DOI:
10.1039/D1RA08088A
(Paper)
RSC Adv., 2022,
12, 4153-4161
Hollow terbium metal–organic-framework spheres: preparation and their performance in Fe3+ detection†
Received
4th November 2021
, Accepted 22nd January 2022
First published on 1st February 2022
Abstract
Hollow metal–organic framework (MOF) micro/nanostructures have been attracting a great amount of research interest in recent years. However, the synthesis of hollow metal–organic frameworks (MOFs) is a great challenge. In this paper, by using 1,3,5-benzenetricarboxylic acid (H3BTC) as the organic ligand and 2,5-thiophenedicarboxylic acid (H2TDC) as the competitive ligand and protective agent, hollow terbium MOFs (Tb-MOFs) spheres were synthesized by a one-pot solvothermal method. By comparing the morphology of Tb-MOFs in the presence and absence of H2TDC, it is found that H2TDC plays a key role in the formation of the hollow spherical structure. Single crystal analyses and element analysis confirm that H2TDC is not involved in the coordination with Tb3+. Interestingly, Tb-MOFs can be used as the luminescent probes for Fe3+ recognition in aqueous and N,N-dimethylformamide (DMF) solutions. In aqueous solution, the quenching constant (KSV) is 5.8 × 10−4 M−1, and the limit of detection (LOD) is 2.05 μM. In DMF, the KSV and LOD are 9.5 × 10−4 M−1 and 0.80 μM, respectively. The sensing mechanism is that the excitation energy absorption of Fe3+ ions reduces the energy transfer efficiency from the ligand to Tb3+ ions.
1. Introduction
Due to their enhanced surface-to-volume ratio, micro-reactor environment, high loading capacities, excellent designability and flexibility, hollow metal–organic frameworks (MOFs) have shown fascinating physicochemical properties and wide applications, especially in molecule adsorption, filtration, diffusion and transfer..1–3 Recently, several strategies have been developed to synthesize hollow MOFs, such as template-mediated assembly, interfacial synthesis, stepped dissolution-regrowth, ion-exchange reactions, chemical etching, etc.4–6 Using carboxylate modified polystyrene spheres as a hard template, hollow zeolitic imidazolate framework-8 (ZIF-8) microspheres were fabricated by electrostatic interaction.7 Choe et al. prepared single-shell and double shelled MOFs using metal organic polyhedra as a self-template and building block.8 Wang et al. synthesized a hollow zirconium-porphyrinic MOF with single atoms embedded using benzoic acid as a competitive reagent.9 By adding carbon dioxide bubbles into continuous ionic liquid to form a liquid–gas interface, hollow Zn-BTC was successfully prepared.10 Hollow MOFs can also be obtained through liquid–liquid interface and solid–liquid interface methods.11–13 Although great achievements have been achieved, the synthesis of hollow MOFs with controllable structure is still a complicated and difficult task; it is urgently needed to synthesize hollow MOFs with a simple and easy method.3,4
The Fe3+ ion is one of the essential metal ions in industrial production and biological systems; a deficiency of Fe3+ ions in the human body will cause physical problems such as anemia, liver injury, diabetes, and heart failure. But excessive Fe3+ will cause serious environmental issues.14–16 Therefore, the simple, rapid and selective detection of Fe3+ ions is meaningful to research. Due to the advantages of high sensitivity, good stability, simple operation and real-time detection, a luminescent quenching-type sensor is often considered to be an ideal choice for high-sensitivity and high-precision Fe3+ ion detection.17,18 Lanthanide metal–organic frameworks have high luminous efficiency, high color purity, large Stokes-shift, long luminescence life, and have been widely used in the selective detection of metal ions and small molecules.18–20 Thus, in this work, novel hollow spherical Tb-MOFs were prepared easily by the solvothermal method based on 1,3,5-benzenetricarboxylic acid (H3BTC) as the ligand, and 2,5-thiophenedicarboxylic acid (H2TDC) as the competitive and protective agent. The crystal structure, morphology, elemental information, thermal stability and luminescent properties of the Tb-MOFs were characterized and analyzed, and the sensing performance of Fe3+ ions is evaluated and discussed in detail.
2. Experimental section
2.1 Reagents
H3BTC (98%), H2TDC (98%) and terbium nitrate hexahydrate (Tb(NO3)3·6H2O, 99.9%) were purchased from Macklin Inc. Ferric nitrate nonaqueous (Fe(NO3)3·9H2O, A.R.) and N,N-dimethylformamide (DMF, A.R.) were obtained from Aladdin. All reagents were not further purified before use.
2.2 Instruments
The single crystal diffraction data were recorded by Bruker D8 Advance X-ray diffractometer with Ga Kα radiation. Powder X-ray diffraction (XRD) patterns were measured on a PANalytical Empyrean Series 2 diffractometer (Cu Kα) in the 5–50° angular range with a step of 0.026° and a scanning speed of 7° per minute. Thermogravimetric analysis (TGA) was performed under a flow of N2 in the temperature range 10–700 °C at a heating rate of 10 °C min−1 on a TGA Q-50 thermogravimetric analyzer. The UV-Vis absorption and luminescence spectra were determined on a UV 3600 spectrometer (Shimadzu, Japan) and an F-4600 fluorescence spectrometer (Shimadzu, Japan), respectively. The emission decay processes and quantum yields were recorded on an FLS980 spectrofluorometer system (Edinburgh, UK). Morphology analysis was obtained using a field emission scanning electron microscope (FE-SEM, JSM-7800F, Japan, LaB6, ZEISS, Germany). The X-ray photoelectron spectroscopy (XPS) was collected on an XPS-Theta probe instrument (Escalab250Xi, Thermo Fisher, USA). The high-resolution transmission electron microscope (HRTEM) with high-angle annular dark field (HAADF, ThermoFisher Scientific Talos F200S, Czech Republic) was used to analyze the element distribution and composition of the Tb-MOFs. Fourier-transform infrared spectroscopy (FTIR) measurements were recorded using an FTIR spectrometer (iS50, Nicolet, USA).
2.3 Synthesis of Tb-MOFs
1 mmol (0.453 g) Tb(NO3)3·6H2O, a certain proportion of H3BTC and H2TDC (Table S4, ESI†) were dissolved in 10 ml DMF, respectively. The above three solutions were fully mixed and transferred to a Teflon-lined reactor and heated at 150 °C for 72 h. The reaction products were centrifuged and cleaned with DMF, and the Tb-MOFs were obtained after drying in a vacuum drying oven at 60 °C.
3. Results and discussion
3.1 Structure of Tb-MOFs
The single-crystal X-ray diffraction analysis results of Tb-MOFs are shown in Tables S1–S3, ESI.† Tb-MOFs are assigned to the monoclinic space group C2/c with the lattice parameters of a = 18.6318(8) Å, b = 18.6318(8) Å, c = 19.7386(8) Å. Both H3BTC and DMF are participated in the coordination, forming a three-dimensional MOFs structure with Tb3+ as the center, while H2TDC does not coordinate with Tb3+.
The synthesis flow chart of Tb-MOFs is shown in Fig. S1, ESI.† Hollow sphere Tb-MOFs are synthesized using H3BTC and Tb3+ in the DMF system under the action of H2TDC. In this process, both H3BTC and DMF participated in the synthesis of Tb-MOFs structure. The coordination environment of Tb3+ is shown in Fig. 1a, Tb01 is 8-coordinated environment consisting of 6 oxygen atoms from H3BTC (H3BTC1, H3BTC2, H3BTC3) anion and 2 oxygen atoms from DMF (DMF1, DMF2, DMF3). As shown in Fig. S2, ESI,† O002 and O005 from H3BTC1 are coordinated with Tb3+ by asymmetric chelation, the Tb–O bond lengths are 2.443(4) Å and 2.399(4) Å, respectively, and the bond angle of Tb–O–Tb is 54.30(12)°. O009 and O006 in H3BTC2 are coordinated with Tb3+ by symmetric chelation, the Tb–O bond lengths are 2.413(4) Å and 2.415(4) Å, respectively, and the bond angle of Tb–O–Tb is 53.61(14)°. O003 and O004 in H3BTC3 and H3BTC4 coordinate with Tb3+ by bridging, and the Tb–O bond lengths are 2.330(4) Å and 2.283(4) Å, respectively. O007 and O008 monodentate coordination Tb3+ from DMF1 and DMF2, the bond lengths of Tb–O are 2.379(5) Å and 2.354(4) Å, respectively. In the whole coordination structure, the bond angle of O–Tb–O ranges from 53.61(14)° to 156.56(14)°.
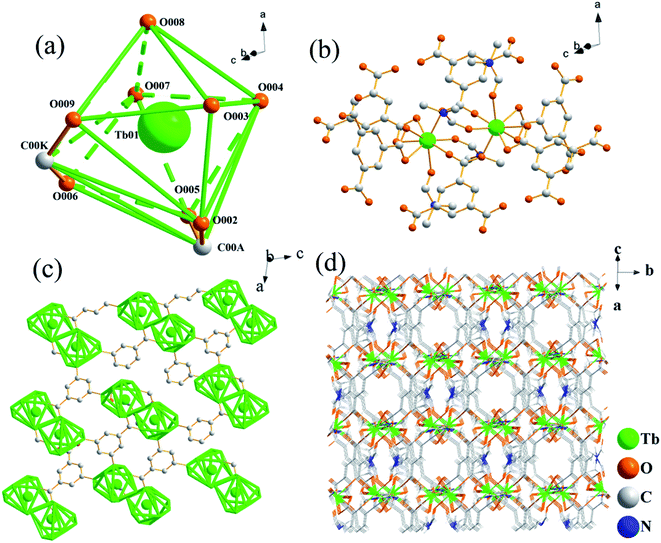 |
| Fig. 1 (a) Coordination environment of Tb atom. (b) Single crystal structure of Tb-MOFs. (c) Two-dimensional frame diagram of Tb-MOFs. (d) Three-dimensional frame diagram of Tb-MOFs. | |
So each local asymmetric unit in the cell contains one Tb3+, four H3BTC anions and two DMF molecules (Fig. 1b). Each structural unit cell contains two local asymmetric units, each local asymmetric unit contains 1 Tb3+, four H3BTC anions and two DMF molecules. Each of the two asymmetric units forms a Tb binuclear structure. The chain connection between the two dual-core structures forms a two-dimensional planar structure (Fig. 1c). The two-dimensional plane structure forms a stable three-dimensional frame structure with micropores through the cross-layer connection form (Fig. 1d).
3.2 XRD, TG, SEM, FTIR and element analyses
The XRD pattern of Tb-MOFs (CCDC 2082489, Fig. S3, ESI†) shows that Tb-MOFs have good crystallinity, and the TG curve (Fig. S4, ESI†) indicates that Tb-MOFs have good thermal stability. The comparative morphologies of Tb-MOFs with and without H2TDC are shown in Fig. 2a and b. It is found that Tb-MOFs without H2TDC are self-assembled into stable rod bundles, while the addition of H2TDC leads to forming the hollow sphere structure. The hollow structure is very stable and ultrasonic treatment for 30 minutes can't damage the hollow structure. SEM morphology of Tb-MOFs prepared with different H3BTC/H2TDC ratios (Fig. S5, ESI†) indicates that the hollow sphere structure is not changed by different H3BTC/H2TDC ratios. These results indicate that H2TDC is not only a competitive reagent but also a protective agent. High-angle annular darkfield (HAADF) and element mappings (C, O, N and Tb) analysis of Tb-MOFs are respectively shown in Fig. 2c–g, which indicate that these elements are distributed uniformly throughout the whole sphere. The Tb-MOFs are consistent with that of the single crystal. The shadow in the sphere center of Tb element indicates that Tb-MOFs are hollow spheres (Fig. 2g). The line scanning profile of Tb element distribution (Fig. S6, ESI†) indicates that there are fewer elements in the center of the sphere, which further confirms that it is a hollow spherical structure.
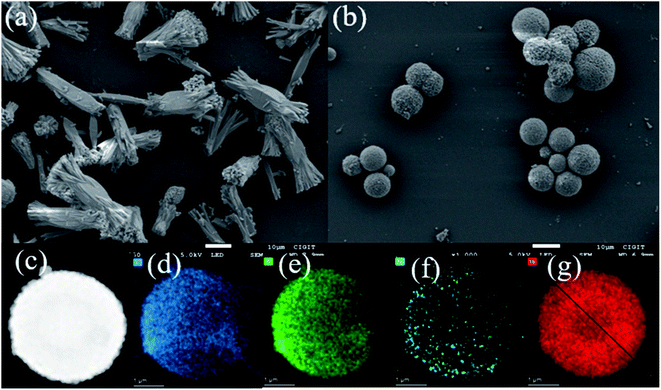 |
| Fig. 2 (a) SEM image of Tb-MOFs without H2TDC. (b) SEM image of Tb-MOFs with H2TDC. (c) HAADF image of Tb-MOF with H2TDC. (d)–(g) mapping images of C, O, N and Tb element, respectively. | |
As shown in Fig. 3, Tables S5 and S6,† no sulfur can be detected in all samples during the preparation process and the final sample, which confirms that H2TDC was not involved in the coordination with Tb3+. So the formation mechanism of hollow spherical Tb-MOF is deduced as follows. In the initial stage, H2TDC serves as the coordination competitor. Due to the coordination ability between H3BTC and Tb3+ being stronger than that of H2TDC, the surface-energy-driven mechanism may be responsible for the crystallization process,21 forming solid spheres stacked by Tb-BTC nanoblocks (1–2 h in Fig. 3a). In the second stage, many defects exist in the inner region of the solid spheres, such as incomplete coordination metal nodes and grain boundaries, which makes the solid spheres unstable. In addition, the concentration of H3BTC decreases due to the participation in coordination, while the concentration of H2TDC does not change. The rich carboxyl groups on H2TDC surface can provide protons and participate in competitive chelation with Tb3+, partially destroying the formed coordination bond. With the dissolution of the original unstable coordination and the formation of new coordination, the solid sphere structure collapsed (3 h in Fig. 3a) to form the hollow structure (4–6 h in Fig. 3a). This deduction is proved by the elemental data in Table S5, ESI.† In the third stage, the protons released by H2TDC not only penetrate the hollow core but also cover the surface of the spheres, shielding the formed coordination bonds. Finally, a stable hollow spherical structure is formed (Fig. S5†). Therefore, H2TDC serves as a competitive ligand and protective agent during the synthesis of Tb-MOF.
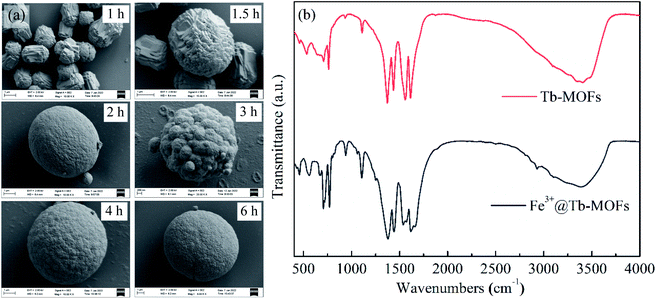 |
| Fig. 3 (a) SEM images for 1 h, 1.5 h, 2 h, 3 h, 4 h, 6 h during Tb-MOFs preparation. The synthesis process of Tb-MOFs hollow spheres. (b) FTIR spectra of Tb-MOFs and Fe3+@Tb-MOFs. | |
The FTIR spectrum of the Tb-MOFs is shown in Fig. 3b. The characteristic band 650–900 cm−1 was due to the out-plane bending vibration of C–H in benzene ring of Tb-MOFs. The peak at 918 cm−1 can be attributed to the out-plane bending vibration of hydroxyl group. Peaks at 1626 cm−1 and 1434 cm−1 (or 1568 cm−1 and 1375 cm−1) are attributed to the asymmetric and symmetric stretch vibration of COO− group (νas(COO−) and νs(COO−)), respectively. These results indicate that the carboxylic groups of H3BTC are dehydrogenated and then coordinated with Tb3+ ions in Tb-MOF.22 The difference between νas(COO−) and νs(COO−) value is 192 cm−1 (or 193 cm−1), which implies that three coordinate modes, monodentate, chelation and bridging exist in the synthesized Tb-MOF.22
3.3 Luminescence properties
The emission spectra of Tb-MOFs with different ratios of H3BTC and H2TDC using the excitation wavelength of 320 nm are shown in Fig. S7, ESI.† In the luminescence process, H3BTC serves a sensitizer to enhance the luminescence of Tb-MOFs. When H3BTC/H2TDC ratio is 1
:
1, the synthesized Tb-MOFs have fewer coordination defects, H3BTC can transfer the absorbed energy to Tb-MOFs easily which promotes the luminescence of Tb-MOFs. While H3BTC/H2TDC ratios are other values, some uncoordinated Tb3+ and carboxyl groups hinder the energy transfer from H3BTC to Tb3+. Thus, Tb-MOFs with the ratio of H3BTC/H3TDC of 1
:
1 have the strongest luminescence intensity. Four emission peaks at 488 nm, 545 nm, 584 nm and 621 nm are corresponding to the 5D4 → 7FJ (J = 6, 5, 4, 3) transitions of Tb3+, respectively. Therefore, Tb-MOFs with the H3BTC/H2TDC ratio of 1
:
1 are synthesized for further exploration.
3.4 Sensing performance of Tb-MOFs to Fe3+
2 mg Tb-MOFs was, respectively, mixed evenly in 1 × 10−3 mol L−1 M(NO3)n (M = Ca2+, Cr3+, NH4+, Ba2+, Zn2+, Mn2+, Co2+, Bi3+, Ag+, Cu2+, Fe2+, Fe3+) aqueous solutions, and then the suspended solutions of different metal ions were obtained. Before photoluminescence (PL) performance measurements, the suspensions were treated with ultrasonication for 10 minutes. The PL properties are recorded and compared in Fig. 4a. Obviously, expected for Fe3+ solution, other cations display a little influence on the emission spectra of Tb-MOFs, while the emission intensities decreased rapidly in Fe3+ solution. Then, when multiple metal ions exist in aqueous solution at the same time, the selectivity to Fe3+ has been investigated (Fig. S9, ESI†). When there are 1, 3, 5, 7, and 9 metal ions in an aqueous solution at the same time, it still has good selectivity to Fe3+. Therefore, Tb-MOFs can be used as a luminescent sensor for Fe3+ ions detection.
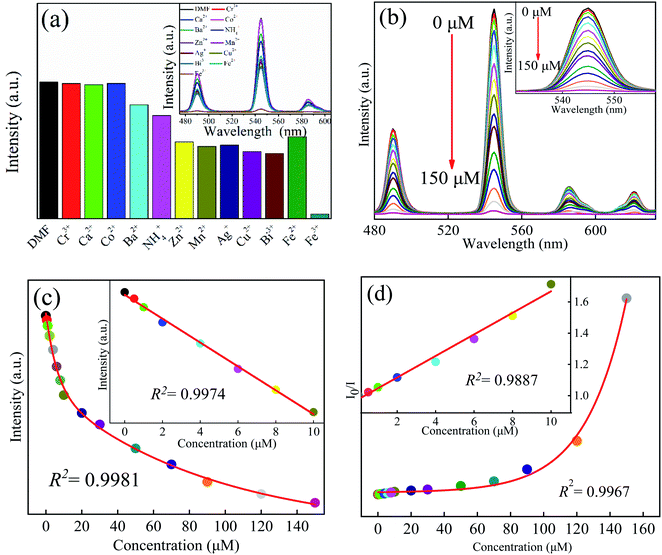 |
| Fig. 4 (a) Histogram of Tb-MOFs luminescence intensities in aqueous solutions with different metal ions (at 545 nm), and the inset is emission spectra. (b) Emission spectra of Tb-MOFs in various concentrations of Fe3+ solutions, and the inset is emission spectra near 545 nm. (c) Fitting curves of luminescence intensities of Tb-MOFs versus Fe3+ concentrations (0–150 μM), and the inset is luminescence intensities versus low Fe3+ concentration (0–10 μM). (d) Stern–Volmer fitting curve (Fe3+ concentration: 0–150 μM), and the inset is Stern–Volmer fitting curve at low (Fe3+ concentration: 0–10 μM). | |
To clarify the quenching behaviors of Tb-MOFs to Fe3+ ions in detail, the concentration-dependent emission measurements were carried out (Fig. 4b). With the increasing concentration of Fe3+ ions, the luminescence intensities of Tb-MOFs gradually decreased, when the concentration of Fe3+ ions is 150 μM, the luminescence is almost completely quenched.
The fitting curve between the luminescence intensity of Tb-MOFs at 545 nm versus the concentration of Fe3+ is shown in Fig. 4c. With the increase of Fe3+ concentration in the range of 0–150 μM, the luminescence intensities decrease exponentially which satisfies the functional relationship y = 526.8
exp(−x/6.3) + 1014.5
exp(−x/89.9) − 176.4, and the fitting parameter R2 = 0.9981. The inset shows that there is a good linear relationship between the luminescence intensities and the Fe3+ concentrations (0–10 μM), which satisfies y = 1335.7 − 55.8x with the linearity of R2 = 0.9974. The limit of detection (LOD) of Tb-MOFs for Fe3+ can be calculated by,23
|
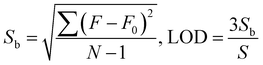 | (1) |
where
N is the number of blank groups measured without Fe
3+ (
N = 20),
F0 is the luminous intensity of the sample in aqueous solution,
F is the average value of
F0, and
Sb (
Sb = 49.7) is the standard deviation of 20 blank groups,
S is the slope of the curve in the Fe
3+ concentration range of 0–10 μM in the inset of
Fig. 4c (
S = 55.8), substituting the above values to calculate the detection limit of Fe
3+ for Tb-MOFs samples in aqueous solution is about 2.05 μM. Further, the quenching efficiency of Tb-MOFs to Fe
3+ can be evaluated by the Stern–Volmer
24 equation,
|
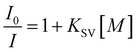 | (2) |
where
I0 and
I are the relative PL intensities before and after incorporation of the analyte, [
M] is the molar concentration of the Fe
3+, and
KSV is the Stern–Volmer quenching constant. As illustrated in inserted
Fig. 4d, the Stern–Volmer quenching curve for Fe
3+ shows a good linear relationship
y = 0.98 + 0.07
x at low concentration (0–10 μM) with a linear fit correlation coefficient of 0.9887. From the inset of
Fig. 4d, the
KSV (5.8 × 10
−4 M
−1) value can be obtained, suggesting a strong quenching effect of Fe
3+ on Tb-MOFs. However, the increase in concentration (10–150 μM) leads to the curve deviation from the linear relation (
Fig. 4d), and a good exponential relationship
y = 0.11
![[thin space (1/6-em)]](https://www.rsc.org/images/entities/char_2009.gif)
exp(−
x/24.61)+1.49 can be obtained with the fitting parameter of
R2 = 0.9967.
In addition, the sensing properties of Tb-MOFs to different metal ions in DMF solvents were also evaluated. The research method is the same as those in aqueous case. Tb-MOFs still have good selectivity to Fe3+ in the DMF system (Fig. S9, ESI†). Continue to explore the sensitivity test of Tb-MOFs to Fe3+ in DMF (Fig. S10, ESI†). Interestingly, Tb-MOFs also show a good specific recognition ability to Fe3+. The LOD is about 0.80 μM, and the Stern–Volmer quenching constant KSV is 9.5 × 10−4 M−1. These results indicate that Tb-MOFs can act as an excellent luminescent sensing material for Fe3+ ion detection.
In general, the luminescence quenching mechanism of the MOFs can be mainly summarized as three kinds: ion exchange, structural collapse or change, photon absorption competition between adsorbed ions and MOFs,25 In order to fully explain the luminescent quenching mechanism of Fe3+ to Tb-MOFs, the following four measurements are carried out. First, the XRD patterns of Tb-MOFs and Fe3+@Tb-MOFs measurement results are shown in Fig. S3, ESI,† the Tb-MOFs and Fe3+@Tb-MOFs display similar XRD pattern characteristics, which excludes the possibility of MOFs skeleton collapse or change. Second, before and after Fe3+ adsorption, XPS curves of the Tb-MOFs and Fe3+@Tb-MOFs (Fig. 5a) show that no obvious variation occurs in the positions of the element binding energy, only a weak characteristic peak of Fe3+ (710.8 eV) appears in the XPS curve of Fe3+@Tb-MOFs. The binding energy positions of Tb 3d remain unchanged (Fig. 5b), which indicates that the coordination environment of Tb atom does not change before and after adsorption of Fe3+, and there is no ion exchange between Fe3+ and Tb3+. Broadening and splitting of carboxyl characteristic peaks in FTIR spectrum of Fe3+@Tb-MOF (Fig. 3b) proves that Fe3+ affected the coordination environment of the carboxyl group. Third, the UV-Vis absorption spectrum of Fe3+ and the excitation spectrum of Tb-MOFs are shown in Fig. 5c, the major degree overlap demonstrates that the excitation energy can be effectively absorbed by Fe3+, and the energy transfer efficiency from organic ligands to Tb3+ ions will be reduced and the luminescence quenching occurs.26,27 In Fig. 5d, the luminescence lifetime of Tb-MOFs is about 0.65 ms, and that of Fe3+@Tb-MOFs is about 0.49 ms, the reduced lifetime further confirms the existence of the energy transfer in Fe3+@Tb-MOFs.28,29 These results indicate that the co-existence of absorption competition and energy transfer should be responsible for the quenching mechanism.
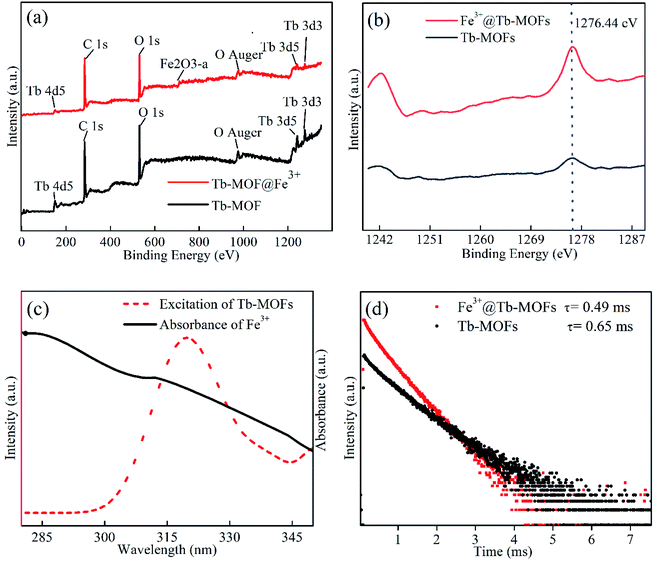 |
| Fig. 5 (a) XPS spectra of Tb-MOFs and Fe3+@Tb-MOFs. (b) Tb 3d XPS curves of Tb-MOFs and Tb–Fe3+@Tb-MOFs. (c) UV-Vis spectrum of Fe3+ and excitation spectrum of Tb-MOFs, (d) Luminescence lifetime of Tb-MOFs and Fe3+@Tb-MOFs. | |
Due to the hollow spherical structure, the large specific surface area of Tb-MOFs, the adsorption performance of Tb-MOFs to Fe3+ are also evaluated. 500 μM Fe3+ aqueous solution was added to the aqueous suspension of Tb-MOFs, and the results are shown in Fig. 6a. After Fe3+ was added to Tb-MOFs suspension, the luminescence of Tb-MOFs was quenched, the original yellow Fe3+ solution turned into a colorless transparent solution (Fig. 6b), and the original white Tb-MOFs powder changed into yellow (Fig. 6c). Combined with the above quenching mechanism analysis, it can be concluded that the hollow Tb-MOFs can not only adsorb Fe3+, but also can capture it, which provides a new platform for the adsorption and removal of Fe3+ in aqueous and DMF solutions.
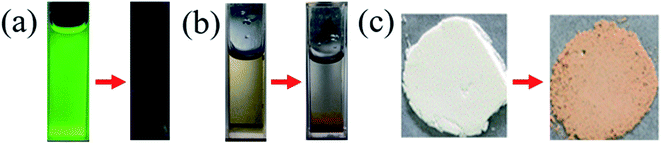 |
| Fig. 6 (a) Photographs of Tb-MOFs suspension (left) and Fe3+ (right) under 365 nm illumination. (b) Photographs of Fe3+ before (left) and after (right) addition of Tb-MOFs. (c) Photographs of Tb-MOFs powder before (left) and after (right) adsorption of Fe3+. | |
For a clearer comparison between this work and related researches, the Fe3+ sensing performance of Tb3+ based MOFs is shown in Table 1. The results indicate that the Fe3+ sensing performance of the Tb-MOFs in this work is better than most related reports.30–34
Table 1 Comparisons of the sensing parameters with other Tb3+ based MOF for Fe3+ sensing
MOF |
KSV (M−1) |
LOD (μM) |
Detection range (μM) |
Ref. |
[Tb(cpia)(H2O)2]n·nH2O |
1.2 × 104 |
2.06 |
0–120 |
30 |
Tb3+ with H4ddb |
7.64 × 104 |
0.936 |
33–330 |
31 |
[Tb(C5H7O4)(C5H6O4)(H2O)2]n |
8.07 × 104 |
— |
0–100 |
32 |
H3O[Tb-(L-N)(H2O)2]·2H2O |
7.93 × 103 |
— |
0–250 |
33 |
[Tb3(L-)2(HCOO)(H2O)4]·6H2O |
1.39 × 104 |
— |
0–400 |
[Tb(tftba)1.5(phen)(H2O)]n |
4.043 × 104 |
12.7 |
0–800 |
34 |
Tb-MOF |
5.8 × 104 |
2.05 in water |
0–150 |
This work |
9.5 × 104 |
0.8 in DMF |
0–150 |
4. Conclusion
Hollow spherical Tb-MOFs were synthesized under one-pot solvothermal method by using H2TDC as a competitive and protective agent. Tb-MOFs can be used to detect Fe3+ by the luminescence quenching effect with excellent selectivity and sensitivity. The LOD and KSV quenching constant of Tb-MOF for Fe3+ in aqueous solution are 2.05 μM and 5.8 × 10−4 M−1, respectively. In DMF, the KSV and LOD are 9.5 × 10−4 M−1 and 0.80 μM, respectively. More importantly, this work extends the synthetic strategy and methodic versatility for further development of hollow MOFs, and this method has the advantages of simple operation, mild conditions, high yield, uniform morphology and good stability, and can be applied to trace Fe3+ detection.
Conflicts of interest
There are no conflicts to declare.
Acknowledgements
This work was supported by the National Natural Science Foundation of China (51574054), Chongqing Municipal Education Commission (KJZD-M201901102), Chongqing Science and Technology Bureau (cstc2021jcyj-msxmX0493, CSTCCXLJRC201905), the Venture & Innovation Support Program for Chongqing Overseas Returnees (Cx2019092), and Guangzhou Science and Technology Project (202002030053).
References
- Z. Wang, Y. L. Yang and D. Wang, When hollow multishelled structures (HoMSs) meet metal–organic frameworks (MOFs), Chem. Sci., 2020, 11, 5359–5368 RSC.
- J. M. Zhou, W. Shi, H. M. Li, H. Li and P. Cheng, Experimental studies and mechanism analysis of high-sensitivity luminescent sensing of pollutional small molecules and ions in Ln4O4 cluster based microporous metal–organic frameworks, J. Phys. Chem. C, 2014, 118, 416–426 CrossRef CAS.
- F. N. Al-Rowaili, A. Jamal, M. S. B. Shammakh and A. Rana, A review on recent advances for electrochemical reduction of carbon dioxide to methanol using MOFs and non-MOFs catalysts; challenges and future prospects, ACS Sustainable Chem. Eng., 2018, 6, 15895–15914 CrossRef CAS.
- T. Qiu, S. Gao, Z. Liang, D. Wang, H. Tabassum, R. Zhong and R. Zou, Pristine hollow metalorganic frameworks: design, synthesis and application, Angew. Chem., Int. Ed., 2021, 60, 17314–17336 CrossRef CAS PubMed.
- J. Zhang, H. Lu, L. Zhang, D. Leng, Y. Zhang, W. Wang, Y. Gao, H. Lu and G. Zhu, Metal–organic framework-derived ZnO hollow nanocages functionalized with nanoscale Ag catalysts for enhanced ethanol sensing properties, Sens. Actuators, B, 2019, 291, 458–469 CrossRef CAS.
- W. Liu, J. Huang, Q. Yang, S. Wang, X. Sun, W. Zhang, J. Liu and F. Huo, Multi-shelled hollow metal–organic frameworks, Angew. Chem., Int. Ed., 2017, 56, 5512–5516 CrossRef CAS PubMed.
- H. Lee, W. Cho and M. Oh, Advanced fabrication of metal–organic frameworks: template-directed formation of polystyrene@ZIF-8 core–shell and hollow ZIF-8 microspheres, Chem. Commun., 2012, 48, 221–223 RSC.
- J. Lee, J. Kwak and W. Choe, Evolution of form in metal–organic frameworks, Nat. Commun., 2017, 8, 1–8 CrossRef PubMed.
- T. He, S. Chen, B. Ni, Y. Gong, Z. Wu, L. Song, L. Gu, W. Hu and X. Wang, Zirconium–porphyrin-based metal–organic framework hollow nanotubes for immobilization of noble-metal single atoms, Angew. Chem., Int. Ed., 2018, 57, 3551–3556 CrossRef.
- L. Peng, J. Zhang, J. Li, B. Han, Z. Xue, B. Zhang, J. Shi and G. Yang, Hollow metal–organic framework polyhedra synthesized by a CO2-ionic liquid interfacial templating route, J. Colloid Interface Sci., 2014, 416, 198–204 CrossRef CAS PubMed.
- G. Y. Jeong, R. Ricco, K. Liang, J. Ludwig, J. O. Kim, P. Falcaro and D. P. Kim, Bioactive MIL-88A framework hollow spheres via interfacial reaction in-droplet microfluidics for enzyme and nanoparticle encapsulation, Chem. Mater., 2015, 27, 7903–7909 CrossRef CAS.
- L. Yu, J. F. Yang and X. W. Lou, Formation of CoS2 nanobubble hollow prisms for highly reversible lithium storage, Angew. Chem., Int. Ed., 2016, 55, 1433–7851 Search PubMed.
- X. Zhang, C. Y. Chuah, P. Dong, Y. Cha and M. K. Song, Hierarchically porous Co-MOF-74 hollow nanorods for enhanced dynamic CO2 separation, ACS Appl. Mater. Interfaces, 2018, 10, 43316–43322 CrossRef CAS PubMed.
- H. H. Yu, M. Y. Fan, Q. Liu, Z. M. Su, X. Li, Q. Q. Pan and X. L. Hu, Two highly water-stable imidazole-based Ln-MOFs for sensing Fe3+, Cr2O72−/CrO42− in a water environment, Inorg. Chem., 2020, 59, 2005–2010 CrossRef CAS PubMed.
- R. C. Geng, H. X. Tang, Q. J. Ma, L. P. Liu, W. S. Feng and Z. J. Zhang, Bimetallic Ag/Zn-ZIF-8: An efficient and sensitive probe for Fe3+ and Cu2+ detection, Colloids Surf., A, 2022, 632, 127755 CrossRef CAS.
- L. Guo, Y. Liu, R. M. Kong, G. Chen, Z. Liu, F. L. Qu, L. Xia and W. H. Tan, A metal–organic framework as selectivity regulator for Fe3+ and ascorbic acid detection, Anal. Chem., 2019, 91, 12453–12460 CrossRef CAS PubMed.
- J. H. Wang, M. N. Li, S. Yan, Y. Zhang, C. C. Liang, X. M. Zhang and Y. B. Zhang, Modulator-induced Zr-MOFs diversification and investigation of their properties in gas sorption and Fe3+ ion sensing, Inorg. Chem., 2020, 59, 2961–2968 CrossRef CAS PubMed.
- Y. M. Jo, K. Lim, H. J. Choi, J. W. Yoon, S. Y. Kim and J. H. Lee, 2D metal–organic framework derived co-loading of Co3O4 and PdO nanocatalysts on In2O3 hollow spheres for tailored design of high-performance breath acetone sensors, Sens. Actuators, B, 2020, 325, 128821 CrossRef CAS.
- S. W. Sun, J. Z. Wang, G. N. Zhang and Z. L. Liu, A luminescent terbium MOF containing uncoordinated carboxyl groups exhibits highly selective sensing for Fe3+ ions, RSC Adv., 2014, 4, 55252–55255 RSC.
- R. j. F. Bogale, Y. Z. Chen, J. W. Ye, Y. Y. Yang, A. Rauf, L. Y. Duan, P. Tian and G. L. Ning, Highly selective and sensitive detection of 4-nitrophenol and Fe3+ ion based on a luminescent layered terbium(III) coordination polymer, Sens. Actuators, B, 2017, 245, 171–178 CrossRef CAS.
- Z. Zhang, Y. Chen, X. Xu, J. Zhang, G. Xiang, W. He and X. Wang, Well-defined metal–organic framework hollow nanocages, Angew. Chem., Int. Ed., 2014, 126, 439–443 CrossRef.
- X. L. Zhou, J. C. Dong, Y. H. Zhu, L. M. Liu, Y. Jiao, H. Li, Y. Han, K. Davey, Q. Xu, Y. Zheng and S. Z. Qiao, Molecular scalpel to chemically cleave metal–organic frameworks for induced phase transition, J. Am. Chem. Soc., 2021, 143, 6681–6690 CrossRef CAS PubMed.
- J. Zhou, H. Li, H. Zhang, H. Li, W. Shi and P. Cheng, A bimetallic lanthanide metal–organic material as a self-calibrating color-gradient luminescent sensor, Adv. Mater., 2015, 27, 7072–7077 CrossRef CAS PubMed.
- J. T. Wang, T. F. Xia, X. Zhang, Q. Zhang, Y. J. Cui, Y. Yang and G. D. Qian, A turn-on fluorescent probe for Cd2+ detection in aqueous environments based on an imine functionalized nanoscale metal–organic framework, Chem.–Eur. J., 2017, 7, 54892–54897 CAS.
- Q. S. Zhang, J. Wang, Y. A. M. Kirillov, W. Dou, C. Xu, C. L. Xu, L. Z. Yang, R. Fang and W. S. Liu, A superior luminescent metal–organic framework sensor for sensing trace Al3+ and picric acid via disparate charge transfer behaviors, ACS Appl. Mater. Interfaces, 2018, 10, 23976–23986 CrossRef CAS PubMed.
- Y. Su, J. Yu, Y. Li, S. F. Z. Phua, G. Liu, W. Q. Lim, X. Yang, R. Ganguly, C. Dang, C. Yang and Y. Zhao, Versatile bimetallic lanthanide metal–organic frameworks for tunable emission and efficient fluorescence sensing, Commun. Chem., 2018, 12, 1–13 Search PubMed.
- K. Fan, S. S. Bao, W. X. Nie, C. H. Liao and L. M. Zheng, Iridium(III)-based metal–organic frameworks as multiresponsive luminescent sensors for Fe3+, Cr2O72−, and ATP2−in aqueous media, Inorg. Chem., 2018, 57, 1079–1089 CrossRef CAS PubMed.
- L. L. Ren, Y. Y. Cui, A. L. Cheng and E. Q. Gao, Water-stable lanthanide-based metal–organic frameworks for rapid and sensitive detection of nitrobenzene derivatives, J. Solid State Chem., 2019, 270, 463–469 CrossRef CAS.
- M. Xiao and P. R. Selvin, Quantum yields of luminescent lanthanide chelates and far-red dyes measured by resonance energy transfer, J. Am. Chem. Soc., 2001, 123, 7067–7073 CrossRef CAS PubMed.
- J. F. Guo, M. Y. Zhang, Q. Z. Guo, G. P. Yan and L. J. Liu, Highly stable terbium(III)-based metal–organic framework for the detection of m-dinitroaromatics and Fe3+ in water, Inorg. Chim. Acta, 2021, 525, 120454 CrossRef CAS.
- C. X. Qi, Y. B. Xu, H. Li, X. B. Chen, L. Xu and B. Liu, A highly sensitive and selective turn-off fluorescence sensor for Fe3+ detection based on a terbium metal–organic framework, J. Solid State Chem., 2021, 294, 121835 CrossRef CAS.
- S. Hussain, X. N. Chen, W. T. A. Harrison, M. R. J. Elsegood, S. Ahmad, S. J. Li, S. Muhammad and D. Awoyelu, Synthesis, crystal structures and photoluminescent properties of one-dimensional europium(III)- and terbium(III)-glutarate coordination polymers, and their applications for the sensing of Fe3+ and nitroaromatics, Front. Chem., 2019, 7, 728 CrossRef CAS PubMed.
- W. Wang, N. Gong, H. Yin, B. Zhang, P. Y. Guo, B. Liu and Y. Y. Wang, Two stable terbium–organic frameworks based on predesigned functionalized ligands: selective sensing of Fe3+ ions and C2H2/CH4 separation, Inorg. Chem., 2019, 58, 10295–10303 CrossRef CAS PubMed.
- H. H. Yu, J. Q. Chi, Z. M. Su, X. Li, J. Sun, C. Zhou, X. L. Hu and Q. Liu, A water-stable terbium metal–organic framework with functionalized ligands for the detection of Fe3+ and Cr2O72− ions in water and picric acid in seawater, CrystEngComm, 2020, 22, 3638–3643 RSC.
Footnote |
† Electronic supplementary information (ESI) available: Crystal data, powder X-ray diffraction patterns (PXRD), TGA curves, SEM images, emission spectra and sensing performance (PDF). CCDC 2082489. For ESI and crystallographic data in CIF or other electronic format see DOI: 10.1039/d1ra08088a |
|
This journal is © The Royal Society of Chemistry 2022 |