DOI:
10.1039/D1RA08484D
(Review Article)
RSC Adv., 2022,
12, 6966-6973
Progress in perylene diimides for organic solar cell applications
Received
19th November 2021
, Accepted 12th February 2022
First published on 2nd March 2022
Abstract
Compared to fullerene materials, non-fullerene acceptor materials have in recent years been more widely used in organic solar cell devices due to their optical properties and due to the ease of carrying out syntheses to tune their electronic energy levels. Non-fullerene acceptors constitute a major focus of research in the development of bulk-heterojunction organic solar cells. Recent developments have yielded increased power conversion efficiency (PCE) levels for non-fullerene acceptor materials, with the PCE levels now shown to exceed 20%. Perylene diimide (PDI), a non-fullerene acceptor material, has been widely studied because of its good transmission capacity and strong electron affinity. This paper summarizes the application of PDI molecules as acceptor materials in organic solar cells in recent years, detailing the strategies and approaches of molecular design and their application effects.
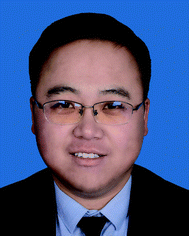 Jin Cao | |
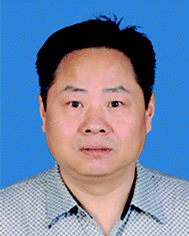 Shaopeng Yang | Shaopeng Yang is a professor in Hebei University. His research directions are organic solar cells, organic and inorganic composite perovskite solar cells, photoelectric functional materials. He is a journal reviewer in Chem. Commun. (CC), ACS Appl. Mater. Interfaces, Mater. Lett., Phys. Chem. Chem. Phys. |
1 Introduction
Solar cells are used to generate energy from solar power. Despite the current availability of various new photovoltaic technologies, such as quantum dot solar cells and perovskite-type solar cells,1–3 organic solar cells still have many advantages,4–6 including (1) low cost, light weight, good mechanical flexibility, and roll-to-roll production, offering the possibility of large-area industrial production, and (2) the ability to modulate the relevant energy levels by performing organic synthesis. The materials used at present for organic solar cell acceptors can be divided into fullerenes and non-fullerenes. Solar cells made with fullerene acceptor materials are mainly based on fullerenes and their derivatives, which are expensive and their energy levels are difficult to alter by means of chemical modification.7,8 The absorption spectra of non-fullerene acceptor materials can complement that of the donor material in the visible and near-infrared regions to achieve effective charge separation. Also, compared to fullerene acceptor materials, the non-fullerene acceptor materials can yield a better morphology in the mixed film with the donor and as a result show high charge mobility and improved charge transfer efficiency. Organic solar cells based on perylene materials were first reported in 1986.9 Compared to the bilayer system, the bulk heterojunction solar cell structure greatly increases the contact area between the donor and acceptor, hence facilitating the diffusion and dissociation of excitons in the active layer and improving cell performance. Bulk heterojunction solar cells with non-fullerene acceptor materials are gradually becoming a hot research topic.10–18
2 Result and discussion
Since 2015, the efficiency of solar cells with non-fullerene acceptor materials has been significantly improved and various non-fullerene acceptor materials have been studied extensively.19–24 Perylene diimide (PDI) materials have become a hot topic due to their high electron mobility levels. They show good photostability, thermal stability and chemical stability, show good absorption in the visible range, and are easily chemically modified. However, for a single layer of PDI solar cell devices, the efficiency is very low. Several studies have shown that the self-aggregation of PDI molecules leads to a restriction of electron transport channels, which affects the efficiency of the device.25 To overcome the self-aggregation of PDI molecules, controlling the formation of molecular Π–Π stacking and maintaining a sufficient overlap of energy levels for better electron transport are effective methods. Also, reducing the planarity of the molecules by introducing bridging groups can lessen self-aggregation.
As shown in Fig. 1(b), the PDI molecule is quite symmetric. It mainly possesses three active positions: modification of PDI units at the nitrogen position can result in a linking together of two or more PDI units, and a destruction of the self-aggregation and an inhibition of intermolecular packing; modification at the harbor position can be used to increase the conjugation length and improve the absorption properties of the molecule, thus modulating the molecular energy level; and modification at the shoulder can enhance Π–Π stacking and improve the mobility of the molecule. This paper focuses on the progress made in research about PDI molecules in recent years, in particular involving these modifications, and their applications in organic solar cells.
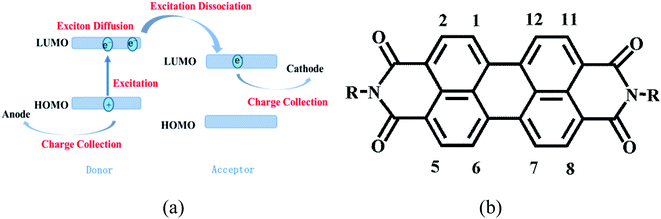 |
| Fig. 1 (a) Schematic of the working mechanism of a BHJ solar cell. (b) Molecular structure of PDI. | |
2.1 Nitrogen position modification
Substitution at the nitrogen atom is the simplest modification that does not cause distortion of the molecular plane. This substitution results in a vertical conformation of the molecular arrangement due to the spatial site resistance of the oxygen atoms.26–33 Molecules with two or more PDI units linked at the nitrogen position have been reported to yield relatively high device efficiencies (Fig. 2).26,27,32,33
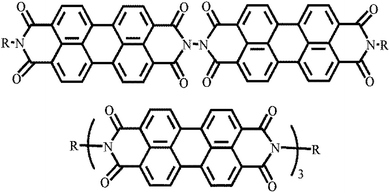 |
| Fig. 2 Structures of PDI molecules modified at the nitrogen position. | |
2.2 Harbor position modification
2.2.1 Single bond linking. As shown in Fig. 3, PDIs linked directly by a carbon–carbon single bond at the harbor position show a certain degree of distortion.34–36 The Wang group synthesized a PDI-containing molecule by linking two single PDI molecules through different bonds, and the power conversion efficiency (PCE) reached 4.39%.37,38 When replacing the donor with the polymer PTB7-TH, the PCE reached 5.90%.34 Subsequently, sulfur and selenium atoms were introduced into the PDI dimer molecule, and PCEs of, respectively, 7.16% and 8.42% were achieved.34,35 The gradual increase in PCE was mainly due to the molecular structure of the dimer having become non-planar and the two PDI units having become related by a dihedral angle resulting in a hindering of face-to-face stacking between molecules and inhibiting strong aggregation between PDI molecules. In addition, the dimer molecules were shown to achieve intermolecular electronic and orbital interactions between the individual PDIs, leading to nanoscale phase separations in the film and increasing the efficiency.
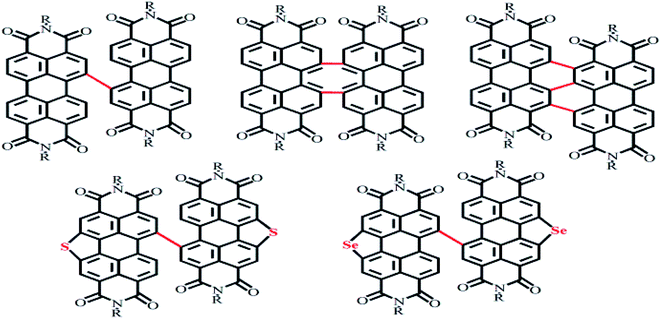 |
| Fig. 3 Structures of PDI units linked together at their harbor positions by single bonds. | |
2.2.2 Planar and non-planar linking.
2.2.2.1 Planar and non-planar dimers. Harbor position modifications can also involve connections by a planar or non-planar unit as a bridge. The Hou Jian-hui and Yao Jian-nian group reported a PDI molecular dimer with thiophene as the bridging unit39 and yielding an efficiency of 4.03%, and the Yan He group reported a PDI molecular dimer with spirofluorene as the bridging unit40 and yielding an efficiency of 9.5% (Fig. 4).
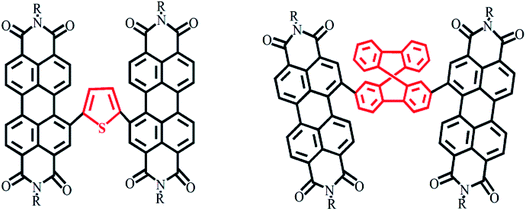 |
| Fig. 4 Structures of planar (left) and non-planar (right) PDI molecular dimers. | |
2.2.2.2 Planar and non-planar trimers. For the fullerene acceptor, the electron transport is homogeneous due to the approximate spherical shape of fullerene. For PDI molecules, the different orientation of each PDI molecular unit not only suppresses the self-aggregation in the large conjugation plane, but also promotes nano-sized phase formation, which is more conducive to intermolecular charge transport.41–43 The Chen Hong-zheng group reported a PDI trimer with a benzene as a planar unit and yielding an efficiency of 5.65%.44 A trimer of PDI molecules with triphenylamine as the bridge was reported by the Zhan Xiao-wei group to display a PCE of 3.32%.45 The Peng Qiang group reported trimers of PDI molecules with 1,3,5-triazine as the bridge and yielding an efficiency of 9.15%.46 1,3,5-Triazine is an electron-deficient group with relatively broad spectral absorption and a small twist angle in its molecular structure, which improves the crystallinity of the molecule and provides for improved Π–Π stacking for intermolecular electron transport (Fig. 5).41
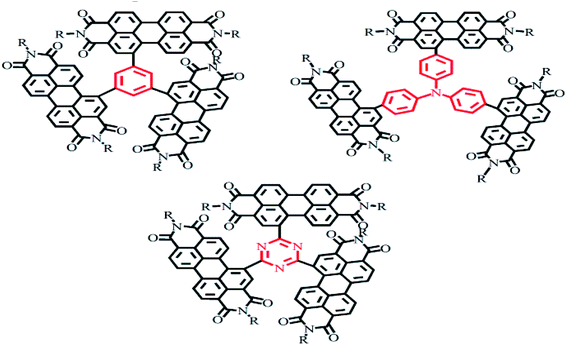 |
| Fig. 5 Structures of planar (top left and bottom) and non-planar (top right) molecular trimers. | |
2.2.2.3 Planar and non-planar tetramers. The Yan He group reported three kinds of 3D-structured PDI acceptor materials with, respectively, tetraphenylcarbon, tetraphenylstyrene and tetraphenylpyrazine as the bridge47 and analyzed the effects of the three molecular structures on their photovoltaic properties. For these molecules, self-aggregation and electron mobility were found to gradually increase with decreasing rotational freedom and the distortion of the molecule. The results showed the larger nucleus of tetraphenylpyrazine resulting in lower free rotation angles of the PDI unit—and hence a reduced distortion of the molecule. However, the electron mobility was increased due to the enhanced aggregation. The highest efficiency obtained for the molecule containing tetraphenylpyrazine as the central group was 7.1%. In addition, PDI molecules with, respectively, spirothiophene and tetraphenylpyrazoloquinoxaline have also been reported (Fig. 6).48,49
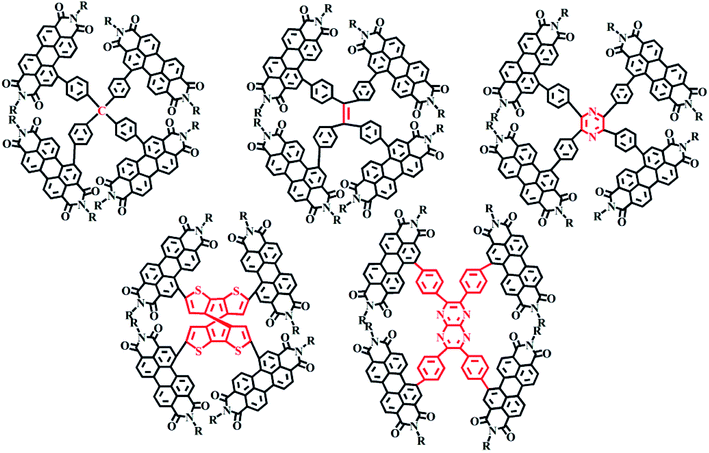 |
| Fig. 6 Structures of non-planar (top left and bottom left) and planar (rest) PDI-like molecular tetramers | |
2.2.3 Fused-ring PDI molecules. Fused-ring PDI molecules have been obtained by performing closed-loop reactions of PDI units through their harbor positions. They have a relatively extensive conjugation and good planarity to facilitate intermolecular stacking, thus improving carrier mobility. Fused-ring compounds with two PDI molecules were first reported in 2014, with an efficiency of 6.05%.50 The compounds with three and four PDI units were reported by the Chen Yong-sheng group to yield efficiencies of 7.9% and 8.3%, respectively.51 As the number of PDI units was increased, the absorption signals of the fused-ring compounds red-shifted and broadened, due to the corresponding increase in extent of conjugation, and their electron affinity increased significantly as well (Fig. 7).
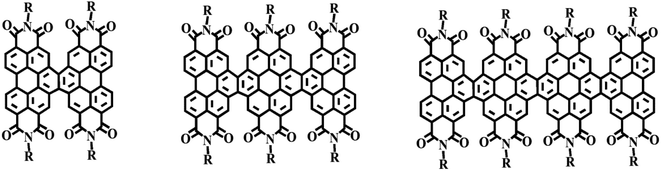 |
| Fig. 7 Structures of fused-ring PDI molecules. | |
Fused-ring PDI molecules made with, respectively, furan, thiophene and selenium atoms were reported by the Alex K.-Y. Jen group of the University of Washington. The results showed that as the radius of the central atoms was changed, the dihedral angle relating the two PDI units also changed, affecting the packing arrangement and the morphology and crystallinity of the mixed film.28 A single-bonded PDI compound based on a thiophene moiety was reported by the Yang Chu-luo group to yield a maximum efficiency of 5.84%.30 A tetrameric benzene-group-containing PDI-like fused-ring compound was reported by the Wang Zhao-hui group. The photovoltaic performances resulting from this compound and a from a selenium derivative of this compound were compared, and showed device efficiencies of 8.28% and 9.28%, respectively (Fig. 8).52
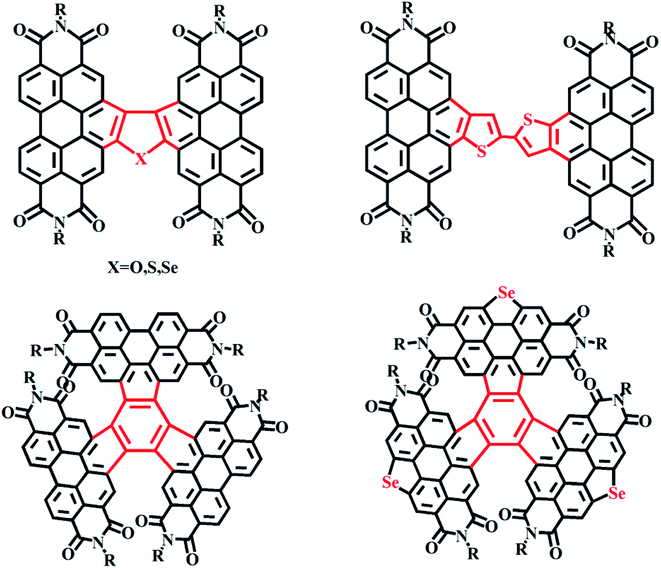 |
| Fig. 8 Structures of fused-ring PDI molecules with different central groups. | |
2.3 Shoulder position modification
It is more difficult to carry out a substitution at the shoulder position of PDI than at the harbor and N positions. Nevertheless, alkyl-chain substitutions of PDI molecules at their shoulders were reported by Müllen.53 Their impacts in solar cell devices were compared with those of N-position-modified PDI molecules. The introduction of an alkyl chain improved the solubility of PDIs and modulated the photophysical properties of the solid-state films. The efficiency effects of branched chains, stacking behavior and the morphology on the shoulder position are reported by Guo Xu-gang.54 The Yu Lu-ping group of the University of Chicago55 compared the differences between the harbor position and the shoulder position. The results indicated that the inclusion of the shoulder position groups distorted the whole molecule, but it ensured the extensive planarity of the PDI unit, which is conducive to the stacking of molecules and to high charge mobility. A 3D structure of a PDI compound modified at the shoulder position was reported. The more planar molecules resulting from shoulder position modification than from harbor position modification facilitated a tighter stacking of the Π-conjugated structure. The efficiency for this structure reached 8.47%,56 attributed to a certain degree of distortion of this structure inhibiting self-aggregation of the molecules and facilitating film formation (Fig. 9).
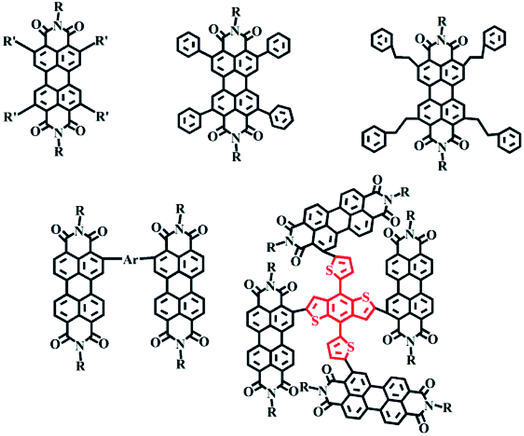 |
| Fig. 9 Structures of PDI molecules modified at the shoulder position. | |
3 Summary
In this paper, some of the main methods used to modify the PDI molecule have been introduced. In order to suppress the self-aggregation of PDI molecules, modifications at N, harbor, and shoulder positions have been reported. The modified compounds showed a broadened range of wavelengths absorbed, adjusted energy levels, and improved electron mobility, enhancing their application in photovoltaics. Therefore, the main idea has been to introduce different groups, in particular at the imide position or the harbor position, in order to control the energy levels and aggregation abilities of the molecules. In particular, PDI molecules with non-planar structures showed unique advantages due to the conformation of the molecule, which inhibit the stacking and self-aggregation and suppress the crystallization. Increasing the extent of conjugation in the structure and broadening the range of wavelengths absorbed by the molecule are expected to be very significant considerations for future design of the structures of PDIs.
Conflicts of interest
There are no conflicts to declare.
Acknowledgements
This work was supported by the Beijing Natural Science Foundation of China (2172024) and the program of Beijing Municipal Education Commission (KM202110015002).
References
- O. Amiri and M. Salavati-Niasari, et al., New Strategy to Overcome the Instability That Could Speed up the Commercialization of Perovskite Solar Cells, Adv. Mater. Interfaces, 2019, 6(9), 1900134 CrossRef.
- O. Amiri and N. Mir, et al., Design and fabrication of a high performance inorganic tandem solar cell with 11.5% conversion efficiency, Electrochim. Acta, 2017, 252, 315–321 CrossRef CAS.
- O. Amiri and M. Salavati-Niasari, et al., Plasmonic enhancement of dye-sensitized solar cells by using Au-decorated Ag dendrites as a morphology-engineered, Renewable energy, 2018, 125, 590–598 CrossRef CAS.
- Y. Cheng, S. Yang and C. Hsu, Synthesis of conjugated polymers for organic solar cell applications, Chem. Rev., 2009, 109, 5868–5923 CrossRef CAS PubMed.
- G. Li, R. Zhu and Y. Yang, Polymer solar cells, Nat. Photonics, 2012, 6, 153–161 CrossRef CAS.
- F. C. Krebs, N. Espinosa, M. Hosel, R. R. Sondergaard and M. Jorgensen, 25th anniversary article: rise to power — OPV-based solar parks, Adv. Mater., 2014, 26, 29–38 CrossRef CAS PubMed.
- A. Distler, et al., The effect of PCBM dimerization on the performance of bulk heterojunction solar cells, Adv. Energy Mater., 2014, 4, 1300693 CrossRef.
- J. T. Bloking, et al., Comparing the device physics and morphology of polymer solar cells employing fullerenes and non-fullerene acceptors, Adv. Energy Mater., 2013, 4, 1301426 CrossRef.
- C. W. Tang, Two layer organic photovoltaic cell, Appl. Phys. Lett., 1986, 48(2), 13 CrossRef.
- J. E. Anthony, Small-molecule, nonfullerene acceptors for polymer bulk heterojunction organic photovoltaics, Chem. Mater., 2011, 23, 583–590 CrossRef CAS.
- P. Sonar, J. P. F. Lim and K. L. Chan, Organic non-fullerene acceptors for organic photovoltaics, Energy Environ. Sci., 2011, 4, 1558–1574 RSC.
- C. Li and H. Wonneberger, Perylene imides for organic photovoltaics: yesterday, today, and tomorrow, Adv. Mater., 2012, 24, 613–636 CrossRef CAS PubMed.
- X. Guo, A. Facchetti and T. J. Marks, Imide- and amide-functionalized polymer semiconductors, Chem. Rev., 2014, 114, 8943–9021 CrossRef CAS PubMed.
- Y. Lin and X. Zhan, Non-fullerene acceptors for organic photovoltaics: an emerging horizon, Mater. Horiz., 2014, 1, 470–488 RSC.
- Y. Lin and X. Zhan, Designing efficient non-fullerene acceptors by tailoring extended fused-rings with electron-deficient groups, Adv. Energy Mater., 2015, 5, 1501063 CrossRef.
- C. B. Nielsen, S. Holliday, H. Chen, S. J. Cryer and I. McCulloch, Non-fullerene electron acceptors for use in organic solar cells, Acc. Chem. Res., 2015, 48, 2803–2812 CrossRef CAS PubMed.
- H. Kang, et al., From fullerene-polymer to all-polymer solar cells: the importance of molecular packing, orientation, and morphology control, Acc. Chem. Res., 2016, 49, 2424–2434 CrossRef CAS PubMed.
- Y. Lin and X. Zhan, Oligomer molecules for efficient organic photovoltaics, Acc. Chem. Res., 2016, 49, 175–183 CrossRef CAS PubMed.
- O. K. Kwon, J.-H. Park, D. W. Kim, S. K. Park and S. Y. Park, An all-small-molecule organic solar cell with high efficiency nonfullerene acceptor, Adv. Mater., 2015, 27, 1951–1956 CrossRef CAS PubMed.
- Y. Patil, R. Misra, M. L. Keshtov and G. D. Sharma, Small molecule carbazole-based diketopyrrolopyrroles with tetracyanobutadiene acceptor unit as a non-fullerene acceptor for bulk heterojunction organic solar cells, J. Mater. Chem. A, 2017, 5, 3311–3319 RSC.
- Y. Shu, et al., A survey of electron-deficient pentacenes as acceptors in polymer bulk heterojunction solar cells, Chem. Sci., 2011, 2, 363–368 RSC.
- H. Li, et al., Beyond fullerenes: design of nonfullerene acceptors for efficient organic photovoltaics, J. Am. Chem. Soc., 2014, 136, 14589–14597 CrossRef CAS PubMed.
- M. Dang, et al., Bis(tri-n-alkylsilyl oxide) silicon phthalocyanines: a start to establishing a structure property relationship as both ternary additives and non-fullerene electron acceptors in bulk heterojunction organic photovoltaic devices, J. Mater. Chem. A, 2017, 5, 12168–12182 RSC.
- X. Long, et al., Polymer acceptor based on double B←N bridged bipyridine (BNBP) unit for high-efficiency all-polymer solar cells, Adv. Mater., 2016, 28, 6504–6508 CrossRef CAS PubMed.
- S. Shoaee, et al., Acceptor energy level control of charge photogeneration in organic donor/acceptor blends, J. Am. Chem. Soc., 2010, 132, 12919–12926 CrossRef CAS PubMed.
- L. Ye, et al., Enhanced efficiency in fullerene-free polymer solar cell by incorporating fine-designed donor and acceptor materials, ACS Appl. Mater. Interfaces, 2015, 7, 9274–9280 CrossRef CAS PubMed.
- N. Liang, et al., Perylene diimide trimers based bulk heterojunction organic solar cells with efficiency over 7%, Adv. Energy Mater., 2016, 6, 1600060 CrossRef.
- H. Langhals and W. Jona, Intense dyes through chromophore–chromophore interactions: bi- and trichromophoric perylene -3,4:9,10-bis(dicarboximide)s, Angew. Chem., Int. Ed., 1998, 37, 952–955 CrossRef CAS PubMed.
- H. Langhals and S. Saulich, Bichromophoric perylene derivatives: energy transfer from non-fluorescent chromophores, Chem.–Eur. J., 2002, 8, 5630–5643 CrossRef CAS.
- M. W. Holman, P. Yan, D. M. Adams, S. Westenhoff and C. Silva, Ultrafast spectroscopy of the solvent dependence of electron transfer in a perylenebisimide dimer, J. Phys. Chem. A, 2005, 109, 8548–8552 CrossRef CAS PubMed.
- T. M. Wilson, M. J. Tauber and M. R. Wasielewski, Toward an n-type molecular wire: electron hopping within linearly linked perylenediimide oligomers, J. Am. Chem. Soc., 2009, 131, 8952–8957 CrossRef CAS PubMed.
- S. Rajaram, R. Shivanna, S. K. Kandappa and K. S. Narayan, Nonplanar perylene diimides as potential alternatives to fullerenes in organic solar cells, J. Phys. Chem. Lett., 2012, 3, 2405–2408 CrossRef CAS PubMed.
- R. Shivanna, et al., Charge generation and transport in efficient organic bulk heterojunction solar cells with a perylene acceptor, Energy Environ. Sci., 2014, 7, 435–441 RSC.
- Y. Zang, et al., Integrated molecular, interfacial, and device engineering towards high-performance non-fullerene based organic solar cells, Adv. Mater., 2014, 26, 5708–5714 CrossRef CAS PubMed.
- D. Sun, et al., Non-fullerene-acceptor-based bulk-heterojunction organic solar cells with efficiency over 7, J. Am. Chem. Soc., 2015, 137, 11156–11162 CrossRef CAS PubMed.
- D. Meng, et al., High-performance solution-processed non-fullerene organic solar cells based on selenophene-containing perylene bisimide acceptor, J. Am. Chem. Soc., 2016, 138, 375–380 CrossRef CAS PubMed.
- X. Zhan, et al., A high-mobility electron-transport polymer with broad absorption and its use in field-effect transistors and all-polymer solar cells, J. Am. Chem. Soc., 2007, 129, 7246–7247 CrossRef CAS PubMed.
- Z. Tan, et al., Efficient all-polymer solar cells based on blend of tris(thienylenevinylene)-substituted polythiophene and poly[perylene diimide- alt-bis(dithienothiophene)], Appl. Phys. Lett., 2008, 93, 073309 CrossRef.
- X. Zhang, et al., A potential perylene diimide dimer-based acceptor material for highly efficient solution-processed non-fullerene organic solar cells with 4.03% efficiency, Adv. Mater., 2013, 25, 5791–5797 CrossRef CAS PubMed.
- J. Liu, et al., Fast charge separation in a non-fullerene organic solar cell with a small driving force, Nat. Energy, 2016, 1, 16089 CrossRef CAS.
- H. Huang, et al., Recent Progress in Perylene Diimide-Based Small Molecule Acceptors for Organic Solar Cells, Acta Phys.-Chim. Sin., 2019, 35(5), 461–471 Search PubMed.
- G. C. Welch, et al., A Non-Fullerene Acceptor with a Diagnostic Morphological Handle for Streamlined Screening of Donor Materials in Organic Solar Cells, J. Mater. Chem. A, 2017, 5, 16907 RSC.
- X. Zhang, C. Zhan and J. Yao, Non-Fullerene Organic Solar Cells with 6.1% Efficiency through FineTuning Parameters of the Film-Forming Process, Chem. Mater., 2014, 27, 166 CrossRef.
- H. Chen, et al., A simple perylene diimide derivative with the highly twisted geometry as electron acceptor for efficient organic solar cells, J. Mater. Chem. A, 2013, 1–3 Search PubMed.
- Y. Lin, et al., A star-shaped perylene diimide electron acceptor for high-performance organic solar cells, Adv. Mater., 2014, 26, 5137–5142 CrossRef CAS PubMed.
- Y. Duan, et al., Pronounced effects of a triazine core on photovoltaic performance-efficient organic solar cells enabled by a PDI trimer-based small molecular acceptor, Adv. Mater., 2017, 29, 1605115 CrossRef PubMed.
- H. Lin, et al., Reduced intramolecular twisting improves the performance of 3D molecular acceptors in non-fullerene organic solar cells, Adv. Mater., 2016, 28, 8546–8551 CrossRef CAS PubMed.
- W. Huang, et al., High Performance Organic Solar Cells Based on Non-Fullerene Acceptor with a Spiro Core, Chem.–Asian J., 2017 Apr 4, 12(7), 721–725 Search PubMed.
- Y. Cao, et al., Non-planar perylenediimide acceptors with different geometrical linker units for efficient non-fullerene organic solar cells, J. Mater. Chem. A, 2017, 5(4), 1713–1723 RSC.
- Y. Zhong, et al., Efficient organic solar cells with helical perylene diimide electron acceptors, J. Am. Chem. Soc., 2014, 136, 15215–15221 CrossRef CAS PubMed.
- Y. Zhong, et al., Molecular helices as electron acceptors in high-performance bulk heterojunction solar cells, Nat. Commun., 2015, 6, 8242 CrossRef CAS PubMed.
- M. Dong, et al., High-Performance Solution-Processed Non-Fullerene Organic Solar Cells Based on Selenophene-Containing Perylene Bisimide Acceptor, J. Am. Chem. Soc., 2016, 138(1), 375–380 CrossRef PubMed.
- M. Klaus, et al., Polythiophene:Perylene Diimide Solar Cells – the Impact of Alkyl-Substitution on the Photovoltaic Performance, Adv. Energy Mater., 2011, 1, 297–302 CrossRef.
- X. Guo, et al., Slip-Stacked Perylenediimides as an Alternative Strategy for High Efficiency Nonfullerene Acceptors in Organic Photovoltaics, J. Am. Chem. Soc., 2014, 136, 16345–16356 CrossRef PubMed.
- Yu. Luping, et al., Electron Acceptors Based on α-Substituted Perylene Diimide (PDI)for Organic Solar Cells, Chem. Mater., 2016, 28, 1139–1146 CrossRef.
- Q. Wu, D. Zhao, A. M. Schneider, W. Chen and L. Yu, Covalently bound clusters of alpha-substituted PDI–rival electron acceptors to fullerene for organic solar cells, J. Am. Chem. Soc., 2016, 138, 7248–7251 CrossRef CAS PubMed.
|
This journal is © The Royal Society of Chemistry 2022 |