanide–menadione double-mediator system is widely applied to analyze the redox activity of S. cerevisiae yeast cells and mammalian cells. Moreover, this double-mediator system has been used to investigate a specific enzyme activity or biochemical process in yeast cells,39,40 single-cell imaging using scanning electrochemical microscopy (SECM),41 and the application in MFCs,42 etc. Even though combining mediators can dramatically increase the current magnitude and thus boost the MFC efficiency, there are significant disadvantages. When microbes are continuously provided with supplementary electron shuttles, it is not easy to save energy for activities such as cell growth and maintenance, which is very important when considering risks for industrial MFC systems.35 In addition to that, synthetic soluble mediators can be leached out in flow systems for practical use, increasing the expense of the process and being unfriendly in terms of environmental protections. To address this limitation, mediators were immobilised on electrodes using different chemical methods. However, due to the reduced mobility (or permeability) of immobilised mediators, this technique was expected to inhibit the mediated electron transfer rate between microbes and electrodes.43 Furthermore, the costs of incorporating artificial mediators into continuous, commercial-scale processes would increase operation costs. Moreover, artificial mediators are introduced into the ecosystem, they can have unanticipated implications for human health and other living organisms.44
3.3. Self-mediator secretion
Microorganisms usually adapt to harsh environmental conditions of nutrient limitation or presence of xenobiotics. They resort to a multiplex of stress response strategies to overcome the stressful condition. For example, in conditions where biofilms are extremely dense and DET mechanisms are limited due to spatial constraints, microbes secrete water-soluble electroactive metabolite(s) in the extracellular matrix as an alternative mechanism to promote self-mediation of electron transport.15,45 The two microbes that have received the most attention in terms of self-mediator secretion are Shewanella oneidensis and Pseudomonas aeruginosa. The Gram-negative microbe, S. oneidensis, is able to secrete various mediators like flavin mononucleotide (FMN) and riboflavin.46–49 Marsili et al. reported that up to 75% of the extracellular electron transfer (EET) in S. oneidensis is self-mediated.50 S. oneidensis mutants deficient in secreting flavins (because of deletion of bfe gene) showed a decrease in the current output when its bio-electrochemical performance was compared with the wild strain.51,52 P. aeruginosa, an opportunistic Gram-negative pathogen, is the second most studied microbe for MET. Pure cultures of P. aeruginosa KRP1 isolated from anode biofilms established MET via the secretion of phenazines, as the quorum sensing (QS) molecules, in particular, pyocyanin and phenazine-1-carboxamide.46,53 Under aerobic conditions, pyocyanin is believed to be active in lipid and carbohydrate degradation as well as electron transfer to oxygen as a terminal electron acceptor.54 Even then, anaerobic condition studies indicated that pyocyanin is able to interact with iron as a terminal electron acceptor.55 Exploiting this kind of mediator self-producing microorganisms to run MFC is a good alternative to artificial mediators, but it necessitates the isolation and analysis of a large number of microbes that are capable of producing endogenous mediators.
4. Characteristics of electroactive biofilms
The biofilm formed by electrogenic microbes is known as the “powerhouse” of MFCs because it serves as the source of electrons to the electrodes for the functioning of MFCs.56 Biofilms are communities of bacteria that assemble to form a single or diverse organism(s) performing various metabolic activities.56 The existence of the electrocatalytically active anode surface is an important factor to remember when forming a matured electrochemical active biofilm from mixed culture.57–60 There are many techniques available to determine when an electrochemically active biofilm has been formed, including electrochemical methods such as cyclic voltammetry (CV), electrochemical impedance spectroscopy (EIS),61 microscopy, biological analysis, and Raman spectroscopy. Some of these techniques have drawbacks in that they often damage the biofilms or require laboratory conditions that are harmful to microbial cells. CV studies revealed the direct electrode interaction of Fe(III) reducing bacteria, e.g. Shewanella putrefaciens, is only enabled when the cells are incubated with the working electrode under anaerobic conditions, whereas the electrode serves as the sole electron acceptor.20,62 As a result, a quasi-reversible CV with a defined cathodic peak at −0.32 V and an anodic peak at 0.03 V against a saturated calomel electrode (SCE) were obtained.62 Redox peaks cannot be found in CV voltammograms in the presence of oxygen since oxygen is an electron acceptor. These findings indicated that, as long as anaerobic conditions were persevered, direct electron exchange using electroactive living organisms would be possible. As a result, the DET was then investigated using a mediator-less-MFC, and substantial current output was observed when the dissolved oxygen content was decreased in the anodic chamber.63,64 Enrichment of a single strain from a consortium of living cultures, accompanied by biofilm formation, could lead to the discovery of novel electrochemically active isolated species from mediator-less microbial fuel cells.
Voltammetric technique can be run in real-time without requiring extensive preparation of the reactor vessel and are non-invasive; nonetheless, it changes the microbial environment for a brief period, which can influence microbial behaviour and its state during that period and potentially for a short time afterward. CV can be used coupled with other physical characterization techniques such as scanning electron microscopy, to image and visualize the mature biofilm formation at the electrode surface as shown in Fig. 3A and B.
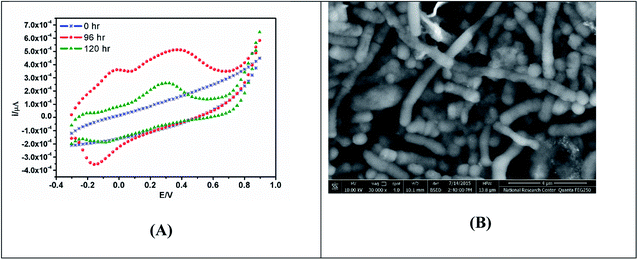 |
| Fig. 3 (A) CV showing bio-electrochemical responses of biofilm formation of bacterial mixed cultures grown on the MnO2-modified screen printed electrode for 120 h under anaerobic condition. (B) SEM image of the modified screen printed electrode (SPE) incubated in bacterial mixed cultures.68 | |
SEM creates a 3D image and detects extracellular polymeric substances (EPS) in biofilms using scattering and absorption of electrons on the biofilm surface.65 On the other hand, SEM is a time-consuming technique that necessitates the fixation, and dehydration of each sample, which can damage biofilms and collapse EPS structures.66,67 Cryo-SEM, environmental-SEM, and focused ion beam-SEM (FIB-SEM) are SEM modifications that mostly skip the disruptive sample preparation steps but result in lower resolution images.66 The downside of many of these techniques is that the biofilms cease to be viable during imaging and it is difficult to see the working nature of an electro-active biofilm. To address these drawbacks, microscopy is often used in conjunction with other techniques in ambient conditions to provide real-time data on biofilm conditions.
5. Challenges and factors affecting electroactive biofilm formation
One of the most complicated issues in achieving high-power densities with electroactive biofilms is tackling the substrate's mass transfer limitations.69 Furthermore, conservation of viable bacterial cells in biofilms with effective wash out of cell debris harness the ability of electroactive biofilms. Research should be focussed on preservation methods for mixed cultures present in electroactive biofilms so that revival of the biofilm result in similar and reproducible results. Biofilm formation in microbial fuel cells is usually affected by several factors such as the electrode materials, reactor configuration, operating conditions, and biological parameters.70
5.1. The influence of MFC configuration on biofilm formation
The structural components of MFCs, such as electrode material, electrode spacing, membrane type, electrical circuit conductivity, working volume, etc., not only influence biofilm formation, but also the final MFCs performance. As a result, the majority of research focus on improving MFC architecture, and testing new materials.71,72
5.1.1. Effect of electrode materials on biofilm formation. The electrode material is critical in biofilm formation because it serves as the scaffold on which the biofilm grows and exchanges electrons to perform anodic respiration. The surface features of bioelectrodes, such as morphological structures, porosity, hydrophobicity, conductivity, charge, and bio-compatibility, depend mainly on the material used, and these properties have a direct impact on the microbial adhesion, and cell viability mechanism.70 The electrode's porosity contributes to an increase in the surface area, which creates more space for living microorganisms to attach and colonize. Electroactive microbes were found to be more selective towards the positively charged and hydrophilic electrode surfaces for the formation of conductive biofilms.73 Generally, bacteria have a net negative charge, therefore, positive charged electrodes are preferred since they provide a good electrostatic surface for negatively charged bacteria to attach to electrode surface. This attachment takes place via hydrogen bonding.73 Furthermore, electrode materials must be corrosion-resistant, have a high specific surface area and electrical conductivity, in addition to low electrical resistance and cost. The anode should be fabricated from a chemically stable material capable of operating in an environment where a wide range of organic and inorganic constituents are present. Those constituents may react with anode materials and result in poor MFC performance.74 Anode material should also be biocompatible to ensure viable growth and efficient electron transfer.75One of the previous reports commented on the effect of electrode nanostructures on the selective capture ability of exoelectrogens from wastewater samples for enhancing the formation of electrochemically active biofilms. Accordingly, the electrochemical activities of the matured biofilm formed on the MnO2 modified electrode confirmed the direct electron transfer, whereas the outer redox species were involved in the extracellular electron transfer.68
5.1.1.1. Conventional anode materials used in MFCs. In previous decades, numerous different materials have been investigated as anodes for MFCs. While the majority of previous research focused on the use of carbon-based materials and metal/metal oxides.68,76–78 Over years, these conventional materials were phased out in favor of more advanced materials that showed efficient MFC performance. The conventional carbon-based materials, shown in Fig. 4, include carbon paper (CP), carbon cloth (CC), graphite rod (GR), flexible graphite sheets (FGS), graphite felt (GF), graphite granules (GG), carbon brush (CB), reticulated glassy carbon (RGC), and activated carbon (AC).79–82
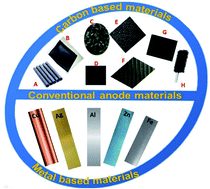 |
| Fig. 4 Some of the most conventional anode materials, in the upper half (A) graphite rods, (B) carbon cloth, (C) granular graphite, (D) carbon paper, (E) reticulated glassy carbon, (F) carbon mesh, (G) carbon felt, (H) carbon brush, and few metallic anode strips in the lower half. | |
Both packed and plane structural configurations, as well as brushes-based conventional anodes, have all been thoroughly reviewed.80 Low cost is one of the benefits of using carbon-based anode materials besides biocompatibility, good electrical conductivity, and excellent chemical stability.80 However, some aspects limit their applications in MFCs. For instance, the graphite-based materials have strong mechanical stability, but a weak biofilm formation on the anode surface, which has a direct negative impact on the MFC performance. The CP, CC, and RGC anode have a considerable rough surface area, which is required for biofilm formation nevertheless, all these are mechanically poor and result in inefficient operation over the long-term, and repeated life-cycles. In terms of microbial adhesion, the carbon felt (CF) showed excellent conductivity and flexibility. Unfortunately, the biofilm growth prevented the diffusion of organic substrate from the exterior to the interior surface, which is unfavorable for stable bacterial colonization. This is because of the high thickness surface of CF material. On other hand, using the CBs as anode enhanced the MFC performance, but they required metal wires to fix the carbon brushes, making them financially unsuitable. This has prompted researchers to develop modified anodes using CP, CF, or GR.77
In addition, carbon-based materials are known for their high hydrophobicity which does not favor microbial adhesion, this results in low electron transfer capacity.83 Electrochemical oxidation, chemical, and heat treatment can all be used to increase the surface area of an anode and therefore enhance the adhesion of microorganisms and the output power of the microbial fuel cell.84–87
Several types of metal/metal oxide nanoparticles (NPs) such as MnO2,68,88 titanium,81 gold,89,90 and copper91 have been contemplated for use as anode catalysts in MFCs. The idea of using different metals as MFC anode has failed due to several reasons such as cost, corrosion, and non-biocompatibility.92 For example, stainless steel mesh meets the required criteria of an ideal anode, but due to the gravity effect, biofilm vanishes over long-term use.93 Likewise, while Au, Ag, and Cu have excellent conductivity, they have poor operational stability when used to produce energy over long periods. As a result, modern anode materials must be integrated into the design while searching for feasible ways to obtain them in order to achieve satisfactory MFC efficiency.
5.1.1.2. Strategies of advanced anode materials. Advanced anode materials are further classified into two categories, each of which has shown their utility in both energy generation and wastewater treatment. Natural biomass-based carbon anode materials and composite-based materials, which comprise metal/metal oxides, carbon-based materials, and conducting polymers, are two subcategories of advanced anode materials.94 Advanced materials are expected to provide a low-cost alternative to traditional anode materials, with the added bonus of superior characteristics. The utility of advanced electrode materials used in MFCs has been the subject of numerous investigations. These advanced materials are described in the sub-sections that follow.
5.1.1.3. Natural anode electrodes. Recently, manufacturing of anode electrodes from natural materials, such as biomass wastes, has gained great interest recently due to various advantages, including the use of recyclable materials, material availability and material stability.95,96 Natural waste-derived materials are the most effective materials for manufacturing electrodes for MFCs because they are less expensive than traditional materials and are characterized with ideal electrode features. Moreover, the micro/mesoporous 3D structure of natural waste derived materials exhibited faster electron transfer and a good electro-kinetics mechanisms for electrochemical processes in MFCs.97 Manufactured 3D sponge was used as low-cost and high-performance anode material for MFC.98 Another example is the development of layered corrugated carbon (LCC)-based anode electrodes from low-cost materials via carbonization. As a result, increasing the number of layers improves current density performance by providing bacteria with a larger surface area for biofilm growth. In previous studies, LCC was used as a cost-effective and high-performance anode material in MFCs. LCC-based MFCs produced about 200 A m−2 and 390 A m−2 of current density by increasing the number of layers to three and six, respectively. Their work reported that LCC enhanced the current density compared to traditional graphite felt.96 Almond shells, waste paper, forestry residues, wood-derived wastes, chestnut shells, corn straw, and silk cocoon were among the used natural wastes for anode electrode fabrication. The abundance of biomass waste is dependent on availability in a specific geographical area, in Malaysia, for example, palm oil-based biomass is the most readily available biomass waste.99 However, several recyclable-waste materials have yet to be tested for anode fabrication. Furthermore, none of the previously developed anodes from natural wastes had a sufficient conductivity efficiency to meet the modern energy demand for MFCs.
5.1.1.4. Conductive polymers. Conducting polymers can be used as a sole or doping electrode materials for MFC operations due to their high conductivity and resistance to environmental conditions. The conductive polymer-modified anodes can be prepared by modification of electrode materials for enhanced bacterial cell attachment. Moreover, for improving anodic performance, the conducting polymer can be doped with nanomaterials forming a composite. Recently, many researches focused on the anode modifications with semiconductor polymers like polyaniline (PANI), polydopamine (PDA), and polypyrroles (PPy). These polymers increase capacitive properties, biocompatibility, and an active surface of the anode.100 When the CV method was used for doped electrochemically polymerized PANI onto graphite felt electrode,101 it was found that graphite felt-PANI decreased the start-up time, because the PANI-modified anode improved the bacterial cell adherence.Anodes modified with polymers or co-modification with other metal or carbon compounds, pure cultures of E. coli102 or Shewanella sp.103,104 are most often used as MFC exoelectrogens. There are few studies on the impact of anode modifications with polymers on the multispecies microbial community of anode. They showed that the modifications increased the number of Proteobacteria, Deltaproteobacteria, and the genus Geobacter.105,106 The presence of polydiallyldimethylammonium (PDDA) on the CF electrode accelerated the adherence of exoelectrogens to the surface through electrostatic attraction. Geobacter sp. and Pseudomonas sp. were about nine and three-fold higher, respectively, on PDDA modified CF anode than on the unmodified anode.106 Exoelectrogens from genera Acinetobacter, Brucella, and Bacillus exhibited 1.4 times lower adherence on PDDA modified CC anode than on the unmodified anode.107 Chen and Wang108 showed that E. coli cells grown in PDDA microcarriers had the same viability as those grown in suspension, as shown by an increase in optical density and cell number. However, Chlorella vulgaris cells showed extremely poor viability inside PDDA microcarriers, possibly due to blockage of nutrient uptake by the diallyldimethylammonium quaternary ammonium cation.109,110 At anodes modified with 50% PDA, an approximate two-fold increase in the percentage of Proteobacteria (up to 33%) and Firmicutes (up to 3%) biomass was observed compared to unmodified anode.111 Modification of the CC anode with PANI stimulated the participation of Geobacter sp. in the biofilm, while the simultaneous use of PDA with rGO on the CC anode caused that Geobacter sp. accounted for over 80% of the microorganisms identified in the biofilm. The anode modifications may act as a selective substrate for the growth of bacteria from the anolyte. Changes in the properties of the anode surface may also affect a transcriptomic profile of microorganisms in MFC; in the cells of microorganisms inhabiting the PDA/rGO modified anode, electrogenesis related to outer surface octaheme c-type cytochrome omcZ was highly expressed.112
5.1.1.5. Composite anode materials. Modification or implementation of nanostructured materials into or on traditional carbon structured electrode materials, such as 3D carbon black, carbon nanotubes (CNTs), porous carbon, graphene, and polyaniline (PANI), has also been highlighted as a potentially useful. Modification of anodes using metals or oxide-based nanocomposites influences the ohmic loss and result in improved bacterial cell attachment on the electrode surface. The newly fabricated anode, which consisted of a composite of iron (II, III) oxide (Fe3O4) and carbon nanotubes (CNT), produced a power density that reached up to 830 mW m−2. When Fe3O4 is attached to carbon nanotubes, it forms a multi-layered network that promotes bacterial growth and electron transfer.113 C/Hematite has been coated onto CC by the cost-effective and simple pyrolysis of ferrocene under atmospheric pressure. This procedure significantly improved the EET efficiency of S. oneidensis and has provided an approximately 6-fold higher current density in comparison to pristine CC anode in MFC.114 Recently, MnO2/MWCNTs nanocomposites were synthesized, characterized and utilized to support the growth of electrochemically active consortium of Enterobacter sp. Besides the formation of matured biofilm on its surface, MnO2/MWCNTs nanocomposite produced the highest electrical potential outputs (710 mV) combined with the highest power density (372 mW m−2), as shown in Fig. 5.78 Hydrothermal-assisted microwave dispersion and sonochemical synthesis were used to tin(IV) oxide (SnO2)-based monohybrids containing reduced graphene oxide (rGO) and carbon nanotubes (CNTs).115,116 During rGO/SnO2-CC based MFC operation, a power density of 1624 mW m−2 was achieved, which was 4.8-fold higher than that produced using a bare anode.116 The improved performance was primarily attributed to the increased specific surface area and higher biocompatibility of the hybrid composite which improve the bacterial adhesion and electron transfer efficiency.117,118
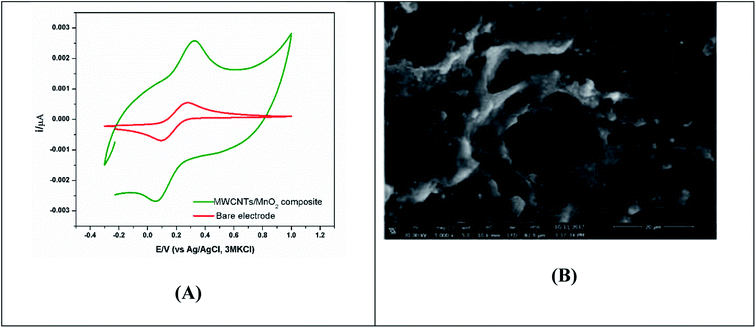 |
| Fig. 5 (A) Cyclic voltammetric characterizations of MnO2/MWCNTs nano-composite and bare electrode, (B) SEM images showing bioactive biofilm of Enterobacter sp. on the MnO2/MWCNTs nano-composite anode surface.78 | |
5.1.2. The effect of scale-up of MFC system on the biofilm formation. MFCs have been investigated at multiple scales varying from a few microliters to 1000.0 L.119–122 Numerous factors such as diffusion of substrates/bio-products, electron transfer rate and biofilm thickness are involved in the regulation of the electron flow from the living microbial cells to the electrode surface (and vice versa). All these factors are greatly influenced by the scale, and size of the MFC being used. It was formerly demonstrated that biofilm formation was more preferred in microhabitats than in other niches.123 This is due to the fact that miniature systems provide higher productivity with shorter start-up times and lower substrate consumption.124,125 Their main disadvantage is that they have relatively low current outputs, which hinders them from being used in industrial scale. Large-scale MFCs must be composed of highly electroactive species for applications such as wastewater treatment and bioremediation. At the moment, miniaturized and liter-scaled MFCs are being investigated in tandem to screen electroactive species for large-scale applications.
5.1.3. Effect of the type of membrane. In MFC system, the membrane governs the electro-neutrality between the two chambers, which permits the selective transfer of cations or anions via the anode and cathode. Proton exchange membranes (PEM) are the most commonly used membranes in MFCs because they allow protons to be transferred from the anode to the cathode.126 It was found that the type and surface area of the membrane had a significant impact on the power generation on MFCs.127 This is because the type of membrane used determines the type of ions transferred, which has a direct impact on the pH of the solution. For instance, when Nafion 117 was used, it was noticed that instead of proton pumping, other cations (such as Na+, K+, Ca2+, and Mg2+) present in the anolyte were transferred to the cathode, causing an increase in pH at the cathode and a subsequent reduction in pH at the anode. This pH variation affected the growth and community structure of the anodic biofilms.128
5.1.4. Membrane biofouling and cost. Problems facing PEM use in MFC are biofouling and expensive cost. Biofouling of the membrane is an unwanted attachment of bacterial cells adhering to the membrane surface. It takes place initially via adhesion of organic or biological materials to the membrane and results in hindering proton transfer and increasing ohmic and charge transfer resistance. Strategies to prevent biofouling focus on: (1) preparation of antifouling composite membrane, (2) changing physical and chemical properties of existing membranes or (3) coating the membrane with anti-fouling agents.129 The main idea about preventing adhesion is to control the roughness, hydrophilicity and charge on the membrane surface. In terms of structure, a model membrane would be one that is smooth, negatively charged and highly hydrophilic.130 Several studies have reported on the anti-biofouling, for example, pre-treatment of Nafion 117 with 3% hydrogen peroxide and 0.5 M sulfuric acid resulted in less biofouling, and increased the power density to 186.7 mA W−2 as compared to only 20.9 mA W−2.131 Polyvinyl alcohol (PVA) has been reported as a suitable material for a smooth surface membrane, adding light expanded clay aggregates to the hydrophilic polyvinyl alcohol hydrogel (PEM-PVA-H-clay) has led to an increase in the proton conductivity with 2.87 fold as compared to that lacking clay.132 The addition of hydrophilic polydopamine coating on the membrane surface led to a reduction in the membrane biofouling with an increase in the direct electric current.133 While adding silver nanoparticles to polydopamine prevented PEM fouling due to its antimicrobial nature.134 Affordable PEM is also considered a bottleneck, a low cost activated carbon derived from coconut shell and blended with natural clay enhanced PEM hydration property and proton transfer which led to an increase in the coulombic efficiency of the MFC.135 In another report, ceramic membrane modified with rice husk ash exhibited high proton transfer and low oxygen diffusion,136 while sulfonated biochar-PVA composite showed an increase in the proton conductivity (32 fold) compared to Nafion membranes.137
5.2. Operation condition
During MFC operation, different physicochemical parameters may affect biofilm formation, and the overall performance of MFC. These parameters include pH, temperature, substrate, and ion concentration. This can be attributed to both bacterial and material surfaces being influenced by their surrounding environmental conditions.138,139
5.2.1. Effect of pH. Effect of anodic pH on the performance of MFCs has been the main focus of many researchers.140–143 It was observed that the optimum pH for most MFC designs was restricted to neutral7 or near-neutral6–8 ranges. However, the operation of MFCs at extreme pH conditions was seldom reported.142,144 Though several factors (such as ion transfer, substrate oxidation, and oxygen reduction) are pH dependent during MFC operation, their effect can be circumvented by using appropriate catalysts, novel ion exchange membranes, and addition of buffering agents. At the same time, pH of the electrolyte has a direct influence on the growth and development of bacterial communities and their viability.145 This is because microorganisms are sensitive to their surrounding medium, and even the slightest variation in pH can cause alterations in cellular metabolism. However, unlike enzymatic reactions, bacteria can adapt themselves to varying external pH to carry out their metabolic reactions. Several physiological parameters such as the membrane potential, proton motive force, cytoplasmic, and electrochemical gradient are affected by the pH of the microenvironment and they ultimately influence the net production and consumption of protons.145 During MFC operation, especially when wastewater is used as a substrate, microorganisms are exposed to varying pH conditions at different time intervals due to the charge transfer between the anode and cathode.141 This change in pH did not only affect the power outputs of MFCs, but also led to a change in the microbial community.145 Apart from power outputs, due to the pH changes, the activity of anode-formed biofilm was inhibited, and thus the efficiency of MFCs for wastewater treatment was inhibited. Therefore, it is essential that the pH of the system is monitored periodically and maintained to obtain high power as well as high bio-degradation performance. Furthermore, sustaining an optimum pH for bacterial growth improves the robustness and longevity of biofilms. However, operating MFCs at constant pH by the addition of acid/alkali increases the cost of the process, which is undesirable. Thus, most studies were focused on maintaining the pH via the addition of a suitable buffer146 or via the use of anion exchange membranes.147 Though these studies have elaborated the role of pH during biofilm formation, further investigation is required to understand its effect on the microbial community structure and their interactions.
5.2.2. Effect of temperature. Microbial biofilms are sensitive to temperature to optimize their growth and electrocatalytic activity.148,149 Depending on the temperature range in which they grow, bacteria can be divided into three groups, psychrophiles (<15 °C), mesophiles (15–40 °C), and thermophiles (>40 °C). Most of the MFC designs have been performed in mesophilic ranges,147,150,151 which suggest that most of the electroactive bacteria are functioning in mesophilic temperatures. However, certain psychrophiles and thermophiles have also been reported to be electroactive in nature.151 In MFCs with mixed cultures, temperature determines the nature and distribution of microbial communities as each species present in the consortia will have different optimum temperatures for growth.150 Furthermore, temperature variation leads to change in the kinetics and thermodynamics of the anodic reactions, which indirectly affect the growth of biofilms.150
5.2.3. Effect of substrate concentration. The nature of the substrate and its concentration in the growth medium influence the dominance of one species over the other in a mixed microbial community.152,153 Different substrates, electron donors, ranging from simple sugars to complex carbohydrates, artificial, real wastewater, lignocellulosic biomass, and some of inorganic substrates, such as sulfide have been used in MFCs.154 A prominent shift in the prevailing dominant microbial community was observed with a change in the substrate concentration.155 This is due to the presence of different microorganisms rather than the electroactive bacteria present in the mixed consortia, which compete for the substrate consumption to gain energy. The substrate concentration, on the other hand, influences the power generated from the MFC system. In general, it was observed that high substrate concentration caused damaging effects to the biofilm. To achieve high coulombic efficiency, all electrons released from substrate oxidation should be channeled toward electricity generation. However, some of these electrons are lost, lowering the efficiency of the system. These intermediate compounds, if toxic to the growth of bacteria, can severely affect biofilm formation and current generation.
5.3. The biological parameters affecting biofilm formation
The biological factors that influence biofilm formation include the source and nature of inoculum, and growth rate. The majority of electroactive microbes tested to date have been found to be Gram-negative in nature.156–158 The change in cell wall composition between Gram-negative and Gram-positive bacteria is thought to cause a variation in cell surface charge, which influences the bacteria's electrogenic activity.159 Gram-positive bacteria have a peptidoglycan layer linked to teichoic acid, which gives them a high zeta potential, whereas Gram-negative bacteria have lipopolysaccharides, which give them a lower zeta potential than Gram-positive bacteria. As previously described, these bacterial surface charges interact with charges on the electrode surfaces, and thus is critical in deciding the sort of material to be utilized in the MFC system. Furthermore, bacterial biofilms can be created by a pure culture or a mixed culture.160 Consequently, the bigger the number of electroactive species present, the faster the substrate degrades and the more protons and electrons are produced. The outputs of MFCs can be significantly increased if all electrons released during substrate degradation are redirected for energy generation. When compared to pure culture biofilms, mixed culture biofilms were found to yield a higher flow of electrons.161 However, the bacterial community present in the mixed culture is inconsistent; making the reproducibility of such biofilm is debatable. The source of the bacterial inoculum is also important in determining the current biofilm's composition. Electroactive microorganisms are abundant in nature and can be found in a variety of environments.162 Therefore, the bacterial population that prevails in an MFC is determined by the source of the inoculum. It was found that mixed cultures use wastewater, anaerobic sludge, or river/marine sediments as the main source of MFC inoculum.163 These mixed cultures are usually favored when the main use is wastewater treatment. But, pure cultures (such as Clostridium sp.) have been used for selective applications, such as BOD biosensors, to achieve batch-to-batch repeatability of the signal output.164
6. Challenges of cathodic oxygen reduction reaction (ORR)
At the cathodic chamber, oxygen is commonly used as the final electron acceptor because of its high oxidation potential. Many studies reported that the oxygen supply to the cathode compartment is energy consuming.165 While the atmospheric oxygen can be used directly by using an air cathode, contact difficulties in the cathode-air surface and the necessity for expensive catalysts are the disadvantages of oxygen utilization.166 The development of low-cost, high-performance nanocatalysts for the intrinsically slow oxygen reduction process (ORR) is a major roadblock in the commercialization of fuel cells for energy conversion. Recently, a lot of research has been directed towards developing Pd-based nanocatalysts with improved stability to use as Pt alternatives.167 Over the years, a wide range of investigations have been focused on the utility of Pd-based alloys during oxygen reduction. For example, Liu et al. developed a surfactant-based synthetic technique to synthesize Pd–Ni nanowires with uniform metallic elements dispersion.168 A previous study showed that the power density of Pt/rGO cathode based MFC was reduced by 5.8% compared to Pt/C cathode. Even though, the power densities for non-Pt cathode catalysts was reduced to 26.4%, 31.1%, and 48.2% for MnO2/rGO, rGO, and MnO2, respectively. Generally, the use of MFCs equipped with the Pt/rGO cathode, Pt/rGO was found to be a relatively cheaper catalyst that can be produced using a facile preparation method in MFC applications. This approach that shown in Fig. 6 should be encouraged, while the voltammetric technique is very efficient in the assessment or screening of new materials that might show catalytic activities towards the ORR.165
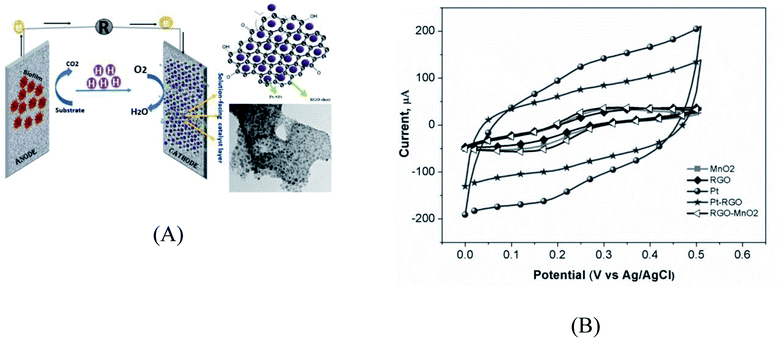 |
| Fig. 6 (A) Schematic representation showing the effect of nanocomposite on the ORR at the cathodic side. (B) Electrochemical catalytic activities of modified electrodes with the prepared nanostructures toward the reduction of dissolved oxygen in the voltammetric cells.165 | |
7. MFC scaling up issues
In order to treat large volumes of wastewater, and generate high current density, scaling up the MFC is essential. It has been demonstrated that as the dimension/configuration of MFC is enlarged, the power density decreases owing to the volumetric ohmic resistance increase and inactive reactor volume, resulting in low MFC power production performance.169 Integrating/collecting multiple small MFC systems to build a large stack for power generation is more realistic and efficient way to scale-up MFC systems than simply increasing the size of each reactor.170 So far, there have been a number of studies that have investigated at the performance of a scale-up MFC stack.171,172 Unfortunately, in some studies the reported power densities were still too low to make the MFC system comparable to traditional anaerobic treatment in terms of energy recovery. For instance Dekker et al.173 fabricated a 20 L stacked MFC fed by synthetic wastewater but due to the reversal voltage in some cell units under series electrical connection, the produced power density was very low and didn't exceed 11 W m−3. Hence, to improve the power output of MFC, the internal resistance which primarily includes kinetic, ohmic, and transportation resistance should be decreased as low as possible.174
8. Concluding remarks and future perspectives
MFCs are a novel suitable, eco-friendly alternative to produce energy through the oxidation of a variety of substrates; however, there are some challenges that need to be addressed to make the technology economically sound. The main prime hurdle is the active biofilm formation which is a critical key for MFC performance. Hence, it is important to understand electroactive biofilms in detail, the electron transfer mechanisms, and the factors affecting biofilm formation. In addition, this review article emphasizes on the importance of a feasible design for MFC scale-up. The majority of designs exhibit drawbacks such as high internal resistance, electrode spacing, exchange of anolyte and catholyte across PEM for scaling-up and long-term operation. Another challenge is to provide cost-effective electrode materials and PEM (if used) for MFCs. In previous decades, many conventional anode materials were introduced, but they have failed to meet the modern demands. An ideal anode would provide basic conductive properties and good microbe-electrode interface that lead to higher electron transfer rates and hence, high MFC performance. Among all the materials, the natural resources and their composite are good materials to fulfill the modern requirements while minimizing other disadvantages. One more obstacle is the choice of electroactive microbes because microbial surface charges interact with charges on the electrode surfaces, and thus is critical in deciding the type of material to be utilized in the MFC system. Indeed, more efforts are required to overcome these difficulties and enhance the overall performance of MFCs prior to industrial scale.
On the other hand, oxygen reduction electrocatalysis is very important for many applications including the microbial fuel cells. However, the cathodic oxygen reduction reaction requires the development of effective, and high performance electrocatalysts to facilitate power output in MFCs. Due to the high cost, replacing noble metal-based electrocatalysts with highly efficient and inexpensive nanomaterials for ORRs is very crucial for the practical application of these technologies. This constrain has been covered in a section of this review.
Conflicts of interest
There are no conflicts to declare.
References
- C. Santoro, C. Arbizzani, B. Erable and I. Ieropoulos, Microbial fuel cells: from fundamentals to applications. A review, J. Power Sources, 2017, 356, 225–244 CrossRef CAS PubMed.
- K. Rabaey, K. Van de Sompel, L. Maignien, N. Boon, P. Aelterman and P. Clauwaert, et al., Microbial fuel cells for sulfide removal, Environ. Sci. Technol., 2006, 40(17), 5218–5224 CrossRef CAS PubMed.
- M. C. Potter, Electrical effects accompanying the decomposition of organic compounds, Proc. R. Soc. London, Ser. B, 1911, 84(571), 260–276 Search PubMed.
- P. Aelterman, K. Rabaey, H. T. Pham, N. Boon and W. Verstraete, Continuous electricity generation at high voltages and currents using stacked microbial fuel cells, Environ. Sci. Technol., 2006, 40(10), 3388–3394 CrossRef CAS PubMed.
- M. Behera, P. S. Jana and M. M. Ghangrekar, Performance evaluation of low cost microbial fuel cell fabricated using earthen pot with biotic and abiotic cathode, Bioresour. Technol., 2010, 101(4), 1183–1189 CrossRef CAS PubMed.
- K. MacVittie, J. Halámek, L. Halámková, M. Southcott, W. D. Jemison and R. Lobel, et al., From “cyborg” lobsters to a pacemaker powered by implantable biofuel cells, Energy Environ. Sci., 2013, 6(1), 81–86 RSC.
- F. Zhang, Z. Ge, J. Grimaud, J. Hurst and Z. He, Long-term performance of liter-scale microbial fuel cells treating primary effluent installed in a municipal wastewater treatment facility, Environ. Sci. Technol., 2013, 47(9), 4941–4948 CrossRef CAS PubMed.
- H. H. Salman and Z. Z. Ismail, Modeling and simulation of the processes in desalination fuel cell fed with actual wetland brackish water, J. Appl. Electrochem., 2021, 51(6), 905–916 CrossRef CAS.
- M. Sogani, A. O. Pankan, A. Dongre, K. Yunus and A. C. Fisher, Augmenting the biodegradation of recalcitrant ethinylestradiol using Rhodopseudomonas palustris in a hybrid photo-assisted microbial fuel cell with enhanced bio-hydrogen production, J. Hazard. Mater., 2021, 408, 124421 CrossRef CAS PubMed.
- A. Adekunle, C. Rickwood and B. Tartakovsky, On-line monitoring of water quality with a floating microbial fuel cell biosensor: field test results, Ecotoxicology, 2021, 5, 851–862 CrossRef PubMed.
- H. Wang, J.-D. Park and Z. J. Ren, Practical energy harvesting for microbial fuel cells: a review, Environ. Sci. Technol., 2015, 49(6), 3267–3277 CrossRef CAS.
- K. Dutta and P. P. Kundu, A review on aromatic conducting polymers-based catalyst supporting matrices for application in microbial fuel cells, Polym. Rev., 2014, 54(3), 401–435 CrossRef CAS.
- H. M. M. Selim, A. M. Kamal, D. M. M. Ali and R. Y. A. Hassan, Bioelectrochemical Systems for Measuring Microbial Cellular Functions, Electroanalysis, 2017, 29(6), 1498–1505 CrossRef CAS.
- K. Dolch, J. Danzer, T. Kabbeck, B. Bierer, J. Erben and A. H. Förster, et al., Characterization of microbial current production as a function of microbe–electrode-interaction, Bioresour. Technol., 2014, 157, 284–292 CrossRef CAS.
- U. Schröder, Anodic electron transfer mechanisms in microbial fuel cells and their energy efficiency, Phys. Chem. Chem. Phys., 2007, 9(21), 2619–2629 RSC.
- Y. Yang, M. Xu, J. Guo and G. Sun, Bacterial extracellular electron transfer in bioelectrochemical systems, Process Biochem., 2012, 47(12), 1707–1714 CrossRef CAS.
- R. Kumar, L. Singh and A. W. Zularisam, Exoelectrogens: recent advances in molecular drivers involved in extracellular electron transfer and strategies used to improve it for microbial fuel cell applications, Renewable Sustainable Energy Rev., 2016, 56, 1322–1336 CrossRef CAS.
- L. Shi, H. Dong, G. Reguera, H. Beyenal, A. Lu and J. Liu, et al., Extracellular electron transfer mechanisms between microorganisms and minerals, Nat. Rev. Microbiol., 2016, 14(10), 651–662 CrossRef CAS PubMed.
- A. A. Carmona-Martinez, F. Harnisch, L. A. Fitzgerald, J. C. Biffinger, B. R. Ringeisen and U. Schröder, Cyclic voltammetric analysis of the electron transfer of Shewanella oneidensis MR-1 and nanofilament and cytochrome knock-out mutants, Bioelectrochemistry, 2011, 81(2), 74–80 CrossRef CAS PubMed.
- A. A. Carmona-Martínez, F. Harnisch, U. Kuhlicke, T. R. Neu and U. Schröder, Electron transfer and biofilm formation of Shewanella putrefaciens as function of anode potential, Bioelectrochemistry, 2013, 93, 23–29 CrossRef PubMed.
- S. Xu, A. Barrozo, L. M. Tender, A. I. Krylov and M. Y. El-Naggar, Multiheme cytochrome mediated redox conduction through Shewanella oneidensis MR-1 cells, J. Am. Chem. Soc., 2018, 140(32), 10085–10089 CrossRef CAS PubMed.
- P. Subramanian, S. Pirbadian, M. Y. El-Naggar and G. J. Jensen, Ultrastructure of Shewanella oneidensis MR-1 nanowires revealed by electron cryotomography, Proc. Natl. Acad. Sci. U. S. A., 2018, 115(14), E3246–E3255 CrossRef CAS PubMed.
- S. Sure, M. L. Ackland, A. A. J. Torriero, A. Adholeya and M. Kochar, Microbial nanowires: an electrifying tale, Microbiology, 2016, 162(12), 2017–2028 CrossRef CAS PubMed.
- D. R. Lovley, Happy together: microbial communities that hook up to swap electrons, ISME J., 2017, 11(2), 327–336 CrossRef CAS PubMed.
- S. Pirbadian, S. E. Barchinger, K. M. Leung, H. S. Byun, Y. Jangir and R. A. Bouhenni, et al., Shewanella oneidensis MR-1 nanowires are outer membrane and periplasmic extensions of the extracellular electron transport components, Proc. Natl. Acad. Sci. U. S. A., 2014, 111(35), 12883–12888 CrossRef CAS PubMed.
- K. M. Leung, G. Wanger, M. Y. El-Naggar, Y. Gorby, G. Southam and W. M. Lau, et al., Shewanella oneidensis MR-1 bacterial nanowires exhibit p-type, tunable electronic behavior, Nano Lett., 2013, 13(6), 2407–2411 CrossRef CAS PubMed.
- N. S. Malvankar and D. R. Lovley, Microbial nanowires for bioenergy applications, Curr. Opin. Biotechnol., 2014, 27, 88–95 CrossRef CAS PubMed.
- G. Reguera, K. D. McCarthy, T. Mehta, J. S. Nicoll, M. T. Tuominen and D. R. Lovley, Extracellular electron transfer via microbial nanowires, Nature, 2005, 435(7045), 1098–1101 CrossRef CAS PubMed.
- N. S. Malvankar and D. R. Lovley, Microbial nanowires: a new paradigm for biological electron transfer and bioelectronics, ChemSusChem, 2012, 5(6), 1039–1046 CrossRef CAS PubMed.
- R. Y. A. Hassan, M. M. Mekawy, P. Ramnani and A. Mulchandani, Monitoring of microbial cell viability using nanostructured electrodes modified with graphene/alumina nanocomposite, Biosens. Bioelectron., 2017, 91, 857–862 CrossRef CAS.
- R. Y. A. Hassan, F. Febbraio and S. Andreescu, Microbial Electrochemical Systems: Principles, Construction and Biosensing Applications, Sensors, 2021, 21, 1279–1298 CrossRef CAS.
- R. H. Mahmoud, S. M. Abdo, F. A. Samhan, M. K. Ibrahim, G. H. Ali and R. Y. A. Hassan, Biosensing of algal-photosynthetic productivity using nanostructured bioelectrochemical systems, J. Chem. Technol. Biotechnol., 2020, 95(4), 1028–1037 CAS.
- C. A. Hernandez and J. F. Osma, Microbial electrochemical systems: deriving future trends from historical perspectives and characterization strategies, Front. Environ. Sci., 2020 DOI:10.3389/fenvs.2020.00044.
- G. Pankratova and L. Gorton, Electrochemical communication between living cells and conductive surfaces, Curr. Opin. Electrochem., 2017, 5(1), 193–202 CrossRef CAS.
- D. R. Lovley, Bug juice: harvesting electricity with microorganisms, Nat. Rev. Microbiol., 2006, 4(7), 497–508 CrossRef CAS PubMed.
- R. Fathey, O. M. Gomaa, A. E.-H. Ali, H. A. El Kareem and M. A. Zaid, Neutral red as a mediator for the enhancement of electricity production using a domestic wastewater double chamber microbial
fuel cell, Ann. Microbiol., 2016, 66(2), 695–702 CrossRef CAS.
- C. Xu, K. Poon, M. M. F. Choi and R. Wang, Using live algae at the anode of a microbial fuel cell to generate electricity, 2015 Search PubMed.
- K. Hasan, K. V. R. Reddy, V. Eßmann, K. Górecki, P. Ó. Conghaile and W. Schuhmann, et al., Electrochemical communication between electrodes and Rhodobacter capsulatus grown in different metabolic modes, Electroanalysis, 2015, 27(1), 118–127 CrossRef CAS.
- C. F. Spégel, A. R. Heiskanen, N. Kostesha, T. H. Johanson, M.-F. Gorwa-Grauslund and M. Koudelka-Hep, et al., Amperometric response from the glycolytic versus the pentose phosphate pathway in Saccharomyces cerevisiae cells, Anal. Chem., 2007, 79(23), 8919–8926 CrossRef.
- K. Baronian, A. Downard, R. Lowen and N. Pasco, Detection of two distinct substrate-dependent catabolic responses in yeast cells using a mediated electrochemical method, Appl. Microbiol. Biotechnol., 2002, 60(1), 108–113 CAS.
- V. Chelikani, A. J. Downard, G. Kunze, R. Gooneratne, N. Pasco and K. H. R. Baronian, Investigating yeast cell responses to oestrogen by electrochemical detection, Electrochim. Acta, 2012, 73, 136–140 CrossRef CAS.
- H. Nakamura, K. Suzuki, H. Ishikuro, S. Kinoshita, R. Koizumi and S. Okuma, et al., A new BOD estimation method employing a double-mediator system by ferricyanide and menadione using the eukaryote Saccharomyces cerevisiae, Talanta, 2007, 72(1), 210–216 CrossRef CAS PubMed.
- I. Vostiar, E. E. Ferapontova and L. Gorton, Electrical “wiring” of viable Gluconobacter oxydans cells with a flexible osmium-redox polyelectrolyte, Electrochem. Commun., 2004, 6(7), 621–626 CrossRef CAS.
- J. R. Beegle and A. P. Borole, Chapter 11 – Exoelectrogens for Microbial Fuel Cells☆, in MFC, ed. P. P. Kundu and K. Dutta, Elsevier, 2018, pp. 193–230 Search PubMed.
- A. P. Borole, G. Reguera, B. Ringeisen, Z.-W. Wang, Y. Feng and B. H. Kim, Electroactive biofilms: current status and future research needs, Energy Environ. Sci., 2011, 4(12), 4813–4834 RSC.
- K. Rabaey, N. Boon, M. Höfte and W. Verstraete, Microbial phenazine production enhances electron transfer in biofuel cells, Environ. Sci. Technol., 2005, 39(9), 3401–3408 CrossRef CAS PubMed.
- H. von Canstein, J. Ogawa, S. Shimizu and J. R. Lloyd, Secretion of Flavins by Shewanella Species and Their Role in Extracellular Electron Transfer, Appl. Environ. Microbiol., 2008, 74(3), 615–623 CrossRef CAS PubMed.
- K. P. Nevin and D. R. Lovley, Mechanisms for Fe(III) Oxide Reduction in Sedimentary Environments, Geomicrobiol. J., 2002, 19(2), 141–159 CrossRef CAS.
- K. Rosso, J. Zachara, J. Fredrickson, Y. Gorby and S. Smith, Nonlocal bacterial electron transfer to hematite, Geochim. Cosmochim. Acta, 2003, 67, 1081–1087 CrossRef CAS.
- E. Marsili, D. B. Baron, I. D. Shikhare, D. Coursolle, J. A. Gralnick and D. R. Bond, Shewanella secretes flavins that mediate extracellular electron transfer, Proc. Natl. Acad. Sci. U. S. A., 2008, 105(10), 3968–3973 CrossRef CAS PubMed.
- N. J. Kotloski and J. A. Gralnick, Flavin Electron Shuttles Dominate Extracellular Electron Transfer by Shewanella oneidensis, mBio, 2013, 4(1), e00553-12 CrossRef PubMed.
- M. A. TerAvest, M. A. Rosenbaum, N. J. Kotloski, J. A. Gralnick and L. T. Angenent, Oxygen allows Shewanella oneidensis MR-1 to overcome mediator washout in a continuously fed bioelectrochemical system, Biotechnol. Bioeng., 2014, 111(4), 692–699 CrossRef CAS PubMed.
- K. Rabaey, N. Boon, S. D. Siciliano, M. Verhaege and W. Verstraete, Biofuel cells select for microbial consortia that self-mediate electron transfer, Appl. Environ. Microbiol., 2004, 70(9), 5373–5382 CrossRef CAS PubMed.
- M. E. Hernandez and D. K. Newman, Extracellular electron transfer, Cell. Mol. Life Sci., 2001, 58(11), 1562–1571 CrossRef CAS PubMed.
- C. D. Cox, Role of pyocyanin in the acquisition of iron from transferrin, Infect. Immun., 1986, 52(1), 263–270 CrossRef CAS PubMed.
- A. E. Franks, N. Malvankar and K. P. Nevin, Bacterial biofilms: the powerhouse of a microbial fuel cell, Biofuels, 2010, 1(4), 589–604 CrossRef CAS.
- J. Babauta, R. Renslow, Z. Lewandowski and H. Beyenal, Electrochemically active biofilms: facts and fiction. A review, Biofouling, 2012, 28, 789–812 CrossRef CAS PubMed.
- I. Schmidt, A. Gad, G. Scholz, H. Boht, M. Martens and M. Schilling, et al., Gold-modified indium tin oxide as a transparent window in optoelectronic diagnostics of electrochemically active biofilms, Biosens. Bioelectron., 2017, 94, 74–80 CrossRef CAS PubMed.
- S. Kalathil, M. M. Khan, J. Lee and M. H. Cho, Production of bioelectricity, bio-hydrogen, high value chemicals and bioinspired nanomaterials by electrochemically active biofilms, Biotechnol. Adv., 2013, 31(6), 915–924 CrossRef CAS PubMed.
- F. Harnisch and K. Rabaey, The Diversity of Techniques to Study Electrochemically Active Biofilms Highlights the Need for Standardization, ChemSusChem, 2012, 5(6), 1027–1038 CrossRef CAS PubMed.
- H. S. Magar, R. Y. A. Hassan and A. Mulchandani, Electrochemical Impedance Spectroscopy (EIS): Principles, Construction, and Biosensing Applications, Sensors, 2021, 21(19), 6578 CrossRef CAS PubMed.
- B. H. Kim, T. Ikeda, H. S. Park, H. J. Kim, M. S. Hyun and K. Kano, et al., Electrochemical activity of an Fe(III)-reducing bacterium, Shewanella putrefaciens IR-1, in the presence of alternative electron acceptors, Biotechnol. Tech., 1999, 13(7), 475–478 CrossRef CAS.
- Y. Dai, T. Sun, Z. Zhang, Z. J. Zhang and J. Li, Effect of zinc oxide film morphologies on the formation of Shewanella putrefaciens biofilm, Biointerphases, 2017, 12(1), 11002 CrossRef.
- M. D. Blakeney, T. Moulaei and T. J. Dichristina, Fe(III) reduction activity and cytochrome content of Shewanella putrefaciens grown on ten compounds as sole terminal electron acceptor, Microbiol. Res., 2000, 155(2), 87–94 CrossRef CAS.
- C. Hung, Y. Zhou, J. S. Pinkner, K. W. Dodson, J. R. Crowley and J. Heuser, et al., Escherichia coli Biofilms Have an Organized and Complex Extracellular Matrix Structure, mBio, 2013, 4(5), e00645-13 CrossRef PubMed.
- C. Hannig, M. Follo, E. Hellwig and A. Al-Ahmad, Visualization of adherent micro-organisms using different techniques, J. Med. Microbiol., 2009, 59, 1–7 CrossRef PubMed.
- M. Alhede, K. Qvortrup, R. Liebrechts, N. Høiby, M. Givskov and T. Bjarnsholt, Combination of microscopic techniques reveals a comprehensive visual impression of biofilm structure and composition, FEMS Immunol. Med. Microbiol., 2012, 65(2), 335–342 CrossRef CAS PubMed.
- R. H. Mahmoud, F. A. Samhan, G. H. Ali, M. K. Ibrahim and R. Y. A. Hassan, Assisting the biofilm formation of exoelectrogens using nanostructured microbial fuel cells, J. Electroanal. Chem., 2018, 824, 128–135 CrossRef CAS.
- J. T. Babauta and H. Beyenal, Mass transfer studies of Geobacter sulfurreducens biofilms on rotating disk electrodes, Biotechnol. Bioeng., 2014, 111(2), 285–294 CrossRef CAS PubMed.
- G. D. Saratale, R. G. Saratale, M. K. Shahid, G. Zhen, G. Kumar and H.-S. Shin, et al., A comprehensive overview on electro-active biofilms, role of exo-electrogens and their microbial niches in microbial fuel cells (MFCs), Chemosphere, 2017, 178, 534–547 CrossRef CAS PubMed.
- M. Di Lorenzo, K. Scott, T. P. Curtis and I. M. Head, Effect of increasing anode surface area on the performance of a single chamber microbial fuel cell, Chem. Eng. J., 2010, 156(1), 40–48 CrossRef CAS.
- L. Pons, M.-L. Délia and A. Bergel, Effect of surface roughness, biofilm coverage and biofilm structure on the electrochemical efficiency of microbial cathodes, Bioresour. Technol., 2011, 102(3), 2678–2683 CrossRef CAS PubMed.
- R. Veerubhotla, J. L. Varanasi and D. Das, Chapter 12 – Biofilm Formation within Microbial Fuel Cells, in MFC, ed. P. P. Kundu and K. Dutta, Elsevier, 2018, pp. 231–42 Search PubMed.
- M. Zhou, M. Chi, J. Luo, H. He and T. Jin, An overview of electrode materials in microbial fuel cells, J. Power Sources, 2011, 196(10), 4427–4435 CrossRef CAS.
- S. Narayanasamy and J. Jayaprakash, Application of carbon-polymer based composite electrodes for microbial fuel cells, Rev. Environ. Sci. Bio/Technol., 2020, 1–26 Search PubMed.
- D. Nosek, P. Jachimowicz and A. Cydzik-Kwiatkowska, Anode Modification as an Alternative Approach to Improve Electricity Generation in Microbial Fuel Cells, Energies, 2020, 13(24), 6596 CrossRef CAS.
- J. M. Sonawane, A. Yadav, P. C. Ghosh and S. B. Adeloju, Recent advances in the development and utilization of modern anode materials for high performance microbial fuel cells, Biosens. Bioelectron., 2017, 90, 558–576 CrossRef CAS PubMed.
- R. H. Mahmoud, F. A. Samhan, M. K. Ibrahim, G. H. Ali and R. Y. A. Hassan, Formation of electroactive biofilms derived by nanostructured anodes surfaces, Bioprocess Biosyst. Eng., 2021, 759–768 CrossRef CAS PubMed.
- F. Li, Y. Sharma, Y. Lei, B. Li and Q. Zhou, Microbial fuel cells: the effects of configurations, electrolyte solutions, and electrode materials on power generation, Appl. Biochem. Biotechnol., 2010, 160(1), 168 CrossRef CAS PubMed.
- J. Wei, P. Liang and X. Huang, Recent progress in electrodes for microbial fuel cells, Bioresour. Technol., 2011, 102(20), 9335–9344 CrossRef CAS PubMed.
- A. Ter Heijne, H. V. M. Hamelers, M. Saakes and C. J. N. Buisman, Performance of non-porous graphite and titanium-based anodes in microbial fuel cells, Electrochim. Acta, 2008, 53(18), 5697–5703 CrossRef CAS.
- X. Wang, S. Cheng, Y. Feng, M. D. Merrill, T. Saito and B. E. Logan, Use of carbon mesh anodes and the effect of different pretreatment methods on power production in microbial fuel cells, Environ. Sci. Technol., 2009, 43(17), 6870–6874 CrossRef CAS.
- Y. Hindatu, M. S. M. Annuar and A. M. Gumel, Mini-review: anode modification for improved performance of microbial fuel cell, Renewable Sustainable Energy Rev., 2017, 73, 236–248 CrossRef CAS.
- T. Kou, Y. Yang, B. Yao and Y. Li, Interpenetrated Bacteria-Carbon Nanotubes Film for Microbial Fuel Cells, Small Methods, 2018, 2(10), 1800152 CrossRef.
- I. Bruch, J. Fritsche, D. Bänninger, U. Alewell, M. Sendelov and H. Hürlimann, et al., Improving the treatment efficiency of constructed wetlands with zeolite-containing filter sands, Bioresour. Technol., 2011, 102(2), 937–941 CrossRef CAS PubMed.
- M. Zhou, M. Chi, H. Wang and T. Jin, Anode modification by electrochemical oxidation: a new practical method to improve the performance of microbial fuel cells, Biochem. Eng. J., 2012, 60, 151–155 CrossRef CAS.
- K. Scott, G. A. Rimbu, K. P. Katuri, K. K. Prasad and I. M. Head, Application of Modified Carbon Anodes in Microbial Fuel Cells, Process Saf. Environ. Prot., 2007, 85(5), 481–488 CrossRef CAS.
- S. Phonsa, P. Sreearunothai, S. Charojrochkul and K. Sombatmankhong, Electrodeposition of MnO2 on polypyrrole-coated stainless steel to enhance electrochemical activities in microbial fuel cells, Solid State Ionics, 2018, 316, 125–134 CrossRef CAS.
- S. R. Crittenden, C. J. Sund and J. J. Sumner, Mediating electron transfer from bacteria to a gold electrode via a self-assembled monolayer, Langmuir, 2006, 22(23), 9473–9476 CrossRef CAS PubMed.
- H. Richter, K. McCarthy, K. P. Nevin, J. P. Johnson, V. M. Rotello and D. R. Lovley, Electricity generation by Geobacter sulfurreducens attached to gold electrodes, Langmuir, 2008, 24(8), 4376–4379 CrossRef CAS PubMed.
- X. Zhu and B. E. Logan, Copper anode corrosion affects power generation in microbial fuel cells, J. Chem. Technol. Biotechnol., 2014, 89(3), 471–474 CrossRef CAS.
- E. Taşkan, S. Bulak, B. Taşkan, M. Şaşmaz, S. El Abed and A. El Abed, Nitinol as a suitable anode material for electricity generation in microbial fuel cells, Bioelectrochemistry, 2019, 128, 118–125 CrossRef PubMed.
- Y. Leng, P. Ming, D. Yang and C. Zhang, Stainless steel bipolar plates for proton exchange membrane fuel cells: materials, flow channel design and forming processes, J. Power Sources, 2020, 451, 227783 CrossRef CAS.
- A. J. Slate, K. A. Whitehead, D. A. C. Brownson and C. E. Banks, Microbial fuel cells: an overview of current technology, Renewable Sustainable Energy Rev., 2019, 101, 60–81 CrossRef CAS.
- J. M. Khudzari, Y. Gariépy, J. Kurian, B. Tartakovsky and G. S. V. Raghavan, Effects of biochar anodes in rice plant microbial fuel cells on the production of bioelectricity, biomass, and methane, Biochem. Eng. J., 2019, 141, 190–199 CrossRef.
- Y. Zhou, L. Tang, Z. Liu, J. Hou, W. Chen and Y. Li, et al., A novel anode fabricated by three-dimensional printing for use in urine-powered microbial fuel cell, Biochem. Eng. J., 2017, 124, 36–43 CrossRef CAS.
- R. Karthikeyan, B. Wang, J. Xuan, J. W. C. Wong, P. K. H. Lee and M. K. H. Leung, Interfacial electron transfer and bioelectrocatalysis of carbonized plant material as effective anode of microbial fuel cell, Electrochim. Acta, 2015, 157, 314–323 CrossRef CAS.
- S. Chen, G. He, X. Hu, M. Xie, S. Wang and D. Zeng, et al., A Three-Dimensionally Ordered Macroporous Carbon Derived from a Natural Resource as Anode for Microbial Bioelectrochemical Systems, ChemSusChem, 2012, 5(6), 1059–1063 CrossRef CAS PubMed.
- W. P. Q. Ng, H. L. Lam, F. Y. Ng, M. Kamal and J. H. E. Lim, Waste-to-wealth: green potential from palm biomass in Malaysia, J. Cleaner Prod., 2012, 34, 57–65 CrossRef.
- Y. Liu, X. Zhang, Q. Zhang and C. Li, Microbial Fuel Cells: Nanomaterials Based on Anode and Their Application, Energy Technol., 2020, 8(9), 2000206 CrossRef.
- P. Wang, H. Li and Z. Du, Polyaniline synthesis by cyclic voltammetry for anodic modification in microbial fuel cells, Int. J. Electrochem. Sci., 2014, 9, 2038–2046 Search PubMed.
- C. J. Kirubaharan, G. G. Kumar, C. Sha, D. Zhou, H. Yang and K. S. Nahm, et al., Facile fabrication of Au@polyaniline core-shell nanocomposite as efficient anodic catalyst for microbial fuel cells, Electrochim. Acta, 2019, 328, 135136 CrossRef CAS.
- A. Sumisha and K. Haribabu, Modification of graphite felt using nano polypyrrole and polythiophene for microbial fuel cell applications-a comparative study, Int. J. Hydrogen Energy, 2018, 43(6), 3308–3316 CrossRef CAS.
- G. Wang and C. Feng, Electrochemical Polymerization of Hydroquinone on Graphite Felt as a Pseudocapacitive Material for Application in a Microbial Fuel Cell, Polymers, 2017, 9(12), 220 CrossRef PubMed.
- Y. Zhang, X. Chen, Y. Yuan, X. Lu, Z. Yang and Y. Wang, et al., Long-term effect of carbon nanotubes on electrochemical properties and microbial community of electrochemically active biofilms in microbial fuel cells, Int. J. Hydrogen Energy, 2018, 43(33), 16240–16247 CrossRef CAS.
- D. Zhong, X. Liao, Y. Liu, N. B. Zhong and Y. Xu, Quick Start-Up and Performance of Microbial Fuel Cell Enhanced with a Polydiallyldimethylammonium Chloride Modified Carbon Felt Anode, Biosens. Bioelectron., 2018, 119, 70–78 CrossRef CAS PubMed.
- X. Chen, Y. Li, X. Yuan, N. Li, W. He and J. Liu, Synergistic effect between poly(diallyldimethylammonium chloride) and reduced graphene oxide for high electrochemically active biofilm in microbial fuel cell, Electrochim. Acta, 2020, 359, 136949 CrossRef CAS.
- Y.-Y. Chen and H.-Y. Wang, Fabrication and characterization of polyelectrolyte microcarriers for microorganism cultivation through a microfluidic droplet system, Biomicrofluidics, 2016, 10, 14126 CrossRef.
- F. K. El-Baz, H. F. Aly, S. M. Abdo, R. Mahmoud and S. A. Saad, Dunaliella salina alleviates renal dysfunction and suppresses inflammatory cytokines in STZ-induced diabetic rats, Int. J. Pharm. Sci. Rev. Res., 2016, 38(2), 210–215 CAS.
- F. K. El-Baz, H. F. Aly, G. H. Ali, R. Mahmoud and S. A. Saad, Antidiabetic efficacy of Dunaliella salina extract in STZ-induced diabetic rats, Int. J. Pharma Bio Sci., 2016, 7(3), 36–45 Search PubMed.
- Q. Du, J. An, J. Li, L. Zhou, N. Li and X. Wang, Polydopamine as a new modification material to accelerate startup and promote anode performance in microbial fuel cells, J. Power Sources, 2017, 343, 477–482 CrossRef CAS.
- X.-Q. Lin, Z.-L. Li, B. Liang, J. Nan and A.-J. Wang, Identification of biofilm formation and exoelectrogenic population structure and function with graphene/polyaniline modified anode in microbial fuel cell, Chemosphere, 2019, 219, 358–364 CrossRef CAS PubMed.
- I. H. Park, M. Christy, P. Kim and K. S. Nahm, Enhanced electrical contact of microbes using Fe3O4/CNT nanocomposite anode in mediator-less microbial fuel cell, Biosens. Bioelectron., 2014, 58, 75–80 CrossRef CAS PubMed.
- S. Zhou, J. Tang and Y. Yuan, Conduction-band edge dependence of carbon-coated hematite stimulated extracellular electron transfer of Shewanella oneidensis in bioelectrochemical systems, Bioelectrochemistry, 2015, 102, 29–34 CrossRef CAS PubMed.
- A. Mehdinia, E. Ziaei and A. Jabbari, Multi-walled carbon nanotube/SnO2 nanocomposite: a novel anode material for microbial fuel cells, Electrochim. Acta, 2014, 130, 512–518 CrossRef CAS.
- N. Garino, A. Sacco, M. Castellino, J. A. Muñoz-Tabares, A. Chiodoni and V. Agostino, et al., Microwave-assisted synthesis of reduced graphene oxide/SnO2 nanocomposite for oxygen reduction reaction in microbial fuel cells, ACS Appl. Mater. Interfaces, 2016, 8(7), 4633–4643 CrossRef CAS PubMed.
- E. Herrero-Hernández, T. J. Smith and R. Akid, Electricity generation from wastewaters with starch as carbon source using a mediatorless microbial fuel cell, Biosens. Bioelectron., 2013, 39(1), 194–198 CrossRef PubMed.
- K. Guo, A. Prévoteau, S. A. Patil and K. Rabaey, Engineering electrodes for microbial electrocatalysis, Curr. Opin. Biotechnol., 2015, 33, 149–156 CrossRef CAS PubMed.
- H. Hiegemann, D. Herzer, E. Nettmann, M. Lübken, P. Schulte and K.-G. Schmelz, et al., An integrated 45 L pilot microbial fuel cell system at a full-scale wastewater treatment plant, Bioresour. Technol., 2016, 218, 115–122 CrossRef CAS PubMed.
- B. E. Logan, Scaling up microbial fuel cells and other bioelectrochemical systems, Appl. Microbiol. Biotechnol., 2010, 85(6), 1665–1671 CrossRef CAS PubMed.
- Y. Dong, Y. Qu, W. He, Y. Du, J. Liu and X. Han, et al., A 90-liter stackable baffled microbial fuel cell for brewery wastewater treatment based on energy self-sufficient mode, Bioresour. Technol., 2015, 195, 66–72 CrossRef CAS PubMed.
- D. Jiang, M. Curtis, E. Troop, K. Scheible, J. McGrath and B. Hu, et al., A pilot-scale study on utilizing multi-anode/cathode microbial fuel cells (MAC MFCs) to enhance the power production
in wastewater treatment, Int. J. Hydrogen Energy, 2011, 36(1), 876–884 CrossRef CAS.
- F. Qian, M. Baum, Q. Gu and D. E. Morse, A 1.5 μL microbial fuel cell for on-chip bioelectricity generation, Lab Chip, 2009, 9(21), 3076–3081 RSC.
- R. Veerubhotla, A. Bandopadhyay, D. Das and S. Chakraborty, Instant power generation from an air-breathing paper and pencil based bacterial bio-fuel cell, Lab Chip, 2015, 15(12), 2580–2583 RSC.
- H. Jiang, L. J. Halverson and L. Dong, A miniature microbial fuel cell with conducting nanofibers-based 3D porous biofilm, J. Micromech. Microeng., 2015, 25(12), 125017 CrossRef.
- K. Rabaey, G. Lissens and W. Verstraete, Microbial fuel cells: performances and perspectives, in Biofuels for fuel cells: renewable energy from biomass fermentation, IWA Publishing, 2005, pp. 377–399 Search PubMed.
- S.-E. Oh and B. E. Logan, Proton exchange membrane and electrode surface areas as factors that affect power generation in microbial fuel cells, Appl. Microbiol. Biotechnol., 2006, 70(2), 162–169 CrossRef CAS PubMed.
- R. A. Rozendal, H. V. M. Hamelers and C. J. N. Buisman, Effects of membrane cation transport on pH and microbial fuel cell performance, Environ. Sci. Technol., 2006, 40(17), 5206–5211 CrossRef CAS PubMed.
- M. T. Noori, M. M. Ghangrekar, C. K. Mukherjee and B. Min, Biofouling effects on the performance of microbial fuel cells and recent advances in biotechnological and chemical strategies for mitigation, Biotechnol. Adv., 2019, 37(8), 107420 CrossRef CAS PubMed.
- L. Koók, P. Bakonyi, F. Harnisch, J. Kretzschmar, K.-J. Chae and G. Zhen, et al., Biofouling of membranes in microbial electrochemical technologies: causes, characterization methods and mitigation strategies, Bioresour. Technol., 2019, 279, 327–338 CrossRef PubMed.
- M. Ghasemi, W. R. W. Daud, M. Ismail, M. Rahimnejad, A. F. Ismail and J. X. Leong, et al., Effect of pre-treatment and biofouling of proton exchange membrane on microbial fuel cell performance, Int. J. Hydrogen Energy, 2013, 38(13), 5480–5484 CrossRef CAS.
- S.-H. Liu, C.-M. Chang and C.-W. Lin, Modifying proton exchange membrane in a microbial fuel cell by adding clay mineral to improve electricity generation without reducing removal of toluene, Biochem. Eng. J., 2018, 134, 101–107 CrossRef CAS.
- M. Vaselbehagh, H. Karkhanechi, R. Takagi and H. Matsuyama, Effect of polydopamine coating and direct electric current application on anti-biofouling properties of anion exchange membranes in electrodialysis, J. Membr. Sci., 2016, 515, 98–108 CrossRef CAS.
- S.-G. Park, P. P. Rajesh, M.-H. Hwang, K. H. Chu, S. Cho and K.-J. Chae, Long-term effects of anti-biofouling proton exchange membrane using silver nanoparticles and polydopamine on the performance of microbial electrolysis cells, Int. J. Hydrogen Energy, 2021, 46(20), 11345–11356 CrossRef CAS.
- B. Neethu, G. D. Bhowmick and M. M. Ghangrekar, A novel proton exchange membrane developed from clay and activated carbon derived from coconut shell for application in microbial fuel cell, Biochem. Eng. J., 2019, 148, 170–177 CrossRef CAS.
- A. Raychaudhuri and M. Behera, Ceramic membrane modified with rice husk ash for application in microbial fuel cells, Electrochim. Acta, 2020, 363, 137261 CrossRef CAS.
- I. Chakraborty, S. Das, B. K. Dubey and M. M. Ghangrekar, Novel low cost proton exchange membrane made from sulphonated biochar for application in microbial fuel cells, Mater. Chem. Phys., 2020, 239, 122025 CrossRef CAS.
- K. Guo, The effects of electrode surface modifications on biofilm formation and electron transfer in bioelectrochemical systems, Ghent University, 2014 Search PubMed.
- S. Malekmohammadi and S. Ahmad Mirbagheri, A review of the operating parameters on the microbial fuel cell for wastewater treatment and electricity generation, Water Sci. Technol., 2021, 84(6), 1309–1323 CrossRef CAS PubMed.
- A. P. Borole, H. O'Neill, C. Tsouris and S. Cesar, A microbial fuel cell operating at low pH using the acidophile Acidiphilium cryptum, Biotechnol. Lett., 2008, 30(8), 1367–1372 CrossRef CAS PubMed.
- L. Zhang, C. Li, L. Ding, K. Xu and H. Ren, Influences of initial pH on performance and anodic microbes of fed-batch microbial fuel cells, J. Chem. Technol. Biotechnol., 2011, 86(9), 1226–1232 CrossRef CAS.
- S. Puig, M. Serra, M. Coma, M. Cabré, M. D. Balaguer and J. Colprim, Effect of pH on nutrient dynamics and electricity production using microbial fuel cells, Bioresour. Technol., 2010, 101(24), 9594–9599 CrossRef CAS PubMed.
- S. Bagchi and M. Behera, Evaluation of the effect of anolyte recirculation and anolyte pH on the performance of a microbial fuel cell employing ceramic separator, Process Biochem., 2021, 102, 207–212 CrossRef CAS.
- S. V. Raghavulu, S. V. Mohan, R. K. Goud and P. N. Sarma, Effect of anodic pH microenvironment on microbial fuel cell (MFC) performance in concurrence with aerated and ferricyanide catholytes, Electrochem. Commun., 2009, 11(2), 371–375 CrossRef CAS.
- S. A. Patil, F. Harnisch, C. Koch, T. Hübschmann, I. Fetzer and A. A. Carmona-Martínez, et al., Electroactive mixed culture derived biofilms in microbial bioelectrochemical systems: the role of pH on biofilm formation, performance and composition, Bioresour. Technol., 2011, 102(20), 9683–9690 CrossRef CAS PubMed.
- J.-Y. Nam, H.-W. Kim, K.-H. Lim, H.-S. Shin and B. E. Logan, Variation of power generation at different buffer types and conductivities in single chamber microbial fuel cells, Biosens. Bioelectron., 2010, 25(5), 1155–1159 CrossRef CAS PubMed.
- S. Pandit, S. Ghosh, M. M. Ghangrekar and D. Das, Performance of an anion exchange membrane in association with cathodic parameters in a dual chamber microbial fuel cell, Int. J. Hydrogen Energy, 2012, 37(11), 9383–9392 CrossRef CAS.
- S. Gadkari, J.-M. Fontmorin, E. Yu and J. Sadhukhan, Influence of temperature and other system parameters on microbial fuel cell performance: numerical and experimental investigation, Chem. Eng. J., 2020, 388, 124176 CrossRef CAS.
- O. Tkach, T. Sangeetha, S. Maria and A. Wang, Performance of low temperature microbial fuel cells (MFCs) catalyzed by mixed bacterial consortia, J. Environ. Sci., 2017, 52, 284–292 CrossRef CAS PubMed.
- A. Larrosa-Guerrero, K. Scott, I. M. Head, F. Mateo, A. Ginesta and C. Godinez, Effect of temperature on the performance of microbial fuel cells, Fuel, 2010, 89(12), 3985–3994 CrossRef CAS.
- I. S. Michie, J. R. Kim, R. M. Dinsdale, A. J. Guwy and G. C. Premier, The influence of psychrophilic and mesophilic start-up temperature on microbial fuel cell system performance, Energy Environ. Sci., 2011, 4(3), 1011–1019 RSC.
- Z. Ullah and S. Zeshan, Effect of substrate type and concentration on the performance of a double chamber microbial fuel cell, Water Sci. Technol., 2020, 81(7), 1336–1344 CrossRef PubMed.
- H. Ni, K. Wang, S. Lv, X. Wang, L. Zhuo and J. Zhang, Effects of concentration variations on the performance and microbial community in microbial fuel cell using swine wastewater, Energies, 2020, 13(9), 2231 CrossRef CAS.
- D. Pant, G. Van Bogaert, L. Diels and K. Vanbroekhoven, A review of the substrates used in microbial fuel cells (MFCs) for sustainable energy production, Bioresour. Technol., 2010, 101(6), 1533–1543 CrossRef CAS PubMed.
- L. Ren, X. Zhang, W. He and B. E. Logan, High current densities enable exoelectrogens to outcompete aerobic heterotrophs for substrate, Biotechnol. Bioeng., 2014, 111(11), 2163–2169 CrossRef CAS PubMed.
- S. Kaur, L. Dutta and O. Sahu, Future of Energy Demand by Microbial Fuel Cell: Review on Green Energy, J. Adv. Res. Biol., 2020, 2(2) Search PubMed.
- S. T. Read, P. Dutta, P. L. Bond, J. Keller and K. Rabaey, Initial development and structure of biofilms on microbial fuel cell
anodes, BMC Microbiol., 2010, 10(1), 1–10 CrossRef PubMed.
- C. Li, L. Zhang, L. Ding, H. Ren and H. Cui, Effect of conductive polymers coated anode on the performance of microbial fuel cells (MFCs) and its biodiversity analysis, Biosens. Bioelectron., 2011, 26(10), 4169–4176 CrossRef CAS PubMed.
- D. F. Juang, P. C. Yang, C. H. Lee, S. C. Hsueh and T. H. Kuo, Electrogenic capabilities of Gram negative and Gram positive bacteria in microbial fuel cell combined with biological wastewater treatment, Int. J. Environ. Sci. Technol., 2011, 8(4), 781–792 CrossRef CAS.
- R. M. Donlan, Biofilms: microbial life on surfaces, Emerging Infect. Dis., 2002, 8(9), 881 CrossRef PubMed.
- D.-F. Juang, Organic removal efficiencies and power production capabilities of microbial fuel cells with pure cultures and mixed culture, APCBEE Proc., 2012, 1, 2–7 CrossRef CAS.
- N. Chabert, O. A. Ali and W. Achouak, All ecosystems potentially host electrogenic bacteria, Bioelectrochemistry, 2015, 106, 88–96 CrossRef CAS PubMed.
- J. L. Varanasi, S. Roy, S. Pandit and D. Das, Improvement of energy recovery from cellobiose by thermophilic dark fermentative hydrogen production followed by microbial fuel cell, Int. J. Hydrogen Energy, 2015, 40(26), 8311–8321 CrossRef CAS.
- Y. Lei, W. Chen and A. Mulchandani, Microbial biosensors, Anal. Chim. Acta, 2006, 568(1–2), 200–210 CrossRef CAS PubMed.
- R. H. Mahmoud, F. A. Samhan, M. K. Ibrahim, G. H. Ali and R. Y. A. Hassan, Boosting the cathode function toward the oxygen reduction reaction in microbial fuel cell using nanostructured surface modification, Electrochem. Sci. Adv., 2020, e202000002 Search PubMed.
- X. Zhang, Q. Wang, C. Tang, H. Wang, P. Liang and X. Huang, et al., High-Power Microbial Fuel Cells Based on a Carbon–Carbon Composite Air Cathode, Small, 2020, 16(15), 1905240 CrossRef CAS PubMed.
- F. D. Sanij, P. Balakrishnan, P. Leung, A. Shah, H. Su and Q. Xu, Advanced Pd-based nanomaterials for electro-catalytic oxygen reduction in fuel cells: a review, Int. J. Hydrogen Energy, 2021, 46(27), 14596–14627 CrossRef CAS.
- H. Liu, C. Koenigsmann, R. R. Adzic and S. S. Wong, Probing ultrathin one-dimensional Pd–Ni nanostructures as oxygen reduction reaction catalysts, ACS Catal., 2014, 4(8), 2544–2555 CrossRef CAS.
- P. Clauwaert, P. Aelterman, L. De Schamphelaire, M. Carballa, K. Rabaey and W. Verstraete, Minimizing losses in bio-electrochemical systems: the road to applications, Appl. Microbiol. Biotechnol., 2008, 79(6), 901–913 CrossRef CAS PubMed.
- I. Ieropoulos, J. Greenman and C. Melhuish, Microbial fuel cells based on carbon veil electrodes: stack configuration and scalability, Int. J. Energy Res., 2008, 32(13), 1228–1240 CrossRef CAS.
- Z. Ge and Z. He, Long-term performance of a 200 liter modularized microbial fuel cell system treating municipal wastewater: treatment, energy, and cost, Environ. Sci.: Water Res. Technol., 2016, 2(2), 274–281 RSC.
- P. Liang, R. Duan, Y. Jiang, X. Zhang, Y. Qiu and X. Huang, One-year operation of 1000-L modularized microbial fuel cell for municipal wastewater treatment, Water Res., 2018, 141, 1–8 CrossRef CAS PubMed.
- A. Dekker, A. T. Heijne, M. Saakes, H. V. M. Hamelers and C. J. N. Buisman, Analysis and improvement of a scaled-up and stacked microbial fuel cell, Environ. Sci. Technol., 2009, 43(23), 9038–9042 CrossRef CAS PubMed.
- S. Wu, H. Li, X. Zhou, P. Liang, X. Zhang and Y. Jiang, et al., A novel pilot-scale stacked microbial fuel cell for efficient electricity generation and wastewater treatment, Water Res., 2016, 98, 396–403 CrossRef CAS PubMed.
|
This journal is © The Royal Society of Chemistry 2022 |