DOI:
10.1039/D1RA08329E
(Paper)
RSC Adv., 2022,
12, 6248-6254
Functionalized quinolizinium-based fluorescent reagents for modification of cysteine-containing peptides and proteins†
Received
13th November 2021
, Accepted 21st January 2022
First published on 22nd February 2022
Abstract
A series of quinolizinium-based fluorescent reagents were prepared by visible light-mediated gold-catalyzed cis-difunctionalization between quinolinium diazonium salts and electron-deficient alkyne-linked phenylethynyl trimethylsilanes. The electron-deficient alkynyl group of the quinolizinium-based fluorescent reagents underwent nucleophilic addition reaction with the sulfhydryl group on cysteine-containing peptides and proteins. The quinolizinium-based fluorescent reagents were found to function as highly selective reagents for the modification of cysteine-containing peptides and proteins with good to excellent conversions (up to 99%). Moreover, the modified BCArg mutants bearing cationic quinolizinium compounds 1b, 1d, 1e and 1h exhibit comparable activity in enzymatic and cytotoxicity assays to the unmodified one.
Introduction
Functionalized organic fluorescent materials have been developed as powerful tools due to their wide applications in chemistry, biology and materials science.1 Among the organic fluorescent scaffolds, fluorescein, rhodamine, coumarin, BODIPY, cyanine and, recently, Seoul-Fluor, have been intensively studied (Scheme 1a).2 However, these fluorescent materials generally have poor water-solubility, leading to limitation of versatility, sensitivity and quantitative capabilities.3 Some of the cationic heterocycles such as isoquinolinium, cinnolinium, quinolizinium, indolizinium, benzimidazolium, azinium and acridinium have been developed as fluorophores to improve water-solubility for biological research,1c,4 but only a few examples have been reported on the unique properties of cationic fluorophores in photocatalysis1c,f,g and cellular imaging.3b,c Besides, these cationic fluorescent heterocycles were first studied as bioactive drug candidates and extensively employed as DNA intercalators.4b However, applications in chemoselective modification of peptides and proteins using these cationic heterocycles remain sparse.
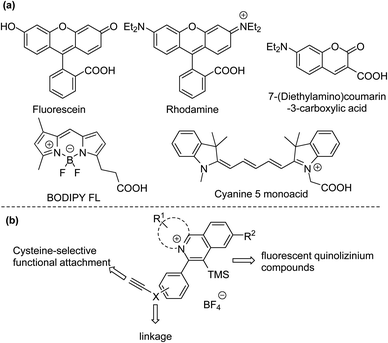 |
| Scheme 1 (a) Selected examples of organic fluorescent dyes commonly used in bioconjugation reactions. (b) Our design of electron-deficient alkyne-containing quinolizinium compounds for cysteine modification. | |
Chemoselective chemical modification of peptides and proteins is of importance in chemical biology,5 which is useful to construct well-defined bioconjugates for biological studies and development of protein-based therapeutics and diagnostics.6 In the past decades, lysine and cysteine are targets-of-interest for chemical modification due to their high nucleophilicity.7,8 However, the high prevalence of lysine on protein surfaces leads to the formation of protein conjugates with a poor level of regioselectivity.5a On the contrary, selective labeling of cysteine can be achieved by taking advantage of intrinsically low abundance (1.7%) and by incorporation using site-directed mutagenesis, rendering cysteine as an ideal target on protein bioconjugations. Conventional cysteine modifications heavily relied on S-alkylation with α-halocarbonyls and Michael addition to maleimides. Yet, owing to cross-reactivity, synthetic difficulties of α-halocarbonyls and hydrolytic instability of the maleimide-based conjugates, Davis et al.,9 Pentelute et al.,10 our group11 and others12 have reported cysteine selective modifications in metal-free and/or metal-mediated manners. Most recent advances demonstrated the use of hypervalent iodine reagents for cysteine modification.13 Despite these new achievements, the development of easily accessible and water-soluble reagents for cysteine selective modification under mild reaction conditions is still an ongoing interest.
Most recently, our group has developed a new series of quinolizinium compounds as versatile cationic fluorescent heterocycles.14 These quinoliziniums exhibit tunable photophysical properties in the visible light region and large Stokes shifts, enriching the applications of visible-light-induced photocatalysis,14a,b cellular imaging14a,c and molecular probes.14c As such, the design of functionalized quinolizinum compounds by incorporation of the electron-deficient alkynyl group delivers easily accessible reagents for cysteine modification of peptides and proteins (Scheme 1). Along with our ongoing interest in the development of bioconjugation reactions,11,15 herein, we report a series of electron-deficient alkyne-linked quinolizinium compounds prepared from the visible light-mediated gold-catalyzed alkyne cis-difunctionalization. Photophysical properties of the newly developed quinolizinium compounds were also examined. We demonstrated that the fluorescent quinolizinium compounds could be used as efficient selective modification reagents for cysteine-containing peptides. The quinoliziniums also worked well for protein modification, including labelling a therapeutic Bacillus caldovelox arginase mutant (BCArg mutant). Enzymatic and anti-cancer activities of the modified BCArg mutants have also been studied.
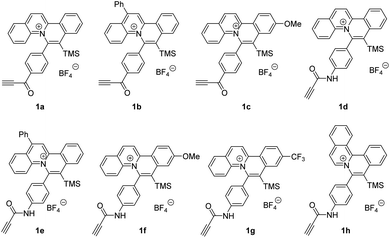 |
| Fig. 1 Chemical structures of quinoliziniums 1a–1h. | |
Results and discussion
Synthesis, characterization and photophysical properties of quinolizinium-based fluorescent reagents
We first prepared a series of quinolizinium-based fluorescent reagents 1a–1h using visible light-mediated gold-catalyzed alkyne cis-difunctionalization developed by our group (Fig. 1).14a Using quinolizinium 1a as an example, it can be prepared by treatment of quinoline-substituted aryl diazonium 3a (0.12 mmol, 1.2 equiv.) and electron-deficient alkyne-containing phenylethynyl trimethylsilane 2a (0.10 mmol, 1 equiv.) with Ph3PAuCl (10 mol%) under irradiation of blue LEDs for 16 h. Electron-deficient alkyne-linked quinolizinium 1b–1h were also prepared using the same method. Both alkynoic ketones 1a–1c and alkynoic amides 1d–1h were isolated in up to 49% yield after flash column purification. Our results revealed that electron-deficient alkynes have a high tolerance to the visible light-mediated gold catalysis reaction without losing the reactivity towards cysteine.
The photophysical properties of electron-deficient alkyne-linked quinoliziniums 1a–1h were then measured by UV/Vis and fluorescence spectroscopies in dichloromethane (Table S3, ESI†). The results showed that the maximum absorption of the 1a–1h was in the visible light region (λabs 405–445 nm) and the maximum emission was between 478–555 nm, with quantum yield (ΦF) of up to 0.22. The molar absorptivity of these compounds was up to 15
700 M−1 cm−1. Moreover, alkynoic amides 1d–1h showed slightly larger Stokes shift than alkynoic ketones 1a–1c. In general, most of these findings were in line with our reported silyl-substituted quinoliziniums,14a which demonstrated that this class of quinolizinium compounds could be amenable for the design of chemoselective reagents by incorporation of electron-deficient alkynes (alkynonic ketones and alkynoic amides) as one of the reactive functionalities towards peptide and protein labelling.
Quinolizinium compounds as cysteine selective reagents
Cysteine-containing peptide STSSSCNLSK and quinolizinium reagent 1a were set up as model substrates for condition screening with reference to our previous works.11a In brief, by treatment of peptide STSSSCNLSK (0.1 mM) with 1a (1 equiv.) in 50 mM PBS (pH 7.4)/DMSO (90
:
10) at 25 °C for 6 h, 1a-modified STSSSCNLSK and dimerized STSSSCNLSK were afforded in 89% and 6% conversions, respectively (entry 1, Table 1). The total ion chromatogram of the crude mixture of 1a-modified STSSSCNLSK by LC-MS analysis showed the modification was efficient (Fig. S2, ESI†). Selective attachment of 1a to the cysteine sulfhydryl group of STSSSCNLSK via nucleophilic addition was confirmed by LC-MS/MS (Fig. S10, ESI†). Neither the N-terminal α-amino group nor the side chains of serine, threonine and lysine were modified. Quinolizinium reagents 1b–1h also worked well in the reaction with STSSSCNLSK that gave 82–97% conversions (entries 2–8 and Fig. S11–S24, ESI†).
Table 1 Bioconjugation reaction of quinolizinium compounds with cysteine-containing peptidesa
We then sought to demonstrate the chemoselectivity of the modification. Treatment of cysteine-containing peptides AYEMWCFSQR, AYEMWCFSQK, CSKFR, KSTFC and ASCGTN with quinolizinium compounds gave the corresponding modified peptides in up to 99% conversion (entries 9–28). MS/MS analysis revealed that only the cysteine residue was modified while other residues remained intact (Fig. S25–S64, ESI†).
Protein modification using quinolizinium compounds
After screening the peptide modification, we further explored the present reaction for protein modification by employing electron-deficient alkyne-containing quinoliziniums (Table 2). Bovine serum albumin (BSA; PDB ID: 4F5S) and human serum albumin (HSA; PDB ID: 1AO6) with a single free cysteine residue were utilized for bioconjugation. Treatment of BSA (0.1 mM) with quinolizinium reagent 1a (1–2 equiv.) in 50 mM PBS (pH 7.4)/DMSO (95
:
5) at 25 °C for 16 h afforded 1a-modified BSA in 74% and 80% conversions (entries 1 and 2), respectively, as confirmed by LC-MS analysis with other residues remained intact (Fig. S66 and S67, ESI†). In this regard, two equivalents of quinolizinium reagents were used for the bioconjugation of proteins as the unreacted reagents can be easily removed by size-exclusion chromatography (Bio-Rad Bio-Spin® 6). The reaction also worked well in 20 mM Tris·HCl (pH 7.4) (74%, entry 3; Fig. S68, ESI†).11a Use of 1b, 1d, 1e or 1h (2 equiv.) afforded the corresponding modified BSA in 28–65% conversions (entries 4–7 and Fig. S69–S72, ESI†). Apart from BSA, HSA was also found to have 59–70% conversions (entries 8–12 and Fig. S77–S81, ESI†). In addition, trypsin digestion of modified BSA and HSA was conducted. After LC-MS/MS analysis of tryptic proteins, the modification was only found on Cys34 residue on peptide fragments, GLVLIAFSQYLQQCPFDEHVK of modified BSA and ALVLIAFAQYLQQCPFEDHVK of modified HSA, while other residues remained intact (Fig. S73–S75, S82 and S83, ESI†). Notably, no modification was observed with lysozyme (PDB ID: 3LYZ), which contains no free cysteine residue (Entries 13–17). These results indicated that our functionalized quinolizinium compounds could be employed as efficient chemoselective reagents in protein modification.
Table 2 Bioconjugation reaction of quinolizinium compounds with proteinsa
Labelling of proteins with fluorescent reagents can work as protein stains in SDS-PAGE gel and has the potential for in vivo tracking the uptake and physiological activities. Therefore, we performed the use of the present functionalized quinolizinium-based fluorescent reagents for labelling of proteins. As shown in SDS-PAGE analysis (Table 3), it was found that 1b-modified BSA and HSA (from entries 4 and 9, Table 2) gave a fluorescent signal excitation at UV 472 nm in SDS-PAGE protein analysis. Treatment of 1b with lysozyme gave no fluorescent signal (from entry 14, Table 2). The results of protein conjugates from SDS-PAGE analysis were found to be consistent to that of LC-MS analysis.
Table 3 SDS-PAGE analysis of 1b-modified proteins
Protein |
1b |
UV 472 nm |
Coomassie blue |
− |
+ |
− |
+ |
BSA |
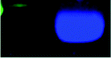 |
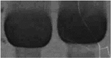 |
HSA |
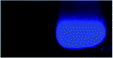 |
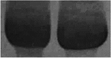 |
Lysozyme |
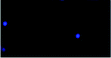 |
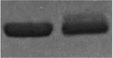 |
Biological studies of modified BCArg mutants
Human arginase is a metalloenzyme, in which its PEGylated form was the first generation of therapeutic protein with an extended half-life that has been reported to treat hepatocellular carcinoma,16 lung cancer,17 pancreatic cancer18 and immunotherapy-resistant melanoma19 in preclinical and clinical trials, respectively. Then, Bacillus caldovelox arginase mutant (BCArg mutant) and its PEGylated form (at the position of Cys161) have has been established as the second generation of therapeutic protein for the treatment of a broad spectrum of cancers such as lung and cervical cancers.20 Apart from BSA and HSA, we also employed the use of present quinolizinium reagents to selectively modify cysteine residue of the BCArg mutant. BCArg mutant (0.1 mM) was first treated with quinolizinium 1a (1 and 2 equiv.) in 20 mM Tris·HCl (pH 7.4)/DMSO (95
:
5) at 25 °C for 16 h to give 1a-modified BCArg mutant in 60% and 82% conversions, respectively (Table 4; Fig. S85 and S86, ESI†). Then, 1b, 1d, 1e and 1h (2 equiv.) gave the corresponding modified BCArg mutants in 78–84% conversions (Table 4 and Fig. S87–S90, ESI†). Excellent cysteine selectivity was demonstrated by the tryptic peptide fragments of 1e- and 1h-modified BCArg mutants found in LC-MS/MS analysis (Fig. S91 and S92, ESI†).
Table 4 Enzymatic activities and IC50 values of BCArg mutant and modified BCArg mutants
Samplea |
Conversionb (%) |
Specific activity (U mg−1) |
IC50 values for LoVo (U mL−1) |
Treatment of proteins (0.1 mM) with quinolizinium (2 equiv.) in 20 mM Tris·HCl (pH 7.4)/MSO (95 : 5) for 16 h. Determined by LC-MS analysis. Quinolizinium (1 equiv.) was used. |
BCArg mutant |
— |
202 ± 20 |
0.067 ± 0.012 |
1a-Modified BCArg mutant |
60c |
— |
— |
1a-Modified BCArg mutant |
82 |
138 ± 16 |
0.077 ± 0.025 |
1b-Modified BCArg mutant |
80 |
179 ± 7 |
0.082 ± 0.030 |
1d-Modified BCArg mutant |
84 |
185 ± 18 |
0.062 ± 0.008 |
1e-Modified BCArg mutant |
78 |
130 ± 9 |
0.079 ± 0.022 |
1h-Modified BCArg mutant |
80 |
169 ± 13 |
0.065 ± 0.004 |
To study the influence of the cationic quinolizinium-based reagents used in the modification on the biological properties of therapeutic proteins, we then evaluated enzyme activities and anti-cancer properties of the modified BCArg mutants with the unmodified analogue (Table 4). The enzymatic properties of the 1b-, 1d- and 1h-modified BCArg mutants were comparable to that of the unmodified BCArg mutant, while 1a- and 1e-modified BCArg mutants displayed slightly lower enzymatic activities. The anti-cancer properties of the unmodified and modified BCArg mutants were then examined using human colon cancer cell line LoVo (CCL-229™, American Type Culture Collection). Experimental IC50 values indicated that the anticancer efficacy of all modified BCArg mutants were comparable to that of the unmodified one. These findings indicated that the modified BCArg mutants retained their biological activities and anticancer efficacies after the bioconjugation with no significant influence over the cationic charge of the quinolizinium reagents.
Conclusions
In this work, we have developed a series of electron-deficient alkyne-containing quinoliziniums as useful reagents for cysteine-selective modification of peptides and proteins with up to 99% conversion. The present method was successfully applied on selective modification of proteins, including the therapeutic protein (BCArg mutant). Most of the resulting cationic quinolizinium-modified BCArg mutants have comparable enzymatic activities and anticancer efficacies to those of the unmodified one.
Experimental
All reagents were commercially available and used without further purification. All peptides (>98% purity) were directly purchased from GL Biochem (Shanghai) Ltd. BSA, HSA and lysozyme were purchased from Sigma Aldrich and used without further purification. Milli-Q® water used as reaction solvent in peptide and protein modification, as well as LC-MS analysis, was deionised using a Milli-Q® Gradient A10 system (Millipore, Billerica, USA). Flash column chromatography was performed using silica gel 60 (230–400 mesh ASTM) with ethyl acetate/n-hexane or methanol/dichloromethane as eluent. All NMR spectra were recorded on a Bruker DPX-400 spectrometer. All chemical shifts are quoted on the scale in ppm using TMS or residual solvent as the internal standard. Coupling constants (J) are reported in Hertz (Hz) with the following splitting abbreviations: s = singlet, br s = broad singlet, d = doublet, dd = double doublet, t = triplet and m = multiplet. All mass spectra were obtained on an ESI source of Agilent 6540 Ultra High Definition (UHD) Accurate-Mass Q-TOF LC/MS systems in the positive and negative ion modes.
All the photochemical experiments were performed in a custom made “light box” with 4 reaction vessels surrounded by 16 blue LED light bulbs. The temperature was maintained by a fan attached to the “light box”. A voltage transformer was connected with the blue LEDs and employed to monitor the power of the light source (P = U × I = 14.3 V × 2.3 A = 32.9 W). 4 reactions were performed in the “light box” every time for measurement of the reaction yields. The emission spectra of the blue LEDs revealed a maximum emission wavelength of the light source at λmax = 468 nm (Fig. S1, ESI†).
General procedure for visible light-mediated gold-catalyzed synthesis of quinolizinium compounds 1a–1h
A solution of aryl diazonium salts 3a–3e (1.2 equiv.), silyl substituted alkynes 2a or 2b (0.5 mmol, 1 equiv.), Ph3PAuCl (10 mol%) and 5 mL of CH3CN was added into a 20 mL test tube. The test tube capped with a rubber septum was evacuated and refilled with nitrogen three times. After that, the tube containing the reaction mixture was irradiated with blue LEDs for 16 h. After the reaction completed, the mixture was concentrated under reduced pressure. The crude product was purified by flash column chromatography using methanol/dichloromethane as eluent to afford compound 1a–1h.
Compound 1a. Yellow powder, 49% yield. 1H NMR (400 MHz, CD3CN) δ 9.00 (d, J = 9.0 Hz, 1H), 8.90 (t, J = 7.7 Hz, 2H), 8.43 (d, J = 8.2 Hz, 1H), 8.26–8.20 (m, 3H), 8.20–8.13 (m, 1H), 8.09–8.03 (m, 1H), 7.70 (t, J = 7.5 Hz, 1H), 7.66 (d, J = 8.4 Hz, 2H), 7.52 (d, J = 8.9 Hz, 1H), 7.37–7.30 (m, 1H), 4.06 (s, 1H), 0.07 (d, J = 3.3 Hz, 9H). 13C NMR (100 MHz, CDCl3) δ 176.23, 149.39, 144.58, 143.82, 143.11, 137.40, 136.77, 136.16, 135.28, 134.45, 132.40, 131.07, 130.71, 130.19, 129.75, 129.39, 129.16, 129.11, 128.35, 127.48, 125.31, 125.04, 119.24, 82.92, 79.92, 2.34. HRMS (ESI) m/z calcd for C29H24NOSi+ [M–BF4−]+ 430.1622, found 430.1623; m/z calcd for BF4− [M–C29H24NOSi+]− 87.0035, found 87.0034.
Compound 1b. Yellow powder, 25% yield. 1H NMR (400 MHz, acetone-d6) δ 9.35 (d, J = 8.4 Hz, 1H), 9.27 (s, 1H), 8.59 (d, J = 8.0 Hz, 1H), 8.35 (d, J = 8.6 Hz, 2H), 8.34–8.25 (m, 1H), 8.21–8.12 (m, 2H), 8.07–7.99 (m, 4H), 7.90 (d, J = 8.9 Hz, 1H), 7.83–7.75 (m, 4H), 7.56–7.47 (m, 1H), 4.61 (s, 1H), 0.17 (s, 9H). 13C NMR (100 MHz, acetone-d6) δ 196.12, 189.90, 184.16, 173.96, 168.85, 165.37, 157.32, 157.19, 157.03, 155.09, 154.50, 153.05, 150.89, 150.47, 150.22, 149.57, 149.45, 149.41, 149.35, 148.44, 148.25, 148.01, 147.73, 147.44, 146.32, 145.40, 138.26, 103.91, 99.90, 49.58, 49.39, 49.20, 49.00, 48.81, 48.62, 48.43, 21.34. HRMS (ESI) m/z calcd for C35H28NOSi+ [M–BF4−]+ 506.1935, found 506.1951; m/z calcd for BF4− [M–C35H28NOSi+]− 87.0035, found 87.0034.
Compound 1c. Brown powder, 17% yield. 1H NMR (400 MHz, CDCl3) δ 9.00 (d, J = 8.3 Hz, 1H), 8.93 (d, J = 9.0 Hz, 1H), 8.75 (d, J = 7.9 Hz, 1H), 8.27 (d, J = 7.2 Hz, 2H), 8.07 (d, J = 7.8 Hz, 1H), 7.66–7.54 (m, 5H), 7.44 (d, J = 8.6 Hz, 1H), 7.24 (t, J = 7.8 Hz, 1H), 4.12 (s, 3H), 3.62 (s, 1H), 0.13 (s, 9H). 13C NMR (100 MHz, CDCl3) δ 176.19, 164.59, 148.66, 145.13, 143.70, 141.75, 137.40, 135.98, 133.65, 132.36, 130.67, 130.19, 129.81, 129.57, 128.85, 128.38, 124.90, 124.10, 120.84, 119.57, 119.10, 111.28, 82.85, 79.92, 56.43, 31.56, 30.32, 2.40. HRMS (ESI) m/z calcd for C30H26NO2Si+ [M–BF4−]+ 460.1727, found 460.1719; m/z calcd for BF4− [M–C30H26NOSi+]− 87.0035, found 87.0033.
Compound 1d. Yellow powder, 41% yield. 1H NMR (400 MHz, acetone-d6) δ 10.19 (s, 1H), 9.29 (d, J = 9.0 Hz, 1H), 9.12 (dd, J = 13.1, 8.7 Hz, 2H), 8.53 (d, J = 8.2 Hz, 1H), 8.38 (d, J = 7.9 Hz, 1H), 8.25 (t, J = 7.5 Hz, 1H), 8.11 (t, J = 7.7 Hz, 1H), 7.90 (d, J = 8.4 Hz, 2H), 7.85 (d, J = 9.0 Hz, 1H), 7.79 (t, J = 7.5 Hz, 1H), 7.65 (d, J = 8.5 Hz, 2H), 7.50 (t, J = 8.0 Hz, 1H), 3.85 (s, 1H), 0.16 (s, 9H). 13C NMR (100 MHz, acetone-d6) δ 169.64, 166.67, 162.03, 160.83, 157.29, 156.92, 154.73, 154.39, 153.82, 152.95, 150.28, 149.70, 149.33, 149.21, 149.17, 149.11, 147.04, 145.60, 145.36, 139.99, 139.92, 138.83, 49.58, 49.39, 49.20, 49.00, 48.81, 48.62, 48.43, 21.39. HRMS (ESI) m/z calcd for C29H25N2OSi+ [M–BF4−]+ 445.1731, found 445.1790; m/z calcd for BF4− [M–C29H25NOSi+]− 87.0035, found 87.0032.
Compound 1e. Deep yellow powder, 45% yield. 1H NMR (400 MHz, CD3CN) δ 9.19 (s, 1H), 8.96 (d, J = 8.5 Hz, 1H), 8.89 (s, 1H), 8.38 (d, J = 8.3 Hz, 1H), 8.12 (t, J = 7.5 Hz, 1H), 8.06 (d, J = 7.5 Hz, 1H), 8.00 (t, J = 7.5 Hz, 1H), 7.92 (dd, J = 6.4, 2.9 Hz, 2H), 7.77–7.68 (m, 6H), 7.62 (t, J = 7.6 Hz, 1H), 7.50 (d, J = 8.0 Hz, 2H), 7.35 (t, J = 8.6 Hz, 1H), 3.45 (s, 1H), 0.10 (s, 9H). 13C NMR (100 MHz, acetone-d6) δ 173.53, 168.68, 157.42, 157.30, 155.12, 154.32, 153.32, 153.00, 150.76, 150.20, 150.08, 149.29, 149.21, 149.11, 148.02, 147.54, 147.33, 146.11, 145.28, 139.94, 138.18, 95.63, 49.58, 49.39, 49.19, 49.00, 48.81, 48.62, 48.42, 21.37. HRMS (ESI) m/z calcd for C35H29N2OSi+ [M–BF4−]+ 521.2044, found 521.2052; m/z calcd for BF4− [M–C35H29N2OSi+]− 87.0035, found 87.0032.
Compound 1f. Yellow powder, 48% yield. 1H NMR (400 MHz, CD3CN) δ 9.23 (s, 1H), 8.81–8.74 (m, 2H), 8.66 (d, J = 9.0 Hz, 1H), 8.11 (d, J = 7.8 Hz, 1H), 7.69 (d, J = 8.5 Hz, 2H), 7.65–7.60 (m, 2H), 7.59 (d, J = 2.2 Hz, 1H), 7.50 (d, J = 8.9 Hz, 1H), 7.40 (d, J = 8.5 Hz, 2H), 7.30 (dd, J = 11.7, 4.3 Hz, 1H), 4.11 (s, 3H), 3.45 (s, 1H), 0.10 (s, 9H). 13C NMR (100 MHz, CD3OD) δ 165.86, 152.41, 150.02, 148.25, 141.86, 137.87, 135.94, 134.02, 133.64, 130.60, 130.47, 130.08, 129.70, 126.39, 121.75, 121.40, 120.74, 119.42, 111.51, 77.20, 56.96, 2.28. HRMS (ESI) m/z calcd for C30H27N2O2Si+ [M–BF4−]+ 475.1836, found 475.1847; m/z calcd for BF4− [M–C30H27N2OSi+]− 87.0035, found 87.0034.
Compound 1g. Yellow powder, 43% yield. 1H NMR (400 MHz, CD3OD) δ 9.24 (d, J = 9.0 Hz, 2H), 9.08 (d, J = 8.9 Hz, 1H), 8.58 (s, 1H), 8.30 (dd, J = 19.0, 8.2 Hz, 2H), 7.78 (dd, J = 12.3, 5.7 Hz, 4H), 7.54–7.42 (m, 3H), 3.85 (s, 1H), 0.16 (s, 9H). 13C NMR (100 MHz, CD3OD) δ 144.33, 138.08, 136.32, 133.96, 133.12, 131.30, 130.70, 130.36, 129.92, 126.62, 125.50, 121.46, 119.88, 49.64, 49.43, 49.21, 49.00, 48.79, 48.57, 48.36, 2.17. 19F NMR (376 MHz, DMSO-d6) δ −61.80, −148.29. HRMS (ESI) m/z calcd for C30H24F3N2OSi+ [M–BF4−]+ 513.1605, found 513.1616; m/z calcd for BF4− [M–C30H24F3N2OSi+]− 87.0035, found 87.0036.
Compound 1h. Yellow powder, 10% yield. 1H NMR (400 MHz, CD3OD) δ 9.12 (d, J = 8.5 Hz, 1H), 9.05 (d, J = 8.4 Hz, 1H), 8.60 (d, J = 8.3 Hz, 1H), 8.36 (d, J = 7.4 Hz, 1H), 8.31 (d, J = 7.8 Hz, 1H), 8.22 (dd, J = 15.7, 7.6 Hz, 2H), 8.16 (dd, J = 18.0, 9.7 Hz, 1H), 8.11 (d, J = 7.4 Hz, 1H), 8.07 (t, J = 7.8 Hz, 1H), 7.96 (d, J = 8.5 Hz, 2H), 7.62 (d, J = 8.6 Hz, 2H), 3.86 (s, 1H), 0.22 (s, 9H). 13C NMR (100 MHz, CD3OD) δ 152.55, 150.28, 145.69, 142.47, 139.33, 136.23, 134.96, 134.43, 133.79, 133.42, 131.96, 131.06, 130.88, 129.47, 128.55, 126.13, 122.67, 121.99, 77.29, 49.64, 49.43, 49.22, 49.00, 48.79, 48.58, 48.37, 1.69. HRMS (ESI) m/z calcd for C29H25N2OSi+ [M–BF4−]+ 445.1731, found 445.1741; m/z calcd for BF4− [M–C29H25N2OSi+]− 87.0035, found 87.0033.
Author contributions
M.-K. Wong and Y.-C. Leung conceptualized and supervised the study. C.-F. Xu and K. K.-Y. Kung performed the organic synthesis in this work. C.-F. Xu, K. K.-Y. Kung and Q. Yu performed characterization of all compounds. K. K.-Y. Kung and W.-Y. O performed UV/Vis and fluorescence measurements, bioconjugation experiments and mass spectroscopy analysis of this work. S.-F. Chung and S.-Y. Tam purified and measured the biological activities of the anticancer proteins. K. K.-Y. Kung prepared this paper.
Conflicts of interest
M.-K. Wong, K. K.-Y. Kung, Q. Yu and W.-Y. O filed a patent on electron-deficient alkyne-containing quinolizinium compounds (CN113402538A).
Acknowledgements
We gratefully acknowledge the financial support from the Shenzhen Science and Technology Innovation Commission (JCYJ20170818104257975), Hong Kong Research Grants Council (15300117), the State Key Laboratory of Chemical Biology and Drug Discovery, The Hong Kong Polytechnic University (G-ZVVG and G-UACN), and Innovation and Technology Commission (PRP/075/19FX). We acknowledge the support on mass spectrometry from the University Research Facility in Life Sciences (ULS) of The Hong Kong Polytechnic University.
Notes and references
-
(a) H. Uoyama, K. Goushi, K. Shizu, H. Nomura and C. Adachi, Nature, 2012, 492, 234–238 CrossRef CAS PubMed;
(b) X. Li, X. Gao, W. Shi and H. Ma, Chem. Rev., 2014, 114, 590–659 CrossRef CAS PubMed;
(c) N. A. Romero and D. A. Nicewicz, Chem. Rev., 2016, 116, 10075–10166 CrossRef CAS PubMed;
(d) G. J. Hedley, A. Ruseckas and I. D. W. Samuel, Chem. Rev., 2017, 117, 796–837 CrossRef CAS PubMed;
(e) W. Sun, M. Li, J. Fan and X. Peng, Acc. Chem. Res., 2019, 52, 2818–2831 CrossRef CAS PubMed;
(f) M. V. Bobo, J. J. Kuchta and A. K. Vannucci, Org. Biomol. Chem., 2021, 19, 4816–4834 RSC;
(g) A. Vega-Peñaloza, J. Mateos, X. Companyó, M. Escudero-Casao and L. Dell'Amico, Angew. Chem., Int. Ed., 2021, 60, 1082–1097 CrossRef PubMed.
-
(a) A. Loudet and K. Burgess, Chem. Rev., 2007, 107, 4891–4932 CrossRef CAS PubMed;
(b) H. Kobayashi, M. Ogawa, R. Alford, P. L. Choyke and Y. Urano, Chem. Rev., 2010, 110, 2620–2640 CrossRef CAS PubMed;
(c) K. M. Dean and A. E. Palmer, Nat. Chem. Biol., 2014, 10, 512–523 CrossRef CAS PubMed;
(d) E. Kim, Y. Lee, S. Lee and S. B. Park, Acc. Chem. Res., 2015, 48, 538–547 CrossRef CAS PubMed.
-
(a) U. Resch-Genger, M. Grabolle, S. Cavaliere-Jaricot, R. Nitschke and T. Nann, Nat. Methods, 2008, 5, 763–775 CrossRef CAS PubMed;
(b) W. Xu, Z. Zeng, J.-H. Jiang, Y.-T. Chang and L. Yuan, Angew. Chem., Int. Ed., 2016, 55, 13658–13699 CrossRef CAS PubMed;
(c) Z. Xu and L. Xu, Chem. Commun., 2016, 52, 1094–1119 RSC.
-
(a) S. P. Pitre, C. D. McTiernan and J. C. Scaiano, ACS Omega, 2016, 1, 66–76 CrossRef CAS PubMed;
(b) D. Sucunza, A. M. Cuadro, J. Alvarez-Builla and J. J. Vaquero, J. Org. Chem., 2016, 81, 10126–10135 CrossRef CAS PubMed;
(c) I. F. A. Mariz, S. N. Pinto, A. M. Santiago, J. M. G. Martinho, J. Recio, J. J. Vaquero, A. M. Cuadro and E. Maçôas, Commun. Chem., 2021, 4, 142 CrossRef CAS.
-
(a) N. Stephanopoulos and M. B. Francis, Nat. Chem. Biol., 2011, 7, 876–884 CrossRef CAS PubMed;
(b) C. D. Spicer and B. G. Davis, Nat. Commun., 2014, 5, 4740 CrossRef CAS PubMed;
(c) O. Boutureira and G. J. L. Bernardes, Chem. Rev., 2015, 115, 2174–2195 CrossRef CAS PubMed;
(d) O. Koniev and A. Wagner, Chem. Soc. Rev., 2015, 44, 5495–5551 RSC;
(e) C. Zhang, E. V. Vinogradova, A. M. Spokoyny, S. L. Buchwald and B. L. Pentelute, Angew. Chem., Int. Ed., 2019, 58, 4810–4839 CrossRef CAS PubMed.
-
(a) N. Krall, F. P. da Cruz, O. Boutureira and G. J. L. Bernardes, Nat. Chem., 2016, 8, 103–113 CrossRef CAS PubMed;
(b) E. A. Hoyt, M. S. D. Cal Pedro, B. L. Oliveira and J. L. Bernardes Gonçalo, Nat. Rev. Chem., 2019, 3, 147–171 CrossRef CAS;
(c) M. Haque, N. Forte and J. R. Baker, Chem. Commun., 2021, 57, 10689–10702 RSC;
(d) B. X. Li, D. K. Kim, S. Bloom, R. Y. C. Huang, J. X. Qiao, W. R. Ewing, D. G. Oblinsky, G. D. Scholes and D. W. C. MacMillan, Nat. Chem., 2021, 13, 902–908 CrossRef CAS PubMed;
(e) M. S. Kang, T. W. S. Kong, J. Y. X. Khoo and T.-P. Loh, Chem. Sci., 2021, 12, 13613–13647 RSC.
-
(a) S. Choi, S. Connelly, N. Reixach, I. A. Wilson and J. W. Kelly, Nat. Chem. Biol., 2010, 6, 133–139 CrossRef CAS PubMed;
(b) S. Asano, J. T. Patterson, T. Gaj and C. F. Barbas III, Angew. Chem., Int. Ed., 2014, 53, 11783–11786 CrossRef CAS PubMed;
(c) A. R. Nanna, X. Li, E. Walseng, L. Pedzisa, R. S. Goydel, D. Hymel, T. R. Burke Jr, W. R. Roush and C. Rader, Nat. Commun., 2017, 8, 1112 CrossRef PubMed;
(d) M. J. Matos, B. L. Oliveira, N. Martínez-Sáez, A. Guerreiro, P. M. S. D. Cal, J. Bertoldo, M. Maneiro, E. Perkins, J. Howard, M. J. Deery, J. M. Chalker, F. Corzana, G. Jiménez-Osés and G. J. L. Bernardes, J. Am. Chem. Soc., 2018, 140, 4004–4017 CrossRef CAS PubMed.
-
(a) J. M. Chalker, G. J. L. Bernardes, Y. A. Lin and B. G. Davis, Chem.–Asian J., 2009, 4, 630–640 CrossRef CAS PubMed;
(b) S. B. Gunnoo and A. Madder, ChemBioChem, 2016, 17, 529–553 CrossRef CAS PubMed.
-
(a) G. J. L. Bernardes, J. M. Chalker, J. C. Errey and B. G. Davis, J. Am. Chem. Soc., 2008, 130, 5052–5053 CrossRef CAS PubMed;
(b) J. M. Chalker, Y. A. Lin, O. Boutureira and B. G. Davis, Chem. Commun., 2009, 3714–3716 RSC.
-
(a) A. M. Spokoyny, Y. Zou, J. J. Ling, H. Yu, Y.-S. Lin and B. L. Pentelute, J. Am. Chem. Soc., 2013, 135, 5946–5949 CrossRef CAS PubMed;
(b) E. V. Vinogradova, C. Zhang, A. M. Spokoyny, B. L. Pentelute and S. L. Buchwald, Nature, 2015, 526, 687–691 CrossRef CAS PubMed;
(c) C. Zhang, M. Welborn, T. Zhu, N. J. Yang, M. S. Santos, T. Van Voorhis and B. L. Pentelute, Nat. Chem., 2016, 8, 120–128 CrossRef CAS PubMed;
(d) M. Jbara, S. Pomplun, C. K. Schissel, S. W. Hawken, A. Boija, I. Klein, J. Rodriguez, S. L. Buchwald and B. L. Pentelute, J. Am. Chem. Soc., 2021, 143, 11788–11798 CrossRef CAS PubMed.
-
(a) H.-Y. Shiu, T.-C. Chan, C.-M. Ho, Y. Liu, M.-K. Wong and C.-M. Che, Chem.–Eur. J., 2009, 15, 3839–3850 CrossRef CAS PubMed;
(b) H.-Y. Shiu, H.-C. Chong, Y.-C. Leung, M.-K. Wong and C.-M. Che, Chem.–Eur. J., 2010, 16, 3308–3313 CrossRef CAS PubMed;
(c) H.-Y. Shiu, M.-K. Wong and C.-M. Che, Chem. Commun., 2011, 47, 4367–4369 RSC;
(d) A. O.-Y. Chan, J. L.-L. Tsai, V. K.-Y. Lo, G.-L. Li, M.-K. Wong and C.-M. Che, Chem. Commun., 2013, 49, 1428–1430 RSC;
(e) K. K.-Y. Kung, H.-M. Ko, J.-F. Cui, H.-C. Chong, Y.-C. Leung and M.-K. Wong, Chem. Commun., 2014, 50, 11899–11902 RSC;
(f) J.-R. Deng, S.-F. Chung, A. S.-L. Leung, W.-M. Yip, B. Yang, M.-C. Choi, J.-F. Cui, K. K.-Y. Kung, Z. Zhang, K.-W. Lo, Y.-C. Leung and M.-K. Wong, Commun. Chem., 2019, 2, 93 CrossRef.
-
(a) J. Yu, X. Yang, Y. Sun and Z. Yin, Angew. Chem., Int. Ed., 2018, 57, 11598–11602 CrossRef CAS PubMed;
(b) Y. Zhang, X. Zhou, Y. Xie, M. M. Greenberg, Z. Xi and C. Zhou, J. Am. Chem. Soc., 2017, 139, 6146–6151 CrossRef CAS PubMed;
(c) M. S. Messina, J. M. Stauber, M. A. Waddington, A. L. Rheingold, H. D. Maynard and A. M. Spokoyny, J. Am. Chem. Soc., 2018, 140, 7065–7069 CrossRef CAS PubMed.
-
(a) A. K. Mishra, R. Tessier, D. P. Hari and J. Waser, Angew. Chem., Int. Ed., 2021, 60, 17963–17968 CrossRef CAS PubMed;
(b) S. A. Byrne, M. J. Bedding, L. Corcilius, D. J. Ford, Y. Zhong, C. Franck, M. Larance, J. P. Mackay and R. J. Payne, Chem. Sci., 2021, 12, 14159–14166 RSC.
-
(a) J.-R. Deng, W.-C. Chan, N. C.-H. Lai, B. Yang, C.-S. Tsang, B. C.-B. Ko, S. L.-F. Chan and M.-K. Wong, Chem. Sci., 2017, 8, 7537–7544 RSC;
(b) W.-M. Yip, Q. Yu, A. Tantipanjaporn, W.-C. Chan, J.-R. Deng, B. C.-B. Ko and M.-K. Wong, Org. Biomol. Chem., 2021, 19, 8507–8515 RSC;
(c) W.-Y. O, W.-C. Chan, C. Xu, J.-R. Deng, B. C.-B. Ko and M.-K. Wong, RSC Adv., 2021, 11, 33294–33299 RSC.
-
(a) A. O.-Y. Chan, C.-M. Ho, H.-C. Chong, Y.-C. Leung, J.-S. Huang, M.-K. Wong and C.-M. Che, J. Am. Chem. Soc., 2012, 134, 2589–2598 CrossRef CAS PubMed;
(b) K. K.-Y. Kung, G.-L. Li, L. Zou, H.-C. Chong, Y.-C. Leung, K.-H. Wong, V. K.-Y. Lo, C.-M. Che and M.-K. Wong, Org. Biomol. Chem., 2012, 10, 925–930 RSC;
(c) G.-L. Li, K. K.-Y. Kung and M.-K. Wong, Chem. Commun., 2012, 48, 4112–4114 RSC;
(d) G.-L. Li, K. K.-Y. Kung, L. Zou, H.-C. Chong, Y.-C. Leung, K.-H. Wong and M.-K. Wong, Chem. Commun., 2012, 48, 3527–3529 RSC;
(e) K. K.-Y. Kung, V. K.-Y. Lo, H.-M. Ko, G.-L. Li, P.-Y. Chan, K.-C. Leung, Z. Zhou, M.-Z. Wang, C.-M. Che and M.-K. Wong, Adv. Synth. Catal., 2013, 355, 2055–2070 CrossRef CAS;
(f) K. K.-Y. Kung, K.-F. Wong, K.-C. Leung and M.-K. Wong, Chem. Commun., 2013, 49, 6888–6890 RSC;
(g) F. K.-C. Leung, J.-F. Cui, T.-W. Hui, K. K.-Y. Kung and M.-K. Wong, Asian J. Org. Chem., 2015, 4, 533–536 CrossRef CAS;
(h) H.-Y. Sit, B. Yang, K. K.-Y. Kung, J. S.-L. Tam and M.-K. Wong, ChemPlusChem, 2019, 84, 1739–1743 CrossRef CAS PubMed;
(i) J.-R. Deng, N. C.-H. Lai, K. K.-Y. Kung, B. Yang, S.-F. Chung, A. S.-L. Leung, M.-C. Choi, Y.-C. Leung and M.-K. Wong, Commun. Chem., 2020, 3, 67 CrossRef CAS;
(j) H.-M. Ko, J.-R. Deng, J.-F. Cui, K. K.-Y. Kung, Y.-C. Leung and M.-K. Wong, Bioorg. Med. Chem., 2020, 28, 115375 CrossRef CAS PubMed.
- P. N.-M. Cheng, T.-L. Lam, W.-M. Lam, S.-M. Tsui, A. W.-M. Cheng, W.-H. Lo and Y.-C. Leung, Cancer Res., 2007, 67, 309–317 CrossRef CAS PubMed.
-
(a) S.-F. Chung, C.-F. Kim, S.-Y. Tam, M.-C. Choi, P.-K. So, K.-Y. Wong, Y.-C. Leung and W.-H. Lo, Appl. Microbiol. Biotechnol., 2020, 104, 3921–3934 CrossRef CAS PubMed;
(b) Y. Zhang, S.-F. Chung, S.-Y. Tam, Y.-C. Leung and X. Guan, Cancer Lett., 2021, 502, 58–70 CrossRef CAS PubMed.
- K.-M. Yu, T. P.-S. Pang, M. Cutler, M. Tian, L. Huang, J. Y.-N. Lau, S.-F. Chung, T. W.-H. Lo and T. Y.-C. Leung, Life Sci., 2021, 264, 118674 CrossRef CAS PubMed.
- C. De Santo, P. Cheng, A. Beggs, S. Egan, A. Bessudo and F. Mussai, J. Hematol. Oncol., 2018, 11, 68 CrossRef PubMed.
-
(a) S.-F. Chung, C.-F. Kim, H.-Y. Chow, H.-C. Chong, S.-Y. Tam, Y.-C. Leung and W.-H. Lo, Int. J. Mol. Sci., 2020, 21, 7445 CrossRef CAS PubMed;
(b) S.-F. Chung, C.-F. Kim, S.-Y. Kwok, S.-Y. Tam, Y. W. Chen, H.-C. Chong, S.-L. Leung, P.-K. So, K.-Y. Wong, Y.-C. Leung and W.-H. Lo, Int. J. Mol. Sci., 2020, 21, 4234 CrossRef CAS PubMed.
Footnote |
† Electronic supplementary information (ESI) available. See DOI: 10.1039/d1ra08329e |
|
This journal is © The Royal Society of Chemistry 2022 |