DOI:
10.1039/D1RA08051B
(Paper)
RSC Adv., 2022,
12, 389-394
Li@C60 thin films: characterization and nonlinear optical properties†
Received
2nd November 2021
, Accepted 15th December 2021
First published on 21st December 2021
Abstract
Organic materials have attracted considerable attention in nonlinear optical (NLO) applications as they have several advantages over inorganic materials, including high NLO response, and fast response time as well as low-cost and easy fabrication. Lithium-containing C60 (Li@C60) is promising for NLO over other organic materials because of its strong NLO response proven by theoretical and experimental studies. However, the low purity of Li@C60 has been a bottleneck for applications in the fields of solar cells, electronics and optics. In 2010, highly purified Li@C60 was finally obtained, encouraging further studies. In this study, we demonstrate a facile method to fabricate thin films of Li@C60 and their strong NLO potential for high harmonic generation by showing its comparatively strong emission of degenerate-six-wave mixing, a fifth-order NLO effect.
Introduction
Nonlinear optics (NLO) provides a variety of applications such as optical signal processing, ultrafast switches, sensors and creation of correlated photon pairs.1–5 In order to observe NLO effects, strong electric fields, typically from ultrashort pulsed lasers, are required. Aside from this condition, the choice of material also plays a deciding role as the optical nonlinearities of the material determine the size of the NLO response. Current materials used in NLO are often inorganic like beta-barium-borate (BBO) or gallium arsenide (GaAs).6,7 These materials have some advantages, such as environmental stability, mechanical strength and a high stability over a large temperature range.6,7 On the other hand, organic materials are typically superior in terms of having large nonlinear properties, high optical damage thresholds, fast response times and flexibility in terms of engineering their structures.6,7 C60 has been suggested as a promising material for applications in high harmonic generation and ultra-fast optics.8,9 Fullerenes allow a variety of modifications to alter or enhance their properties. These modifications can be generally divided into two categories: endohedral and exohedral.10,11 Endohedral modification of C60 involves the incorporation of atoms/ions or small molecules into C60. Among those, lithium containing C60 (Li@C60) is an interesting candidate for NLO applications. Campbell et al. have studied its NLO properties by using mixed C60/Li@C60 films.12,13 They have been able to show an increased NLO response of Li@C60 compared to ordinary C60 and found a response comparable to other favorable organic molecules.12,13 One problem that remained was the low purity of the samples as Li@C60 was mixed with ordinary C60. However, in 2010 Aoyagi et al. have succeeded in obtaining highly purified Li@C60 reaching purities as high as 99%.14 Increased NLO properties have been theoretically predicted for Li+@C60(PF6−) salt.15 However, the NLO properties using these high purity samples have not been experimentally examined yet.
In this study, we demonstrate a simple method of obtaining Li@C60 thin films and characterize them by using Raman spectroscopy and atomic force microscopy (AFM). We further examine the NLO properties of the Li@C60 thin film excited by two distinct femtosecond (fs) pulsed laser beams on an optical microscope with epi-detection configuration. It is found that the Li@C60 thin films exhibit strong degenerate-six-wave mixing (DSWM), a fifth-order nonlinear optical effect with high reproducibility thanks to the high purity, marking it as a strong candidate for high-harmonic generation (HHG).
Experimental
Sample preparation
Li+@C60(PF6−) powder purified through recrystallization was purchased from Idea International Co. Ltd. Thin films of Li@C60 have been produced by simple dropcasting from a saturated o-dichlorobenzene (DCB) solution of Li+@C60(PF6−) in the presence of a trace amount of sodium chloride (NaCl) or sodium iodide (NaI) on a cleaned glass coverslip. C60–Ad films have been prepared by dropcasting from toluene solution of C60–Ad16 in toluene (0.5 mg mL−1). C60 films have been prepared following a reprecipitation method outlined by Masuhara et al.17 Shortly, C60 is dissolved in m-xylene (good solvent) and subsequently injected into ethanol (poor solvent).
Methods
Nonlinear optical (NLO) measurements and Raman spectroscopy were performed using an inverted optical microscope (Ti-U, Nikon) equipped with an OmegaScope 1000 for Atomic Force Microscopy (AFM) measurements. Observation of films by scanning electron microscope (SEM) was performed by Hitachi SU8230. Energy-dispersive X-ray spectroscopy (EDX) under SEM observation was performed by Bruker Quantax EDS. For NLO, the sample was illuminated by fs pulses at 820 nm (Mai Tai HP, Spectra-Physics, 120 fs, 80 MHz) and 1164 nm (Inspire HF 100, Spectra-Physics, 200 fs, 80 MHz, pumped by Mai Tai HP) focused by an objective lens (60x, Plan Apo, air, NA 0.95, Nikon). Temporal overlap of the two wavelengths has been achieved using a delay line that allows precise adjustment of the 820 nm beam path length. The laser pulses were directed to sample with a shortpass dichroic mirror (T750spxrxt, Chroma Technology Corporation). In order to block excitation light, a 750 short-pass (ET750sp, Chroma) was used. The fitting of NLO intensity depending on power has been done using “curve_fit” included in Python's scipy library. For Raman spectroscopy, a 633 nm laser (Research Electro-Optics, Inc.) was focused on samples with a 60x Plan Fluor objective (NA 0.85, Nikon). The laser was reflected by using a longpass dichroic mirror (ZT633rdc, Chroma Technology Corporation). To block excitation laser light, a 645 long-pass (HQ645LP, Chroma Technology Corporation) was used. The backscattered NLO and Raman signals were collected by the respective objectives used for excitation and spectra were recorded using a spectrograph (iHR320, HORIBA) equipped with a charge-coupled device (CCD) camera (Newton DU920P, Andor Technology Ltd). NLO/Raman signal from out of focus was removed by a pinhole (diameter 100 μm). AFM data was analyzed using Gwyddion.18
Results and discussion
Li@C60 thin film characterization
Li@C60 films have been made using a simple dropcasting method. 30 μL of a saturated solution of dichlorobenzene (DCB) containing Li+@C60(PF6−) (purity 91%) and NaCl or NaI were dropped on a cleaned glass coverslip. Previous studies of Li@C60 thin films have primarily used sublimation under ultra-high vacuum (UHV) to obtain thin films.19–22 In contrast to this conventional method, the approach presented here offers an easy and quick alternative that does not require UHV. After evaporation of the solvent, long-stretched films can be clearly observed on the surface in an optical transmission microscope image (Fig. 1a). To further investigate these films, Raman spectroscopy and AFM measurements have been carried out. The characteristic Raman spectrum of C60 can be clearly observed (Fig. 1b). An interesting feature is found when taking a closer look at the Ag(2) mode of C60 that is typically positioned at 1470 cm−1 for ordinary C60.23 In the case of Li@C60 films, the Ag(2) mode peak is shifted to 1466 cm−1. This is in good agreement with Li+@C60[PF6−] powder and means a shift compared to C60 powder by 3 cm−1 (see Fig. S5†). Experiments with potassium/calcium-doped C60 have shown that the origin of this shift can be traced to negative charge on the fullerene cage.24,25
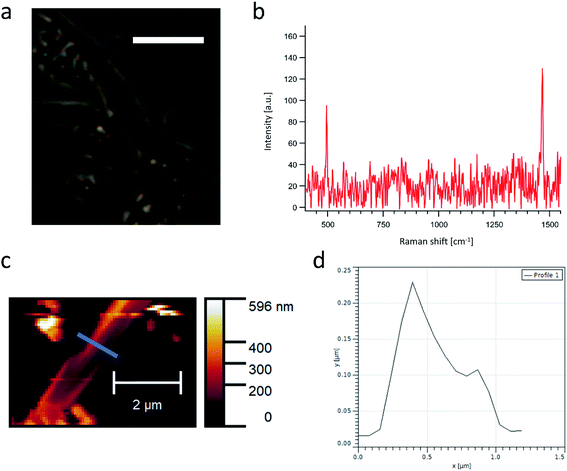 |
| Fig. 1 Li@C60 film characterization. (a) Optical transmission microscope image showing the films obtained after dropcasting the Li@C60 containing solution on the surface. Scale bar is 5 μm. (b) Raman spectrum obtained from Li@C60 film by irradiation with 633 nm laser. The spectrum displays the signature of C60. The spectrum was smoothed using Savitzky–Golay filter and background corrected. (c) AFM image showing the topography of a film formed after dropcasting. (d) Height profile of film shown in (c), position marked by blue line. | |
AFM measurements (Fig. 1c and d) reveal a small thickness of a range of 10–20 nm for these films. The thickness falls into the nanoscale region and is advantageous for epi-detecting of NLO signals. In case of four-wave mixing (FWM) and second-harmonic generation (SHG) the phase matching condition is typically only fulfilled in the forward direction but this is different for objects in the nanoscale region. For nanoscale objects, the phase matching condition is usually fulfilled in both forward and backward direction instead of just the forward direction making detection of NLO phenomena easier.26,27 This can be an advantage for later applications as designs can be more versatile in terms of excitation and detection directions. Taking advantage of the nanoscale structure, such thin films have been thus used in THz applications and ultra-fast information processing.1,28 EDX mapping under SEM observation was performed to chemically analyze the films formed using NaI as a salt. The long-stretched film was visualized by SEM, which is in good agreement with the AFM analysis (Fig. 2a, see S6† for additional SEM images). It can be seen from Fig. 2b–d that the films contain mainly carbon, phosphorus and fluorine, meaning the films are composed of Li@C60 and PF6 (see Fig. S7† for full EDX spectrum). On the other hand, sodium and iodine are not present in the films, meaning the salt is not directly involved in the film formation (Fig. 2e and f). If no salt was added to the solution, however, film formation was not observed at room temperature under ambient conditions. It is well known that salts can slow down the evaporation of a solvent since they increase the energy necessary for evaporation. If Li@C60 and PF6 films form slowly, a slow evaporation of the solvent would be necessary to see film formation. To check if such a factor was at play here, we cooled a solution without salt down for about 5 min in a freezer (−18 °C) and dropcasted the cold solution onto a coverslip. Cooling the solution is meant to simulate the effect of salt slowing down the evaporation of the solvent. As can be seen in Fig. S8,† film formation could be observed. We further tested the same procedure under cold conditions with a solution that did contain salt and film formation could be observed with even higher coverage. These results support the aforementioned effect of salt and suggest that Li@C60 and PF6 films form slowly and thus the solvent has to evaporate slowly in order to obtain films. Thus, salt supports film formation by slowing down the evaporation of the solvent.
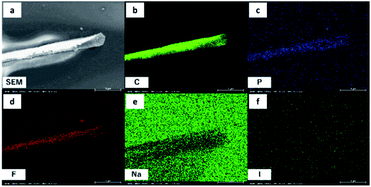 |
| Fig. 2 SEM and corresponding EDX maps showcasing the distribution of various elements of interest. (a) SEM image. (b)–(f) EDX maps showing the distribution of carbon (b), phosphorus (c), fluorine (d), sodium (e) and iodine (f). Scale bars are 9 μm. | |
Nonlinear optical properties of Li@C60 thin films
Fig. 3 shows a typical NLO spectrum observed while irradiating the sample with temporally overlapped two coherent fs pulses, i.e. pump at 820 nm and Stokes at 1164 nm. The two beams are linearly polarized, parallel to each other. Several NLO signals have been observed from a thin film of Li@C60: SHG (at 582 nm), sum-frequency generation (SFG, at 480 nm), degenerate-FWM (DFWM, at 630 nm) as well as a peak at roughly 455 nm that cannot be explained with just second or third order nonlinear optical effects. Degenerate six-wave mixing (DSWM), a higher order nonlinear effect, can be generated at the frequency 4ωs − ωp with the wave vector 4ks − kp, where ωi and ki (i = s or p) represent frequency and wave vector of Stokes or pump light, respectively. Converting 820 nm and 1164 nm wavelengths to their frequencies that are roughly 3.66 × 1014 Hz and 2.58 × 1014 Hz, respectively, we can see that |
4 × 258 THz − 1 × 366 THz = 666 THz ≙ 451 nm
| (1) |
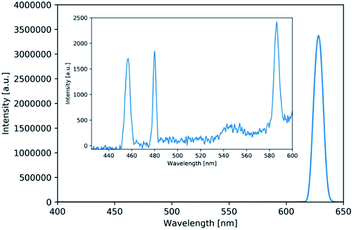 |
| Fig. 3 Spectrum obtained by irradiating a Li@C60 film with fs pulses at 820 nm and 1164 nm. Inset shows a zoomed in view of the spectrum from 425 to 600 nm. The peaks observed are DFWM (630 nm), SHG (582 nm), SFG (480 nm) and DSWM (455 nm). Peak power densities are 19.7 GW cm−2 (820 nm) and 22.5 GW cm−2 (1164 nm). | |
This calculation suggests that the observed peak around 455 nm is in fact DSWM, a fifth-order NLO effect. The small deviation from the actual peak position can be explained by fluctuations in the output wavelength of the Stokes beam.
In order to experimentally confirm that the observed effect can be attributed to a fifth-order process, the power dependence of this effect has been studied. The peak intensity was measured with varying powers of 820 nm and 1164 nm fs pulsed lasers for several films and the results fitted to a power law equation:
In this case, y is the intensity of the signal, x the input power, b depends on the number of photons involved in the process, while a is a constant. The results from two separated measurement sessions are displayed in Table S1.† The measurements show that the intensity of the 455 nm peak depends on the 1164 nm beam to the power of 4 and the 820 nm beam to the power of 1, supporting the above calculation (eqn (1)). The question remains if this is a direct six-wave mixing or a cascade effect meaning two subsequent third-order processes following each other. An example of such a cascade effect has been reported by Pelegati et al., observing cascade coherent anti-Stokes Raman scattering (CCARS).29 However, CCARS would show a power dependence of 3 for the pump beam and of 2 for the Stokes beam. Furthermore, the difference between the two exciting lasers corresponds to roughly 3604 cm−1, which is not in resonance with any Raman-active vibration of C60. There is still the possibility though of the process consisting of a combination of third-harmonic generation (THG) and FWM, both third-order processes. In order to compare the NLO performance of Li@C60 with other C60 derivatives, C60 films have been made following a method discussed by Masuhara et al.17 as well as thin films of C60–adamantylidene (C60–Ad) by using a similar dropcasting method as for Li@C60 (see Fig. S9† for optical microscope images, S10† for Raman spectra). C60–Ad was chosen for its good film formation property as well as having an exohedral modification that allows us to compare NLO performance from both an endohedral and exohedral fullerene. Multiple NLO spectra have been measured for Li@C60/C60–Ad/C60, respectively and each average spectrum was calculated from these. As all three types of films show different average thicknesses (20 nm for Li@C60 sample 1 and 10 nm for sample 2, 450 nm for C60, 300 nm for C60–Ad, see Fig. S11, S12, S13 and S14†), the average spectra for DFWM and DSWM have been adjusted by multiplying the spectrum of Li@C60 by 22.5/45 (for sample 1 and 2, respectively) and of C60–Ad by 1.5 (using the ratio of thicknesses of C60
:
Li@C60 and C60
:
C60–Ad, respectively).¶ A factor in NLO microscopy is the difference in emission in forward and backward direction. However, until a sample thickness of roughly half of the excitation wavelength, emission intensity is nearly similar in both directions and increases roughly linearly with the thickness in case of FWM.26,27 Fig. 4a shows the thickness adjusted DFWM signal. After accounting for the film thickness, DFWM signal from Li@C60 come out roughly 8 times as strong as ordinary C60, while C60–Ad shows only a small increase in intensity. Different values have been reported for χ(3) of C60 typically ranging from a magnitude of 10−9 to 10−12 esu.9 We predict the value of Li@C60 to be roughly one order of magnitude larger compared to ordinary C60, in line with the value reported by Campbell et al.12 The reason for this increase was attributed to a partial charge transfer, which has also been confirmed to happen here as determined from the Raman spectrum when looking at the Ag(2) mode peak shift at 1466 cm−1 (Fig. S5†). A striking feature is that SFG is of significant strength for Li@C60, even though it remains weaker than C60–Ad (Fig. 4b). SFG is a second order NLO effect which usually require breaking of centrosymmetry. However, observation of SHG in C60 films with significant bulk contribution has been reported30 despite it being a centrosymmetric molecule and typically having a centrosymmetric bulk structure.31 It is thus difficult to make quantitative comparisons as the signal is composed of contributions from the surface and the bulk. For Li@C60[PF6−] too, a centrosymmetric structure has been reported.32 Despite C60 showing significant bulk contribution at 450 nm thickness,30 the Li@C60 signal is still stronger despite the films being thinner by a factor of more than 22, pointing to its strong enhancement in Li@C60. We will provide reasons why enhancement of second-order effects is expected for Li@C60 and C60–Ad in the following section. The breaking of centrosymmetry is obvious for C60–Ad considering its exohedral modification. It is however less obvious for Li@C60. As discussed previously, the Raman spectrum of Li@C60 films has shown an Ag(2) mode peak shift by 3 cm−1 compared to ordinary C60 due to a charge transfer (Fig. S5†). It has been shown that negative charge reduces the degeneracy of HOMO and LUMO of C60 as well as its overall symmetry.33 Additionally, it has been shown that the encapsulated lithium ion is off center, thus causing further symmetry breaking.14 Symmetry breaking has also been reported for other endohedral fullerenes.34 The second and strikingly remarkable feature is that DSWM appears the strongest for Li@C60 and only weakly for C60 and C60–Ad (Fig. 4c) after adjusting for thickness. Since DSWM is a fifth-order effect it does not require breaking of centrosymmetry and can thus be efficiently generated in the bulk, meaning that thickness adjustment is similarly justified as for DFWM. It should be mentioned that for sample 2 of Li@C60, the beam spot size is slightly larger than the lateral size of the films meaning some excitation intensity is lost. Assuming a diffraction limited spot size, an objective with an NA of 0.95 means the spot size is roughly 1 μm while the films are typically around 700 nm. However, as the edges of a Gaussian beam have only low intensity and DSWM has a power dependence of 5 on the electric field, we assume that this loss can be neglected. The reason for the increase in intensity is likely due to a partial charge transfer, similar to the case of DFWM. Another possible reason could be its higher absorption in the UV region compared to ordinary C60.35 Chang et al. reported an enhanced emission of THG when the sample showed higher absorption even if no specific electronic transition was affected.36 C60 has been suggested as a promising material for HHG as harmonics can be generated with comparatively low input powers.8,9 The high strength of DSWM and its higher absorption all the way into the UV region35 indicates that Li@C60 can be an interesting choice for HHG.
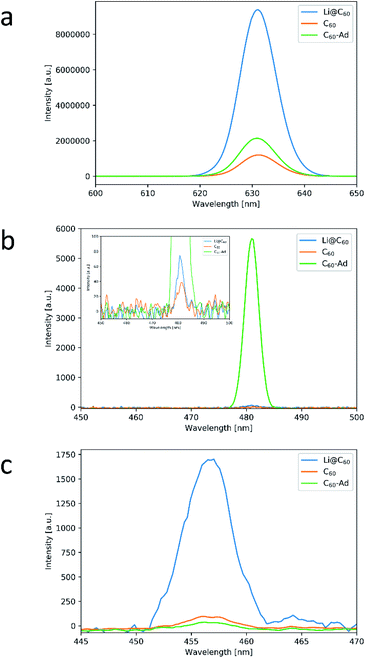 |
| Fig. 4 NLO spectra obtained by irradiation with 820 nm and 1164 nm fs pulses from Li@C60 films (blue), C60 (orange) and C60–Ad (green). (a) DFWM, thickness adjusted, sample 1. (b) SFG. Inset shows a zoomed in view of the spectrum. (c) DSWM, thickness adjusted, sample 2. Peak power densities were 21.0 GW cm−2 (820 nm) and 6.2 GW cm−2 (1164 nm) for (a) and (b) and 19.7 GW cm−2 (820 nm) and 22.5 GW cm−2 (1164 nm) for (c). | |
Conclusions
In conclusion, we have shown the formation and characterization of thin films made from Li@C60 and their NLO response. In particular, we were able to observe DSWM and significant second-order enhancement over ordinary C60. The observation of such high order NLO effects proves the strong NLO properties of Li@C60 and makes it is a promising candidate for HHG. This could further improve future X-ray lasers. Furthermore, SWM has been proposed as a way to obtain correlated photon triplets,37 which could allow further tests of quantum mechanics.
Author contributions
M. W.: conceptualization, methodology, formal analysis, investigation, visualization, writing – original drafts. S. T.: conceptualization, methodology, aupervision, writing – original draft. P. W.: writing – review and editing. K. U.: resources, writing – review and editing. A. M.: resources, writing – review and editing. H. F.: resources, writing – review and editing. Y. T.: resources, writing – review and editing. M. Y.: resources, writing – review and editing. K. H.: methodology, investigation, visualization, writing – original draft. E. F.: resources, writing – review and editing, funding acquisition. H. U.: conceptualization, supervision, writing – original draft, project administration, funding acquisition.
Conflicts of interest
There are no conflicts of interest to declare.
Acknowledgements
This work was supported by the research Foundation – Flanders (FWO, G0D4519N, G081916N) and the KU Leuven Research Fund (C14/15/053, C14/19/079). M. W. would like to thank Ngoc-Uyen Tran, M.Sc., for preparation of the TOC. M. W. acknowledges the FWO PhD-SB fellowship (1S87918N). JSPS Kakenhi (JP19KK0136, JP20K21200, JP17H03003, JP20K21165, JP21H04634), JSPS ‘Core-to-Core Program, A. Advanced Research Networks’, ‘Network Joint Research Center for Materials and Devices’ and the Photo-excitonix Project in Hokkaido University are greatly acknowledged. Funding was provided by the European Regional Development Fund and the programme Mobilitas Pluss (MOBJD609). We thank the open facility at Hokkaido University for accessing FE-SEM. H. F. thanks Kazuhiko Kawachi and Yasuhiko Kasama (IDEA international) for various information on synthesis and characterization of Li@C60. The authors want to thank Dr Yasuhiko Kasama (IDEA international) for providing a sample of Li@C60[PF6−]. The authors would like to thank Prof. Stijn Van Cleuvenbergen for his valuable advice.
Notes and references
- J. Y. Wu, Z. A. Li, J. D. Luo and A. K. Y. Jen, J. Mater. Chem. C, 2020, 8, 15009–15026 RSC.
- D. Cotter, R. J. Manning, K. J. Blow, A. D. Ellis, A. E. Kelly, D. Nesset, I. D. Phillips, A. J. Poustie and D. C. Rogers, Science, 1999, 286, 1523–1528 CrossRef CAS PubMed.
- Z. Chai, X. Y. Hu, F. F. Wang, X. X. Niu, J. Y. Xie and Q. H. Gong, Adv. Opt. Mater., 2017, 5, 1600665 CrossRef.
- L. Thevenaz, Front. Optoelectron. China, 2010, 3, 13–21 CrossRef.
- L. Caspani, C. L. Xiong, B. J. Eggleton, D. Bajoni, M. Liscidini, M. Galli, R. Morandotti and D. J. Moss, Light: Sci. Appl., 2017, 6, e17100 CrossRef CAS PubMed.
- H. S. Nalwa, Appl. Organomet. Chem., 1991, 5, 349–377 CrossRef CAS.
- M. Li, Y. Li, H. Zhang, S. Wang, Y. Ao and Z. Cui, J. Mater. Chem. C, 2017, 5, 4111–4122 RSC.
- G. P. Zhang, Phys. Rev. Lett., 2005, 95, 047401 CrossRef CAS PubMed.
- G. P. Zhang, X. Sun and T. F. George, J. Phys. Chem. A, 2009, 113, 1175–1188 CrossRef CAS PubMed.
- H. Shinohara, Rep. Prog. Phys., 2000, 63, 843–892 CrossRef CAS.
- R. Taylor, C. R. Chim., 2006, 9, 982–1000 CrossRef CAS.
- E. E. B. Campbell, S. Couris, M. Fanti, E. Koudoumas, N. Krawez and F. Zerbetto, Adv. Mater., 1999, 11, 405–408 CrossRef CAS.
- E. E. B. Campbell, M. Fanti, I. V. Hertel, R. Mitzner and F. Zerbetto, Chem. Phys. Lett., 1998, 288, 131–137 CrossRef CAS.
- S. Aoyagi, E. Nishibori, H. Sawa, K. Sugimoto, M. Takata, Y. Miyata, R. Kitaura, H. Shinohara, H. Okada, T. Sakai, Y. Ono, K. Kawachi, K. Yokoo, S. Ono, K. Omote, Y. Kasama, S. Ishikawa, T. Komuro and H. Tobita, Nat. Chem., 2010, 2, 678–683 CrossRef CAS PubMed.
- A. K. Srivastava, A. Kumar and N. Misra, Phys. E, 2016, 84, 524–529 CrossRef CAS.
- T. Akasaka, M. T. H. Liu, Y. Niino, Y. Maeda, T. Wakahara, M. Okamura, K. Kobayashi and S. Nagase, J. Am. Chem. Soc., 2000, 122, 7134–7145 CrossRef CAS.
- A. Masuhara, Z. Tan, H. Kasai, H. Nakanishi and H. Oikawa, Jpn. J. Appl. Phys., 2009, 48, 050206 CrossRef.
- D. Nečas and P. Klapetek, Cent. Eur. J. Phys., 2012, 10, 181–188 Search PubMed.
- H. J. Chandler, M. Stefanou, E. E. B. Campbell and R. Schaub, Nat. Commun., 2019, 10, 2283 CrossRef PubMed.
- M. Stefanou, H. J. Chandler, B. Mignolet, E. Williams, S. A. Nanoh, J. O. F. Thompson, F. Remacle, R. Schaub and E. E. B. Campbell, Nanoscale, 2019, 11, 2668–2678 RSC.
- Y. Yamada, A. V. Kuklin, S. Sato, F. Esaka, N. Sumi, C. Zhang, M. Sasaki, E. Kwon, Y. Kasama, P. V. Avramov and S. Sakai, Carbon, 2018, 133, 23–30 CrossRef CAS.
- H. Yagi, N. Ogasawara, M. Zenki, T. Miyazaki and S. Hino, Chem. Phys. Lett., 2016, 651, 124–126 CrossRef CAS.
- J. Menendez and J. B. Page, in Light Scattering in Solids VIII, ed. M. Cardona and G. Güntherodt, Springer, Berlin, 1st edn, 2000, ch. 2, vol. 76, pp. 27–95 Search PubMed.
- X. H. Chen, X. J. Zhou and S. Roth, Phys. Rev. B, 1996, 54, 3971–3975 CrossRef CAS PubMed.
- J. Winter and H. Kuzmany, Solid State Commun., 1992, 84, 935–938 CrossRef CAS.
- J. X. Cheng, A. Volkmer and X. S. Xie, J. Opt. Soc. Am. B, 2002, 19, 1363–1375 CrossRef CAS.
- R. LaComb, O. Nadiarnykh, S. S. Townsend and P. J. Campagnola, Opt. Commun., 2008, 281, 1823–1832 CrossRef CAS PubMed.
- M. Jazbinsek, U. Puc, A. Abina and A. Zidansek, Appl. Sci., 2019, 9, 882 CrossRef CAS.
- V. B. Pelegati, B. B. C. Kyotoku, L. A. Padilha and C. L. Cesar, Biomed. Opt. Express, 2018, 9, 2407–2417 CrossRef CAS PubMed.
- X. K. Wang, T. G. Zhang, W. P. Lin, S. Z. Liu, G. K. Wong, M. M. Kappes, R. P. H. Chang and J. B. Ketterson, Appl. Phys. Lett., 1992, 60, 810–812 CrossRef CAS.
- K. Prassides, H. W. Kroto, R. Taylor, D. R. M. Walton, W. I. F. David, J. Tomkinson, R. C. Haddon, M. J. Rosseinsky and D. W. Murphy, Carbon, 1992, 30, 1277–1286 CrossRef CAS.
- S. Aoyagi, Y. Sado, E. Nishibori, H. Sawa, H. Okada, H. Tobita, Y. Kasama, R. Kitaura and H. Shinohara, Angew. Chem., Int. Ed., 2012, 51, 3377–3381 CrossRef CAS PubMed.
- W. H. Green, S. M. Gorun, G. Fitzgerald, P. W. Fowler, A. Ceulemans and B. C. Titeca, J. Phys. Chem., 1996, 100, 14892–14898 CrossRef CAS.
- P. M. Felker, V. Vlček, I. Hietanen, S. Fitzgerald, D. Neuhauser and Z. Bačić, Phys. Chem. Chem. Phys., 2017, 19, 31274 RSC.
- F. Cataldo, D. A. García-Hernández and A. Manchado, Fullerenes, Nanotubes, Carbon Nanostruct., 2019, 27, 695–701 CrossRef CAS.
- C. F. Chang, C. H. Yu and C. K. Sun, J. Biophotonics, 2010, 3, 678–685 CrossRef CAS PubMed.
- S. Q. Zhang, W. Li, K. K. Li, Y. M. Li, F. Mu, Y. Feng, Y. Liu and Y. P. Zhang, Ann. Phys., 2020, 412, 168000 CAS.
Footnotes |
† Electronic supplementary information (ESI) available. See DOI: 10.1039/d1ra08051b |
‡ Present address: Department of Applied Chemistry, National Yang Ming Chiao Tung University, 1001 Ta Hsueh Rd., Hsinchu 30010, Taiwan. |
§ Present address: Department of Materials and Environmental Technology, Tallinn University of Technology, Ehitajate tee 5, 19086 Tallinn, Estonia. |
¶ Polarization dependence is not considered here as it was reported previously that C60 films do not show any polarization dependence when rotating linear polarized light in the x,y-plane.30 As Li@C60[PF6−] has a similar structure to C60, we assume a similar situation here.32 The spectra obtained from Li@C60 films, as well as C60–Ad and C60 films can be seen in Fig. S15–17† together with further discussion concerning the polarization dependence. |
|
This journal is © The Royal Society of Chemistry 2022 |
Click here to see how this site uses Cookies. View our privacy policy here.