DOI:
10.1039/D1RA08224H
(Paper)
RSC Adv., 2022,
12, 10280-10288
Heterofunctional carbosilane polyphenolic dendrons: new antioxidants platforms†
Received
9th November 2021
, Accepted 24th March 2022
First published on 1st April 2022
Abstract
Reactive oxygen species (ROS) play a critical role in different human pathophysiological processes. ROS, together with nitrogen reactive species, generated as by-products of cellular metabolism or external factors, affects intracellular redox homeostasis. Redox-active groups found in proteins and other compounds such as polyphenols are involved in maintaining intracellular redox homeostasis. In this work, a new family of heterofunctional first-generation carbosilane dendrons functionalised with different polyphenols at the focal point and dimethylammonium groups at the periphery has been obtained through two synthetic strategies: reductive amination and straightforward amidation reaction. Their antioxidant activity has been evaluated through two spectrophotometric methods: ferric reducing antioxidant power (FRAP) assay and 2,2′-diphenyl-1-picrylhydrazyl (DPPH) radical scavenging assay to establish a correlation between the number of hydroxyl groups and the antioxidant activity.
1. Introduction
The presence of reactive oxygen species (ROS) is a consequence of cellular metabolism and external factors such as exposure to ionising radiation, tobacco smoke or hyperoxia, among others, which increase the levels of these reactive species in our organism. Increasement of intracellular ROS levels due to the imbalance between ROS generation and antioxidant defence systems results in the damage of different biomolecules such as lipids, proteins or DNA. Diseases of notable morbidity and mortality (atherosclerosis, cancer, central nervous system diseases, autoimmune diseases, ischaemia–reperfusion injury) appear to be associated with this redox imbalance.1,2 On the other hand, many chemotherapeutic agents currently used in the fight against cancer raise intracellular ROS levels producing severe side effects in healthy cells.3
Among all antioxidants reported in the literature, polyphenols exhibit unique properties. Structurally, phenolic compounds cover from simple molecules like phenolic acids to more complex polymerised compounds.4 All of them present aromatic rings with one or more hydroxyl groups in their structure and can exert their antioxidant activity not only as “scavengers” of free radicals by hydrogen atom or electron transfer as a direct mechanism5 but also as indirect activators of antioxidant enzymes.6 In addition to polyphenols of natural source, it is also possible in the literature to find polyphenolic systems that have been chemically modified to exhibit radical chain trapping activity.7–10
However, their low stability, poor solubility and reduced bioavailability are some of their most significant shortcomings.11 To overcome this drawback, nanomedicine arises as a promising alternative capable of slowing down the drug metabolism and improving their bioavailability by increasing their half-life.12 Among all nanocarriers, dendritic systems are cutting-edge platforms with high structural precision, multivalence, monodispersity, and high surface area. Several strategies for the functionalisation of dendritic structures with polyphenols through hydrophobic/hydrophilic interactions or covalent bonds have been reported showing engaging antioxidant activities.13–17 However, not only the functional groups but also the nature of the dendritic scaffold plays a crucial role in their biological activity.18 Carbosilane dendrimers present C–C and C–Si bonds providing a hydrophobic structure and enhancing their interaction with biological membranes.19–21 Our research group has demonstrated that the inclusion of different polyphenols in a carbosilane dendritic platform retains or even increases these polyphenols' antioxidant properties. Besides, these systems present anticancer activity against advanced prostate cancer cell line PC-3 when the polyphenol is vanillin22 and a bacteriostatic effect at concentrations that lack toxicity in the human skin fibroblast cell line HFF-1 when including gallic, ferulic or caffeic moieties.23 However, the carbosilane skeleton's lipophilicity decreases the solubility in an aqueous medium, limiting their therapeutic action in other fields. To overcome these drawbacks, dendritic wedges enable the design of heterofunctional systems in a controlled process that increases the bioavailability of polyphenols located at the focal point through the functionalisation of the dendritic periphery with ionic groups. However, according to our knowledge, no examples about the use of polyphenolic dendrons in biomedicine have been described.
2. Materials and methods
2.1. Synthesis and characterisation of polyphenolic dendrons
General conditions: all chemical reactions were carried out under an inert atmosphere and solvents were purified with appropriate drying agents. NMR spectra were recorded on a Varian Unity and Varian Mercury 300 MHz, and Varian 500 MHz instruments. Chemical shifts are given in ppm relative to internal deuterated solvent peaks. When necessary, the assignment of resonances was done from bidimensional {1H–13C}-HSQC-2D-NMR, {1H–13C}-HMBC-2D-NMR, {1H–1H}-COSY-2D-NMR and 1H-DOSY-2D-NMR experiments. Elemental analyses were performed on a LECO CHNS-932 instrument. Mass spectra were obtained using an Agilent 6210 TOF-LC/MS instrument for ESI-TOF in MeOH. Spectra obtained through the different techniques for the new compounds described in this work are collected in the ESI (Fig. S1–S28).† All cationic systems synthesised are soluble ans stable in aqueous media.
2.1.1. Synthesis of [(OH)(OCH3)Ph(C
N)]-G1-(NMe2)2 (1). To a dry THF solution of ([NH2-G1-(NMe2)2]) (I) (96.9 mg, 0.199 mmol), MgSO4 activated in the oven at 60 °C, vanillin (II) (30.2 mg, 0.199 mmol) was added. The mixture was stirred for 12 hours at room temperature and under an inert atmosphere. In this way, intermediate 1 was obtained but not isolated. 1H-NMR (CD3OD, 300 MHz): δ (ppm) −0.03 (s, 3H, SiCH3), −0.01 (s, 12H, Si(CH3)2), 0.39–0.75 (m, overlapping of signals, 14H, NCH2CH2CH2CH2Si, SiCH2CH2CH2Si and SiCH2CH2CH2N(CH3)2), 1.27–1.60 (m, overlapping of signals, 10H, NCH2CH2CH2CH2Si, SiCH2CH2CH2Si and SiCH2CH2CH2N(CH3)2), 1.75 (m, 2H, NCH2CH2CH2CH2Si), 2.31 (s, 12H, NCH3)2, 2.38 (m, 2H, NCH2CH2CH2N(CH3)2), 3.60 (m, 2H, NCH2CH2CH2CH2Si), 3.91 (s, 3H, OCH3), 6.78 (d, 3J(H–H) = 8.2 Hz, HAr, (ortho-OH)), 7.18 (d, 3J(H–H) = 8.2 Hz, HAr, meta-OH, ortho-C
N), 7.42 (s, HAr, ortho-OCH3, ortho-C
N), 8.16 (s, 1H, HC
N).
2.1.2. Synthesis of [(OH)2Ph(C
N)]-G1-(NMe2)2 (2). Compound 2 was obtained by using the same protocol as for compound 1. Starting from the precursor NH2G1(NMe2)2 (I) (118.1 mg, 0.242 mmol) and 3,4-dyhydroxibenzaldehyde (III) (33.4 mg, 0.242 mmol). In this way, intermediate 2 was obtained but not isolated. 1H-NMR (CDCl3, 300 MHz): δ (ppm) −0.13 (s, 3H, SiCH3), −0.12 (s, 12H, Si(CH3)2), 0.31–0.63 (m, overlapping of signals, 14H, NCH2CH2CH2CH2Si, SiCH2CH2CH2Si and SiCH2CH2CH2N(CH3)2), 1.14–1.51 (m, overlapping of signals, 10H, NCH2CH2CH2CH2Si, SiCH2CH2CH2Si and SiCH2CH2CH2N(CH3)2), 1.67 (m, 2H, NCH2CH2CH2CH2Si), 2.20–2.36 (s, overlapping of signals, 14H, N(CH3)2 and NCH2CH2CH2N(CH3)2), 3.55 (m, 2H, NCH2CH2CH2CH2Si), 6.74 (d, 3J(H–H) = 8.2 Hz, HAr, ortho-OH), 7.00 (dd, 3J(H–H) = 8.2 Hz, 4J(H–H) = 1.7 Hz, HAr, meta-OH, ortho-C
N), 7.19 (d, 4J(H–H) = 1.7 Hz, HAr, ortho-OH, ortho-C
N), 8.01 (s, 1H, HCNCH2CH2CH2CH2Si).
2.1.3. Synthesis of [(OH)(OCH3)PhCH2NH]-G1-(NMe2)2 (3). Once compound 1 was obtained, the solution was filtered, NaBH4 (7.53 mg, 0.199 mmol) was added and the mixture was maintained under stirring and inert atmosphere for 12 hours. Afterward, an extraction CH2Cl2/NaHCO3 was carried out obtaining compound 3 as a yellow oil (85.2 mg, 70%). 1H-NMR (CD3OD, 300 MHz): δ (ppm) −0.02 (s, 3H, SiCH3), 0.02 (s, 12H, Si(CH3)2), 0.43–0.70 (m, overlapping of signals, 14H, NHCH2CH2CH2CH2Si, SiCH2CH2CH2Si and SiCH2CH2CH2N(CH3)2), 1.27–1.64 (m, 12H, overlapping of signals, NHCH2CH2CH2CH2Si, SiCH2CH2CH2Si and SiCH2CH2CH2N(CH3)2), 2.26 (s, 12H, N(CH3)2), 2.33 (m, 4H, SiCH2CH2CH2N(CH3)2), 2.59 (m, 2H, NHCH2CH2CH2CH2Si), 3.66 (s, 2H,CH2NHCH2CH2CH2CH2Si), 3.88 (s, 3H, OCH3), 6.76 (s, 2HAr, (meta-OH, ortho-C
N) and (ortho-OH)), 6.95 (s, HAr, ortho-OCH3, ortho-C
N). 13C-NMR (CD3OD, 126 MHz): δ (ppm) −4.8 (SiCH3), −3.2 (Si(CH3)2), 13.9–23.0 (NH2CH2CH2CH2CH2Si, SiCH2CH2CH2Si and SiCH2CH2CH2NH(CH3)2), 34.2 (NH2CH2CH2CH2CH2Si), 45.4 (N(CH3)2), 49.0 (overlapped with the solvent, NHCH2CH2CH2CH2Si), 54.3 (CH2NH2CH2CH2CH2CH2Si), 56.4 (OCH3), 64.4 (SiCH2CH2CH2N(CH3)2), 113.3 (CAr, ortho-OCH3, ortho-C
N), 116.1 (CAr, ortho-OH), 122.4 (CAr, meta-OH, ortho-C
N), 131.4 (C ipso), 147.1 (C ipso), 149.1 (C ipso). MS: [M + H]+ = 624.48 u (calc. 624.47 u). Elemental analysis (%): calc. for C33H69N3O2Si3 (624.19 g mol−1): C, 63.50; H, 11.14; N, 6.73. Exp.: C, 62.96; H, 10.83; N, 6.25.
2.1.4. Synthesis of [(OH)2PhCH2NH]-G1-(NMe2)2 (4). Compound 4 has been obtained by following the same protocol as for compound 3, adding NaBH4 (9.2 mg, 0.242 mmol) on the reaction mixture of compound 2 (147.7 mg, 0.242 mmol). Dendron 4 was isolated as brown oil (110.7 mg, 75%). C32H67N3O2Si3 (610.16 gmol−1). 1H-NMR (CD3OD, 300 MHz): δ (ppm) −0.02 (s, 3H, SiCH3), 0.02 (s, 12H, Si(CH3)2), 0.43–0.75 (m, overlapping of signals, 14H, NHCH2CH2CH2CH2Si, SiCH2CH2CH2Si and SiCH2CH2CH2N(CH3)2), 1.26–1.65 (m, overlapping of signals, 12H, NHCH2CH2CH2CH2Si, SiCH2CH2CH2Si and SiCH2CH2CH2N(CH3)2), 2.27 (s, 12H, (NCH3)2), 2.34 (m, 4H, SiCH2CH2CH2NH(CH3)2), 2.62 (m, 2H, CH2NHCH2CH2CH2CH2Si), 3.65 (s, 2H,CH2NHCH2CH2CH2CH2Si), 6.61–6.82 (m, 3H, HAr). MS: [M + H]+ = 610.46 u (calc. 610.46 u).
2.1.5. Synthesis of [(OH)(OCH3)PhCH2NH2Cl]-G1-(NMe2HCl)2 (5). The synthesis of the compound 5 was carried out by adding 0.4 mL (0.768 mmol) of HCl (2.0 N, diethyl ether) to compound 3 (80.0 mg, 0.128 mmol) and through the evaporation of the volatile compounds. Compound 5 was obtained as a yellow oil (85.4 mg, 91%). 1H-NMR (CD3OD, 500 MHz): δ (ppm) 0.00 (s, 3H, SiCH3), 0.06 (s, 12H, Si(CH3)2), 0.50–0.73 (m, overlapping of signals, 14H, NH2CH2CH2CH2CH2Si, SiCH2CH2CH2Si and SiCH2CH2CH2NH(CH3)2), 1.27–1.51 (m, overlapping of signals, 6H, NH2CH2CH2CH2CH2Si and SiCH2CH2CH2Si), 1.65–1.83 (m, overlapping of signals, 6H, NH2CH2CH2CH2CH2Si and SiCH2CH2CH2NH(CH3)2), 2.90 (s, 12H, NH(CH3)2), 3.04 (m, 2H, NH2CH2CH2CH2CH2Si), 3.14 (m, 4H, SiCH2CH2CH2NH(CH3)2), 3.93 (s, 3H, OCH3), 4.14 (s, 2H,CH2NH2CH2CH2CH2CH2Si), 6.87 (d, 3J(H–H) = 8.0 Hz, HAr, ortho-OH), 6.99 (dd, 3J(H–H) = 8.0 Hz, 4J(H–H) = 1.6 Hz, HAr, meta-OH, ortho-C
N), 7.20 (d, HAr, 4J(H–H) = 1.6 Hz, ortho-OCH3, ortho-C
N). 13C-NMR (CD3OD, 126 MHz): δ (ppm) −5.0 (SiCH3), −3.4 (Si(CH3)2), 12.9–22.4 (NH2CH2CH2CH2CH2Si, SiCH2CH2CH2Si and SiCH2CH2CH2NH(CH3)2), 31.0 (NH2CH2CH2CH2CH2Si), 43.4 (NH(CH3)2), 49.0 (overlapped with the solvent, NH2CH2CH2CH2CH2Si), 52.3 (CH2NH2CH2CH2CH2CH2Si), 56.6 (OCH3), 62.0 (SiCH2CH2CH2NH(CH3)2), 114.6 (CAr, (ortho-OCH3, ortho-C
N), 116.5 (CAr, ortho-OH), 123.6 (CAr, meta-OH, ortho-C
N)), 124.2 (C ipso), 148.9 (C ipso), 149.3 (C ipso). MS: [M − 2Cl]+ = 330.68 u (calc. 330.73 u). Elemental analysis (%): calc. for C33H72Cl3N3O2Si3 (733.56 gmol−1): C, 54.03; H, 9.89; N, 5.73. Exp.: C, 53.76; H, 9.80; N, 5.20.
2.1.6. Synthesis of [(OH)2PhCH2NH2Cl]-G1-(NMe2HCl)2 (6). The synthesis of the compound 6 was carried out following the same protocol as for compound 3. Starting from [(OH)2PhNH]-G1-(NMe2)2 (4) (93.9 mg, 0.154 mmol) and 0.5 mL (0.924 mmol) of HCl (2.0 N, diethyl ether), obtaining compound 6 as a brown oil (94.2 mg, 85%). 1H-NMR (CD3OD, 500 MHz): δ (ppm) 0.00 (s, 3H, SiCH3), 0.07 (s, 12H, Si(CH3)2), 0.50–0.73 (m, overlapping of signals, 14H, NH2CH2CH2CH2CH2Si, SiCH2CH2CH2Si and SiCH2CH2CH2NH(CH3)2), 1.27–1.51 (m, 6H, CH2NH2CH2CH2CH2CH2Si and SiCH2CH2CH2Si), 1.65–1.83 (m, 6H, CH2NH2CH2CH2CH2CH2Si and SiCH2CH2CH2NH(CH3)2), 2.90 (s, 12H, NH(CH3)2), 3.01 (m, 2H, NH2CH2CH2CH2CH2Si), 3.13 (m, 4H, SiCH2CH2CH2NH(CH3)2), 4.05 (s, 2H,CH2NH2CH2CH2CH2CH2Si), 6.84 (s, 2HAr), 6.95 (s, HAr). 13C-NMR (CD3OD, 126 MHz): δ (ppm) −4.8 (SiCH3), −3.2 (Si(CH3)2), 12.9–22.5 (NH2CH2CH2CH2CH2Si, SiCH2CH2CH2Si and SiCH2CH2CH2NH(CH3)2), 31.1 (CH2NH2CH2CH2CH2CH2Si), 44.1 (N(CH3)2), 49.0 (overlapped with the solvent, NH2CH2CH2CH2CH2Si), 52.6 (CH2NH2CH2CH2CH2CH2Si), 62.2 (SiCH2CH2CH2NH(CH3)2), 116.6 (CAr, ortho-OH), 118.1 (CAr, ortho-OH, ortho-C
N), 123.0 (CAr, meta-OH, ortho-C
N), 123.5 (C ipso), 146.8 (C ipso), 147.7 (C ipso). MS: [M − 2Cl]+ = 323.71 u (calc. 323.72 u). Elemental analysis (%): calc. for C32H70Cl3N3O2Si3 (719.54 gmol−1): C, 53.42; H, 9.81; N, 5.84. Exp.: C,52.62; H, 9.91; N, 6.01.
2.1.7. Synthesis of [(OH)2PhCH2CH
CHC(O)NH]-G1-(NMe2)2 (7). The caffeic acid (41.5 mg, 0.230 mmol) was dissolved in dry DMF under stirring. Hydroxybenzotriazole (HOBt) (31.1 mg, 0.230 mmol) and 1-ethyl-3-(3-dimethylaminopropyl) carbodiimide hydrochloride (EDCI·HCl) (44.1 mg, 0.230 mmol) were added solved in DMF and the resulting mixture was stirred for 1 hour at room temperature. Afterwards, the mixture was added dropwise on a solution of the amine ([NH2]-G1-(NMe2)2) (I) (93.6 mg, 0.192 mmol) at 0 °C. The final solution was stirred for 12 hours at 60 °C. Then, the solvent was vacuum removed and the final compound was purified by an extraction CH2Cl2/H2O (NaHCO3), obtaining the compound 7 as a brown oil (87.4 mg, 70%). 1H-NMR (CD3OD, 300 MHz): δ (ppm) −0.02 (s, 3H, SiCH3), 0.00 (s, 12H, Si(CH3)2), 0.40–0.72 (m, overlapping of signals, 14H, C(O)NHCH2CH2CH2CH2Si, SiCH2CH2CH2Si and SiCH2CH2CH2N(CH3)2), 1.30–1.67 (m, overlapping of signals, 10H, C(O)NHCH2CH2CH2CH2Si, SiCH2CH2CH2Si and SiCH2CH2CH2N(CH3)2), 2.29 (s, 12H, N(CH3)2), 2.37 (m, 4H, SiCH2CH2CH2NH(CH3)2), 3.32 (2H, overlapped with the solvent, C(O)NHCH2CH2CH2CH2Si), 6.38 (d, 1H, PhCH
CHC(O)NH), 6.79 (HAr, ortho-OH, meta-CH
CH), 6.91 (HAr, meta-OH, ortho-CH
CH), 7.03 (HAr, ortho-OH, ortho-CH
CH), 7.41 (d, 1H, PhCH
CHC(O)NH). 13C-NMR (CD3OD, 126 MHz): δ (ppm) −4.8 (SiCH3), −3.1 (Si(CH3)2), 12.8–22.5 (C(O)NHCH2CH2CH2CH2Si, SiCH2CH2CH2Si and SiCH2CH2CH2N(CH3)2), 34.4 (C(O)NHCH2CH2CH2CH2Si), 40.1 (C(O)NHCH2CH2CH2CH2Si), 45.2 (N(CH3)2), 63.4 (SiCH2CH2CH2NH(CH3)2), 115.0 (CAr, ortho-OH, ortho-CH
CH), 116.5 (CAr, ortho-OH, meta-CH
CH), 118.3 (PhCH
CH(CO)NH), 122.1 (CAr, meta-OH, ortho-CH
CH), 128.1 (C ipso), 142.1 (PhCH
CH(CO)NH), 147.0 (C ipso), 149.4 (C ipso)), 169.2 (C(O)NH). MS: [M + H]+ = 650.45 u (calc. 650.45 u). Elemental analysis (%): calc. for C34H67N3O3Si3 (650.18 g mol−1): C, 62.81; H, 10.39; N, 6.46. Exp.: C, 62.36; H, 9.82 N, 6.13.
2.1.8. Synthesis of [(OH)2PhCH2CH
CHC(O) NH]-G1-(NMe2·HCl)2 (8). Compound 7 (87.4 mg, 134.4 mmol) was dissolved in dry THF and 0.3 mL (0.538 mmol) of HCl (2.0 N) was added and the solution was maintained 2 hours under stirring at room temperature. Afterwards, the volatile compounds were evaporated obtaining compound 8 as a brown oil (89.0 mg, 95%). 1H-NMR (CD3OD, 300 MHz): δ (ppm) −0.01 (s, 3H, SiCH3), 0.05 (s, 12H, Si(CH3)2), 0.47–0.74 (m, overlapping of signals, 14H, C(O)NHCH2CH2CH2CH2Si, SiCH2CH2CH2Si and SiCH2CH2CH2NH(CH3)2), 1.32–1.49 (m, overlapping of signals, 6H, C(O)NHCH2CH2CH2CH2Si and SiCH2CH2CH2Si), 1.62 (m, 2H, C(O)NHCH2CH2CH2CH2Si), 1.73 (m, 4H, SiCH2CH2CH2NH(CH3)2), 2.89 (s, 12H, NH(CH3)2), 3.12 (m, 4H, SiCH2CH2CH2NH(CH3)2), 3.31 (2H, overlapped with the solvent, C(O)NHCH2CH2CH2CH2Si), 6.41 (d, HAr, (3J(H–H) = 15.7 Hz), PhCH
CHC(O)NH), 6.81 (d, HAr, ortho-OH, meta-CH
CH), 6.94 (dd, HAr, 3J(H–H) = 8.2 Hz, 5J(H–H) = 2.1 Hz, meta-OH, ortho-CH
CH), 7.05 (d, HAr, 5J(H–H) = 2.1 Hz, ortho-OH, ortho-CH
CH), 7.41 (d, 1H (3J(H–H) = 15.7 Hz, PhCH
CHC(O)NH). 13C-NMR (CD3OD, 126 MHz): δ (ppm) −4.8 (SiCH3), −3.4 (Si(CH3)2), 12.8–22.4 (C(O)NHCH2CH2CH2CH2Si, SiCH2CH2CH2Si and SiCH2CH2CH2N(CH3)2)), 34.3 (C(O)NHCH2CH2CH2CH2Si), 40.0 (C(O)NHCH2CH2CH2CH2Si), 43.4 (NH(CH3)2), 62.0 (SiCH2CH2CH2NH(CH3)2), 115.1 (CAr, ortho-OH, ortho-CH
CH), 116.5 (CAr, ortho-OH, meta-CH
CH), 118.6 (CAr, PhCH
CHC(O)NH), 122.1 (CAr, meta-OH, ortho-CH
CH), 128.4 (C ipso), 142.0 (PhCH
CHC(O)NH), 146.7 (C ipso), 148.7 (C ipso), 169.2 (C(O)NH). MS: [M − 2Cl]+ = 325.73 u (calc. 325.73 u). Elemental analysis (%): calc. for C34H69Cl2N3O3Si3 (723.10 g mol−1): C, 56.48; H, 9.62; N, 9.81. Exp.: C, 57.21; H, 9.12; N, 10.01.
2.2. Determination of the antioxidant activity
The antioxidant capacity of cationic compounds 5, 6 and 8 were determined through DPPH and FRAP methods following a previously reported protocol.23
2.2.1. DPPH free radical-scavenging activity. An aliquot of 100 μL of different concentrations of the stock sample in the range of 0.01 and 100 μM in methanol was added to a 900 μL DPPH stock solution (0.12 mM) in a DMSO/H2O (1
:
1). The mixture was stirred, stored in the dark at room temperature and registered at 530 nm from 5 min to 30 min. The control consisted on methanol instead of the sample.
2.2.2. FRAP assay. Preparation of the FRAP stock solutions: mixed in a 1
:
1
:
10 ratio in the following solutions (1) TPTZ (10 mM solution in 40 mM of hydrochloric acid), (2) FeCl3 (20 mM solution in ACS water), and (3) acetate buffer (20 mM in 100 mL of ACS water, pH 3.6). Once FRAP solution was prepared, to 980 μL of a FRAP stock solution in acetate buffer was added to an aliquot of 20 μL of different concentrations of the stock sample in the range of 0.01 and 100 μM in methanol. The mixture was stirred, stored in the dark at room temperature and registered at 593 nm from 5 min to 30 min. The blank was constituted by methanol instead of the sample.The IC50 (the concentration of polyphenolic dendrons required for scavenging 50% of DPPH radical activity) and EC50 (the concentration of polyphenolic dendrons that reduce 50% of the ferric solution) were calculated following similar protocols. For each concentration, it was necessary to calculate the DPPH remnant (DPPHREM), as well as the Fe(II) formed (Fe(II)FOR), through the following equations:
|
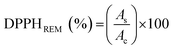 | (1) |
|
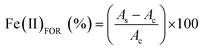 | (2) |
where
As = sample absorbance, and
Ac = control absorbance.
The graphical representation of the percentage of the remnant or formed reagent versus the −log[X] (X = sample concentration) gives the concentration that produces 50% antioxidant activity. This determination was carried out using GraphPad Prism version 6.00 for Windows, GraphPad Software (La Jolla California USA, http://www.graphpad.com). The results are expressed as mean ± SEM, and are representative of at least three independent experiments.
For the standard Trolox, the linear regression was carried out in the same way as the samples, with a range of concentrations between 5 and 40 μM for DPPH and FRAP.23 The concentration at which each compound reached maximum activity was calculated through the intersection point with the line of the equation obtained from the data before and after the steady-state. The absorbance's interpolation where the maximum activity was reached in the Trolox standard calibration curve gave us the corresponding Trolox concentration. Afterwards, to express the results as micromole of Trolox per micromole of compound or polyphenol, the Trolox concentration, obtained through interpolation, was divided by the dendrimer or polyphenol concentration corresponding to the maximum activity (Fig. S29†). The results are expressed as mean ± SEM and are representative of at least three independent experiments.
3. Results and discussion
3.1. Synthesis and structural characterisation of polyphenolic dendritic wedges
The polyphenols selected to carry out this work were vanillin (4-hydroxy-3-methoxybenzaldehyde), protocatechuic aldehyde (3,4-dihydroxibenzaldehyde) and caffeic acid (3,4-dihydroxy-cinnamic). In case of vanillin and protocatechuic aldehyde, with one and two hydroxyl groups respectively, the presence of an aldehyde group in their structure allows anchoring of the polyphenolic systems to the carbosilane dendritic wedge with amino groups at the focal point and peripheral dimethylamino groups [NH2-G1-(NMe2)2] (I)24 by reductive amination (see Scheme 1).
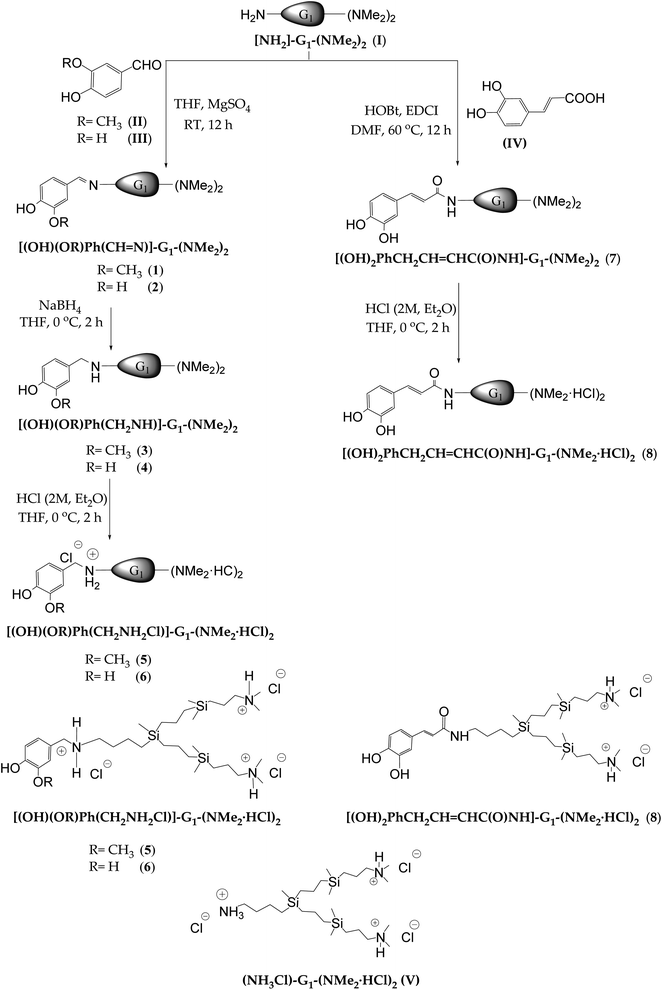 |
| Scheme 1 Synthetic strategy and proposed structures for the first generation cationic polyphenolic dendrons (5, 6 and 8) and dendron without polyphenol (V). | |
Due to the lack of stability of these imines, the reductive amination was carried out in one pot. The first step of condensation reaction in anhydrous THF as solvent and MgSO4 as desiccant agent between I and free polyphenols resulted in the imines [(OH)(OR)Ph(HC
N)]-G1-(NMe2)2 (R = CH3 (1) R = H (2)). The imine formation was confirmed through 1H-NMR by the presence of a singlet around 8.00 ppm attributed to the proton of imine (–HC
N–), and a multiplet located at 3.60 ppm attributed to the protons of the methylene group directly bonded to the nitrogen of the imine fragment (HC
N–CH2–) (Fig. 1A). Secondly, the reduction of the imine bond with NaBH4, at 0 °C in the same solvent led to the formation of the corresponding amines [(OH)(OR)Ph(CH2NH)]-G1-(NMe2)2 (R = CH3 (3) R = H (4)). The following-up of the reaction through 1H-NMR and 13C-NMR confirmed the reduction of the imine bond by the presence of a signal at 3.70 ppm and 54.0 ppm, respectively, corresponding to the methylene group located between the phenyl ring and the nitrogen atom (PhCH2NH–). Also, the multiplet attributed to the protons from the methylene group directly bonded to the nitrogen atom (PhCH2NHCH2–) appears at 2.60 ppm in 1H-NMR (Fig. 1B). Finally, after purification, the treatment with a solution hydrogen chloride resulted in the protonated systems [(OH)(OR)Ph(CH2NH2Cl)]-G1-(NMe2·HCl)2 (R = CH3 (5) R = H (6)) as water-soluble oils with good yields. Through the NMR study, it was possible to observe a deshielding of the chemical shift of the methylene groups next to the positive charged nitrogen atom (Fig. 1C).
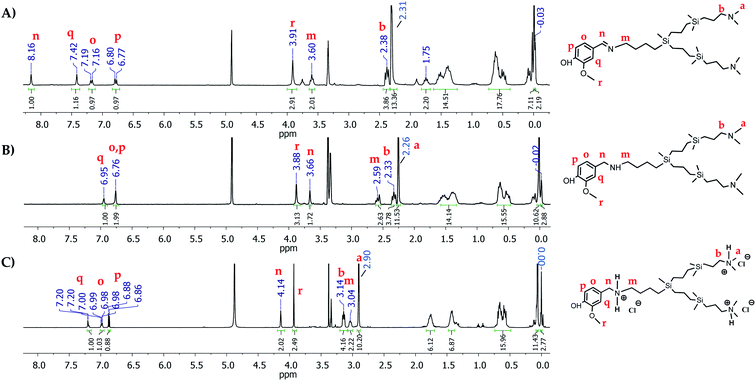 |
| Fig. 1 1H-NMR spectra in CD3OD of (A) imine [(OH)(OCH3)Ph(C N)]-G1-(NMe2)2 (1) (B) amine [(OH)(OCH3)Ph(CH2NH)]-G1-(NMe2)2 (3) (C) ammonium [(OH)(OCH3)Ph(CH2NH2Cl)]-G1-(NMe2·HCl)2 (5). | |
For the caffeic acid, the attachment was achieved through covalent amidation reaction using EDCI·HCl and HOBt as coupling reagents and DMF as a reaction solvent, leading to the obtention of the derivative [(OH)2PhCH2CH
CHC(O)NH]-G1-(NMe2)2 (7). The amide formation was confirmed by 1H-NMR and 13C-NMR, due to the presence of a signal attributed to the methylene group next to the amide fragment (–C(O)NHCH2–) at 3.31 ppm and 40.0 ppm, respectively. Moreover, in 13C-NMR it is possible to observe a signal corresponding to the carbon of the amide fragment at 169.2 ppm. The reaction with hydrogen chloride resulted in protonated system [(OH)2PhCH2CH
CHC(O)NH]-G1-(NMe2·HCl)2 (8) as a water-soluble brown oil with good yields. Once again, the positive charge provokes a deshielding of the chemical shift of protons corresponding to the methyl groups located in the periphery (N(CH3)2), as well as the methylene group –CH2(N(CH3)2), as previously observed. Fig. 2 shows the corresponding 1H-NMR, 13C-NMR, and 1H-DOSY-2D-NMR as an example for the final compound 8.
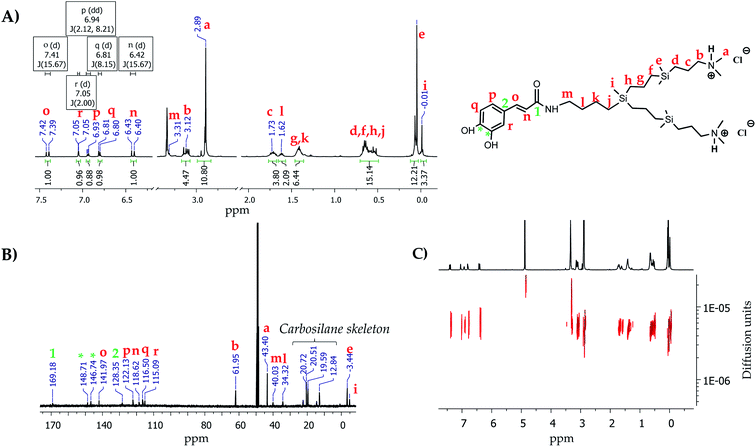 |
| Fig. 2 NMR spectra in CD3OD of the dendron [(OH)2PhCH2CH CHC(O)NH-G1-(NMe2·HCl)2] (8). (A) 1H-NMR, (B) 13C-NMR and (C) 1H-DOSY-2D-NMR. | |
The proposed structures were confirmed through mass spectrometry (ESI-TOF), observing the molecular peaks for neutral precursors (3, 4 and 7). Regarding the cationic derivatives (5, 6 and 8), it was possible to observe the molecular peak attributed to the loss of two chlorides. Unfortunately, it was not possible to characterise through this technique the imine derivatives due to their lack of stability. Elemental analysis corroborated the purity of the synthesised systems.
3.2. Antioxidant activity – spectrophotometric methods
The antioxidant activity of new derivates 5, 6 and 8, free polyphenols (II, III and IV) and the wedge [NH3Cl-G1-(NMe2·HCl)2] (V) without polyphenol have been studied from two different points of view: as IC50 (concentration of antioxidant compound that scavenges 50% of free radical DPPH) and EC50 (concentration of antioxidant compound that increases 50% of FRAP capacity) (Fig. 4A), as well as TEAC (Trolox equivalent antioxidant capacity) (Fig. 4B).
Dendritic wedge V was obtained by protonation of the precursor I with hydrogen chloride to use as a control in the antioxidant assays. Both spectrophotometric assays are based on different mechanisms: FRAP assay consists of a redox reaction based on a single electron transference mechanism (SET) in which Fe(III) is reduced to Fe(II), while DPPH is a radical scavenging method that involves the single electron and the hydrogen atom transference, SET and HAT, respectively. To carry out these experiments, a wide range of concentrations and incubation times were tested to check at which conditions the steady-state is reached (Fig. 3(A) DPPH assay and (B) FRAP assay). Interestingly, variations in activity were not as pronounced as we previously observed for the spherical polyphenolic dendrons.23 To compare these results with those previously obtained for spherical dendrimers, an incubation time of 30 minutes was selected. According to the previously reported procedure, the Trolox equivalent activity was also evaluated through the interpolation of the absorbance equivalent to the maximum activity in the Trolox standard curve for both methods.23
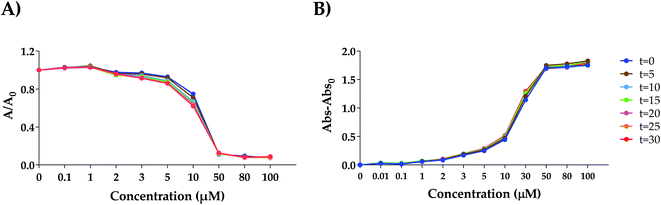 |
| Fig. 3 Study of the antioxidant activity for dendron 8 by DPPH assay (A) and FRAP assay (B) in an established range of concentrations (0–100 μM) and times (t = 0–30 min. See legend located on the right of (B), applicable for both (A) and (B). Different colors correspond to the different times). | |
In both experiments, the results obtained for the two dendrons belonging to the family of reductive amination derivates (5 and 6) highlight the importance of the number of hydroxyl groups in the antioxidant activity to observe that the vanillin derivative 5 which contains a single hydroxyl group, has the lowest activity with respect to the derivatives from protocatechuic aldehyde 6 and caffeic acid 8, as previously observed.23
On the other hand, the study of the dendrons 6 and 8 with the same number of hydroxyl groups enables us to determine the influence of the different bonds and electronic disposition on the antioxidant capacity. In this case, the similar activity observed for the dendritic wedges 6 and 8 reveals that the type of bond established between polyphenol and dendron does not affect the electronic disposition. Additionally, the comparison between the results obtained for polyphenolic dendrons and free polyphenols point that the anchoring of the polyphenol to the dendritic skeleton alters the ability of polyphenolic dendrons to donate electrons to carry out the reduction of the Fe(III) in FRAP complex compared to free polyphenols and the study of dendron V without polyphenolic in its structure confirm that the antioxidant activity is provided by the polyphenol and not by the dendritic wedge. However, from an electronic point of view, FRAP's activity was lower for the dendrons than the free polyphenols in all cases, probably since the electronic arrangement is not so favourable to carry out the redox reaction. This behaviour is in accordance with the results obtained in the TEAC assay (Fig. 4B). Regarding the analysis of the radical scavenging capacity by DPPH, it was not possible to observe any appreciable difference in the IC50 values for dendrons 6 and 8. Interestingly, the first generation dendron with unique caffeic acid at the focal point (8) exhibited an IC50 value of 13.7 μM (Figure 4A), 2.5 higher than that previously observed for the first generation carbosilane dendrimer functionalised with four caffeic acids that presented an IC50 of 5.6 μM.23 This fact might suggest a cooperative effect between the cationic dendritic wedge and the polyphenol, which probably is due to the presence of the positive charges in 8 that cause an increase in the solubility of the system in polar solvents compared with the spherical derivative thus favouring its antioxidant capacity. These results, together with the higher solubility in aqueous media of the new systems and the hydrophobicity provided by the presence of the carbosilane backbone which would allow a higher interaction with cell membranes, make these systems suitable candidates for further in vitro study for use in biomedical applications in the treatment for pathologies associated with oxidative stress or as part of a combined therapy with other chemotherapeutic agents that generate redox imbalance as a side-effect.
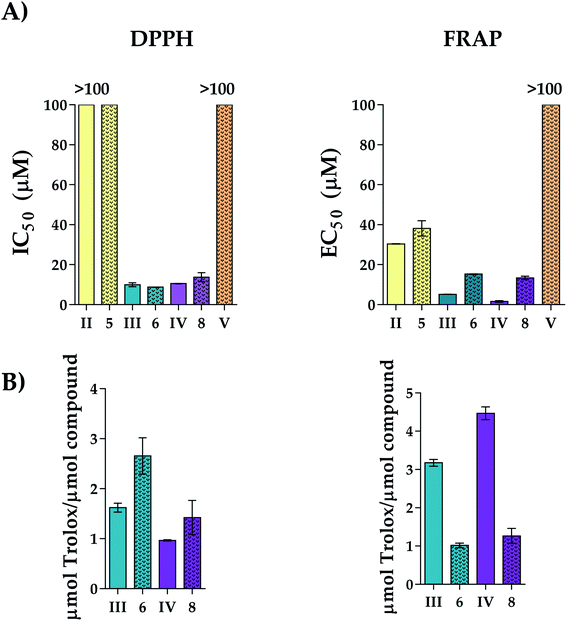 |
| Fig. 4 Antioxidant activity of the polyphenolic dendrons (5, 6 and 8), free polyphenols (II, III and IV) and control wedge (V). (A) IC50 and EC50 values for DPPH and FRAP, respectively. (B) Trolox equivalent antioxidant capacity (TEAC) of polyphenolic dendrons expressed as μmol Trolox per μmol compound. Mean values ± SEM representative of at least three independent experiments are shown. | |
4. Conclusions
The generation of reactive oxygen species (ROS) is partly responsible for the emergence of different diseases with notable morbidity and mortality. Also, ROS are one of the factors responsible for several chemotherapeutic drugs' secondary toxicity. Accordingly, the search for new antioxidants compounds that balance ROS generation and antioxidant defence systems are of great interest. In this work, we have synthesised a family of cationic carbosilane dendrons with different polyphenols such as vanillin, protocatechuic aldehyde and caffeic acid (3,4-dihydroxy-cinnamic), at the focal point where the main structural difference between these polyphenols lies in the number of hydroxyl groups and the way that the polyphenol is anchored to the dendritic platform, allowing us to determine the influence of these parameters in the antioxidant activity. We improve the water solubility as well as the bioavailability of these polyphenols by attaching them to carbosilane dendrons with dimethylammonium groups on the surface. Evaluation of the antioxidant activity of these new systems by two different spectrophotometric methods (FRAP and DPPH) confirms that conjugation of the free polyphenol's to the dendritic structure does not modify their antioxidant activity. In these studies, the number of hydroxyl groups is determinant for their antioxidant activity, discarding the derivative functionalised with vanillin (5) from our biological studies, and placing both the protocatechuic and caffeic derivatives (6 and 8) as promising compounds with similar antioxidant activities regardless of the type of bond that holds the polyphenol to the dendritic structure. These results make these systems suitable candidates for further in vitro study to determine the possible application of carbosilane dendrons functionalised with polyphenols as antioxidants agents in pathologies associated with oxidative stress.
Author contributions
Methodology, P. O. and F. J. d. l. M; investigation, N. S. O. and J. C. G.; resources, N. S. O. and J. C. G. writing—original draft preparation, P. O., N. S. O. and J. C. G.; writing—review and editing, P. O, F. J. d. l. M; visualisation, F. J. d. l. M. and P. O.; supervision, P. O, F. J. d. l. M; funding acquisition, F. J. d. l. M., and R. G.
Funding
Authors thanks funding by grants from CTQ2017-86224-P (MINECO) and project SBPLY/17/180501/000358 Junta de Comunidades de Castilla-La Mancha (JCCM), PID2019-104070RB-C22 (MICINN) consortiums IMMUNOTHERCAN-CM B2017/BMD-3733 and NANODENDMED II-CM ref. B2017/BMD-3703. CIBER-BBN is an initiative funded by the VI National R&D&i Plan 2008–2011, Iniciativa Ingenio 2010, the Consolider Program, and CIBER Actions and financed by the Instituto de Salud Carlos III with assistance from the European Regional Development Fund.
Conflicts of interest
The authors declare no conflict of interest.
References
- S. Banerjee, S. Ghosh, A. Mandal, N. Ghosh and P. C. Sil, ROS-associated immune response and metabolism: a mechanistic approach with implication of various diseases, Arch. Toxicol., 2020, 94, 2293–2317, DOI:10.1007/s00204-020-02801-7.
- S. Yang and G. Lian, ROS and diseases: role in metabolism and energy supply, Mol. Cell. Biochem., 2020, 467, 1–12, DOI:10.1007/s11010-019-03667-9.
- C. Yokoyama, Y. Sueyoshi, M. Ema, Y. Mori, K. Takaishi and H. Hisatomi, Induction of oxidative stress by anticancer drugs in the presence and absence of cells, Oncol. Lett., 2017, 14, 6066–6070, DOI:10.3892/ol.2017.6931.
- J. Dai and R. J. Mumper, Plant phenolics: extraction, analysis and their antioxidant and anticancer properties, Molecules, 2010, 15, 7313–7352 CrossRef CAS.
- F. Di Meo, V. Lemaur, J. r. m. Cornil, R. Lazzaroni, J.-L. Duroux, Y. Olivier and P. Trouillas, Free radical scavenging by natural polyphenols: atom versus electron transfer, J. Phys. Chem. A, 2013, 117, 2082–2092 CrossRef CAS.
- D. Stevenson and R. Hurst, Polyphenolic phytochemicals-just antioxidants or much more?, Cell. Mol. Life Sci., 2007, 64, 2900–2916 CrossRef CAS PubMed.
- A. Upadhyay, B. Singh Bhakuni, R. Meena and S. Kumar, Radical Chain Breaking Bis(ortho-organoselenium) Substituted Phenolic Antioxidants, Chem.–Asian J., 2021, 16, 966–973 CrossRef CAS PubMed.
- A. Upadhyay, R. Kumar Jha, M. Batabyal, T. Dutta, A. L. Koner and S. Kumar, Janus-faced oxidant and antioxidant profiles of organo diselenides, Dalton Trans., 2021, 50, 14576–14594, 10.1039/D1DT01565F.
- D. A. Pratt, G. A. DiLabio, G. Brigati, G. F. Pedulli and L. Valgimigli, 5-Pyrimidinols: Novel Chain-Breaking Antioxidants More Effective than Phenols, J. Am. Chem. Soc., 2001, 123, 4625–4626, DOI:10.1021/ja005679l.
- T.-g. Nam, C. L. Rector, H.-y. Kim, A. F. P. Sonnen, R. Meyer, W. M. Nau, J. Atkinson, J. Rintoul, D. A. Pratt and N. A. Porter, Tetrahydro-1,8-naphthyridinol Analogues of α-Tocopherol as Antioxidants in Lipid Membranes and Low-Density Lipoproteins, J. Am. Chem. Soc., 2007, 129, 10211–10219, DOI:10.1021/ja072371m.
- D. D. Milinčić, D. A. Popović, S. M. Lević, A. Ž. Kostić, Ž. L. Tešić, V. A. Nedović and M. B. Pešić, Application of polyphenol-loaded nanoparticles in food industry, Nanomaterials, 2019, 9, 1629 CrossRef PubMed.
- Y. H. Choi and H.-K. Han, Nanomedicines: current status and future perspectives in aspect of drug delivery and pharmacokinetics, Int. J. Pharm. Invest., 2018, 48, 43–60 CrossRef CAS PubMed.
- L. Cruz, N. Basílio, J. Mendoza, N. Mateus, V. de Freitas, M. H. Tawara, J. Correa and E. Fernandez-Megia, Impact of a Water-Soluble Gallic Acid-Based Dendrimer on the Color-Stabilizing Mechanisms of Anthocyanins, Chem.–Eur. J., 2019, 25, 11696–11706 CrossRef CAS PubMed.
- M. De La Fuente, M. Raviña, A. Sousa-Herves, J. Correa, R. Riguera, E. Fernandez-Megia, A. Sánchez and M. J. Alonso, Exploring the efficiency of gallic acid-based dendrimers and their block copolymers with PEG as gene carriers, Nanomedicine, 2012, 7, 1667–1681 CrossRef CAS PubMed.
- C. Y. Lee, C. N. Nanah, R. A. Held, A. R. Clark, U. G. Huynh, M. C. Maraskine, R. L. Uzarski, J. McCracken and A. Sharma, Effect of electron donating groups on polyphenol-based antioxidant dendrimers, Biochimie, 2015, 111, 125–134 CrossRef CAS PubMed.
- C. Y. Lee, A. Sharma, J. E. Cheong and J. L. Nelson, Synthesis and antioxidant properties of dendritic polyphenols, Bioorg. Med. Chem. Lett., 2009, 19, 6326–6330 CrossRef CAS PubMed.
- C. Y. Lee, A. Sharma, R. L. Uzarski, J. E. Cheong, H. Xu, R. A. Held, S. K. Upadhaya and J. L. Nelson, Potent antioxidant dendrimers lacking pro-oxidant activity, Free Radical Biol. Med., 2011, 50, 918–925 CrossRef CAS PubMed.
- A.-M. Caminade, S. Fruchon, C.-O. Turrin, M. Poupot, A. Ouali, A. Maraval, M. Garzoni, M. Maly, V. Furer and V. Kovalenko, The key role of the scaffold on the efficiency of dendrimer nanodrugs, Nat. Commun., 2015, 6, 1–11 Search PubMed.
- R. Carloni, N. Sanz del Olmo, P. Ortega, A. Fattori, R. Gómez, M. F. Ottaviani, S. García-Gallego, M. Cangiotti and F. J. de la Mata, Exploring the interactions of ruthenium(II) carbosilane metallodendrimers and precursors with model cell membranes through a dual spin-label spin-probe technique using EPR, Biomolecules, 2019, 9, 540 CrossRef PubMed.
- N. Sanz del Olmo, R. Carloni, A. M. Bajo, P. Ortega, A. Fattori, R. Gómez, M. F. Ottaviani, S. García-Gallego, M. Cangiotti and F. J. de la Mata, Insight into the antitumor activity of carbosilane Cu(II)-metallodendrimers through their interaction with biological membrane models, Nanoscale, 2019, 11, 13330–13342 RSC.
- D. Wrobel, A. Kłys, M. Ionov, P. Vitovic, I. Waczulikowa, T. Hianik, R. Gomez-Ramirez, J. de la Mata, B. Klajnert and M. Bryszewska, Cationic carbosilane dendrimers–lipid membrane interactions, Chem. Phys. Lipids, 2012, 165, 401–407 CrossRef CAS PubMed.
- G. Mencía, N. Sanz del Olmo, L. Muñoz-Moreno, M. Maroto-Diaz, R. Gómez, P. Ortega, M. J. Carmena and F. J. de la Mata, Polyphenolic carbosilane dendrimers as anticancer agents against prostate cancer, New J. Chem., 2016, 40, 10488–10497 RSC.
- N. Sanz del Olmo, C. E. Peña González, J. D. Rojas, R. Gómez, P. Ortega, A. Escarpa and F. J. de la Mata, Antioxidant and Antibacterial Properties of Carbosilane Dendrimers Functionalized with Polyphenolic Moieties, Pharmaceutics, 2020, 12, 698 CrossRef PubMed.
- T. Lozano-Cruz, P. Ortega, B. Batanero, J. L. Copa-Patiño, J. Soliveri, F. J. de la Mata and R. Gómez, Synthesis, characterization and antibacterial behavior of water-soluble carbosilane dendrons containing ferrocene at the focal point, Dalton Trans., 2015, 44, 19294–19304 RSC.
Footnote |
† Electronic supplementary information (ESI) available. See DOI: 10.1039/d1ra08224h |
|
This journal is © The Royal Society of Chemistry 2022 |