DOI:
10.1039/D1RA07654J
(Paper)
RSC Adv., 2022,
12, 1319-1330
Supported L-tryptophan on Fe3O4@SiO2 as an efficient and magnetically separable catalyst for one-pot construction of spiro[indene-2,2′-naphthalene]-4′-carbonitrile derivatives†
Received
15th October 2021
, Accepted 17th December 2021
First published on 5th January 2022
Abstract
In this work, L-tryptophan functionalized silica-coated magnetic nanoparticles were readily prepared and evaluated as a recyclable magnetic nanocatalyst for the synthesis of spiro[indene-2,2′-naphthalene]-4′-carbonitrile derivatives through the one-pot four-component reaction of malononitrile, cyclohexanone, aromatic aldehydes, and 1,3-indandione. This novel magnetic nanocatalyst was confirmed to be effective and provide products in moderate to excellent yields under reflux conditions. The structure of obtained nanoparticles was characterized using FT-IR, XRD, VSM, EDX, elemental mapping, FE-SEM, and TGA. This synthetic protocol provides several benefits such as excellent yields in short reaction times (64–91%), saving costs, reusability of the catalyst using an external magnet (seven runs), and low catalyst loading.
1. Introduction
Multicomponent reactions (MCRs) and associated one-pot synthesis such as domino, tandem and cascade reactions have always been one of the most significant scopes in the development of new methodologies in organic synthesis and catalysis due to their great applications, particularly in pharmaceutical studies.1–4 MCRs often produce highly complex molecules from simple precursors, thus, avoiding complicated purification steps and saving solvents, reagents and times.5–7
Spiro compounds show a broad range of effective performance in pharmacology. They have a large number of pharmacological and biological properties such as anti-cancer,8 antimicrobial,9 anti-diabetic,10 antitumor,11 and anti-hypertensive12 activities due to their fascinating structures (Scheme 1). Among the spiro family members, spirocarbocycles are imperative scaffolds known for their extensive pharmacological significance that are found in numerous products and bioactive molecules. It is worth pointing out that, MCRs are one of the most potent techniques for the production of spiro compounds.13,14 Furthermore, vinylogous Michael addition was reported as a key step in the preparation of many spiro compounds.15–17
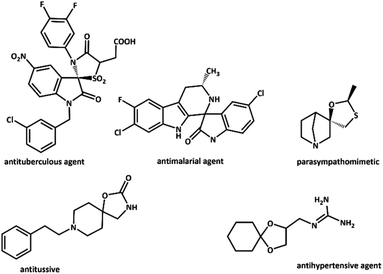 |
| Scheme 1 Relevant bioactive compounds containing spiro frameworks. | |
In recent years, a few synthesis methods for the synthesis of spiro[indene-2,2′-naphthalene]-4′-carbonitriles have been reported in the literature. L-Proline,18 ethylene glycol19 and quaternary ammonium surfactant [C18-Dabco][Br]20 have been utilized in these methods. Nevertheless, there are drawbacks with these reported strategies, for instance, prolonged reaction time, catalyst toxicity, and more importantly the catalyst reusability is difficult. However, further studies are still essential for the efficient, environmental and economical multicomponent methodology for the synthesis of these spiro compounds, even though each of the known methods for the synthesis of spiro[indene-2,2′-naphthalene]-4′-carbonitrile compounds has its competency.
In general, magnetic nanoparticles have been applied for a range of biomedical applications, particularly in the areas of medical imaging, diagnostics, and treatment.21–25 Additionally, they have been commonly employed in many organic reactions because of their easy removal and convenient separation using an external magnetic field.26–29 Among all magnetic nanoparticle types, Fe3O4 nanoparticles (NPs) have received more attention owing to their exclusive features such as superparamagnetic nature, non-toxic, biocompatibility, and facile synthesis process from accessible precursors (e.g., ferrous and ferric salts).30 Also, Fe3O4 nanoparticles possess plenty of hydroxyl groups (OH) on their surfaces, thus, they are normally hydrophilic. However, the naked Fe3O4 is not stable since it can be easily oxidized into the other substances in the air, therefore, coating materials or modification is critical in order to prevent them from oxidation. For this purpose, silica (SiO2) has been widely considered as one of the best protection shells since it is inexpensive, has a high specific surface area, and has high resistance under catalytic conditions. It is noteworthy that the functional groups containing oxygen, which are distributed on the surface of Fe3O4@SiO2 can act as active sites.
L-Tryptophan is known as a chiral molecule. Environmental-friendliness, less-expensive, and easy availability are some of the benefits of L-tryptophan. Moreover, this α-amino acid can participate in many organic reactions as a catalyst because it possesses active site groups (amino group and carboxylic group). In other words, L-tryptophan can be broadly used in base-catalyzed organic reactions.31 However, tough recovery and prolonged reaction times are prominent drawbacks of this catalyst. Several strategies can be used to overcome the above-mentioned problems such as co-catalysts, varying types of supports such as ionic liquids,32 polymers,33 and silica.34
In this study, we introduce a straightforward approach for the fabrication of Fe3O4@SiO2-L-tryptophan as a robust inorganic-organic hybrid catalyst. This heterogeneous, environmentally benign, and highly reusable catalyst represents high catalytic activity to synthesize spiro[indene-2,2′-naphthalene]-4′-carbonitrile derivatives via a one-pot, four-component condensation reaction of malononitrile (1 mmol), cyclohexanone (1 mmol), various aromatic aldehydes (1 mmol) and 1,3-indandione (1 mmol) under reflux conditions in EtOH.
2. Result and discussion
2.1 Preparation and characterization of Fe3O4@SiO2-L-tryptophan catalyst
Magnetic nanoparticles (MNPs) were prepared using Fe2+ and Fe3+ ions by the co-precipitation method. Then, Fe3O4 NPs coated by the silica network provided reaction sites for further functionalization and thermal stability. In the end, Fe3O4@SiO2 was reacted with L-tryptophan in the presence of H2SO4 in order to produce Fe3O4@SiO2-L-tryptophan nanoparticles (Scheme 2). Of note, the L-tryptophan layer is linked on the surface of Fe3O4@SiO2 by chemical adsorption.35
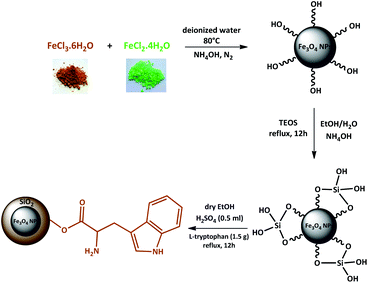 |
| Scheme 2 Schematic for the preparation of Fe3O4@SiO2-L-tryptophan nanocatalyst. | |
Fig. 1 illustrates FT-IR spectra of Fe3O4, Fe3O4@SiO2, Fe3O4@SiO2-L-tryptophan, and L-tryptophan. It can be observed that the band in the region of 578 cm−1 is attributed to vibrations of the Fe–O bond and bands at 3400 and 1626 cm−1 are ascribed to O–H stretching and bending vibrations, respectively (Fig. 1a). In the Fe3O4@SiO2 spectrum, the strong peak at 1089 cm−1 could be assigned to asymmetric stretching vibrations of Si–O–Si bonds. This peak verifies that the silica layer was coated on the surface of Fe3O4 very well (Fig. 1b). For the L-tryptophan functional group, the absorption band at 1162 cm−1 belongs to the C–N stretching vibration and the peak at 3409 cm−1 corresponds to O–H stretching vibrations (Fig. 1c).
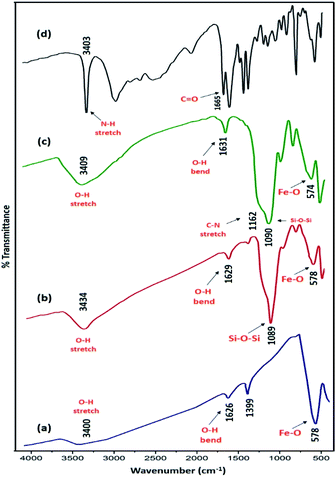 |
| Fig. 1 FT-IR spectra of (a) Fe3O4, (b) Fe3O4@SiO2, (c) Fe3O4@SiO2@L-tryptophan and (d) L-tryptophan. | |
The powder X-ray diffraction (XRD) patterns of Fe3O4 and Fe3O4@SiO2-L-tryptophan are displayed in Fig. 2. XRD data clearly demonstrated 6 diffraction angles (2θ) at 30.2°, 35.5°, 43.2°, 53.6°, 57.1°, and 62.8°, following standard Fe3O4 XRD patterns (JCPDS No. 75-0449) (Fig. 2a). From Fig. 2b, it can be clearly observed at about 2θ = 24°, which is assigned to the amorphous organic group. The diameter (D) of the Fe3O4@SiO2-L-tryptophan NPs was calculated using the Scherrer equation (D = Kλ/(β
cos
θ)), where θ is the Bragg angle of the maximum of the diffraction peak and β is the line broadening at half the maximum, while λ is the X-ray wavelength (0.154 nm for CuKα). K is a dimensionless shape factor, which usually takes a value of about 0.9.
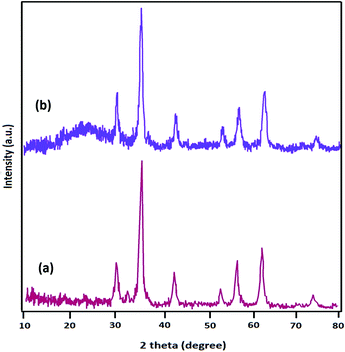 |
| Fig. 2 XRD patterns of (a) Fe3O4, and (b) Fe3O4@SiO2-L-tryptophan. | |
Magnetic properties of Fe3O4, Fe3O4@SiO2, and Fe3O4@SiO2-L-tryptophan nanoparticles were measured with the help of a vibrating sample magnetometer (VSM) (Fig. 3). According to these results, all three samples are superparamagnetic at 60.7 emu g−1, which belongs to Fe3O4 NPs showing the highest value of saturation magnetization (Ms). Additionally, the magnitude of saturation magnetization values for Fe3O4@SiO2 and Fe3O4@SiO2-L-tryptophan are 48.23 emu g−1 and 29.64 emu g−1, respectively. These results illustrate that the magnetization property decreases after coating and functionalization.
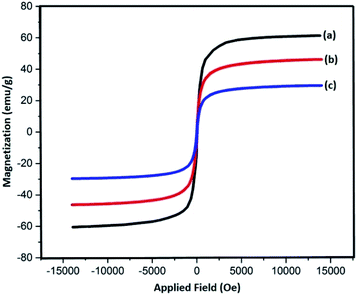 |
| Fig. 3 VSM curves of (a) Fe3O4, (b) Fe3O4@SiO2 and, (c) Fe3O4@SiO2-L-tryptophan nanoparticles. | |
The presenting elements can be clearly seen in the structure of Fe3O4@SiO2-L-tryptophan using energy-dispersive X-ray spectroscopy (EDX) (Fig. 4). Besides, all elements are well distributed throughout the Fe3O4@SiO2-L-tryptophan, which is revealed by elemental mapping images (Fig. 5).
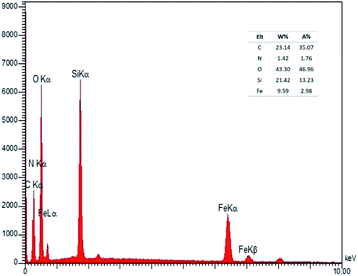 |
| Fig. 4 EDX analysis of Fe3O4@SiO2-L-tryptophan nanoparticles. | |
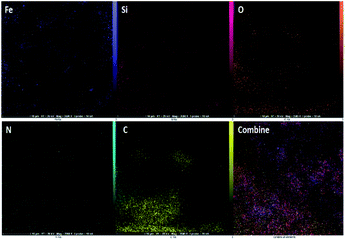 |
| Fig. 5 Elemental mapping images of Fe3O4@SiO2-L-tryptophan nanoparticles. | |
In order to study the morphology, uniformity and size of the catalyst, FE-SEM was used. As it is obvious from Fig. 6, SEM images of the sample indicate that the prepared nanoparticles have a uniform size, spherical shape, and disordered mesosphere. The average size of Fe3O4@SiO2-L-tryptophan MNPs was calculated to be about 37 nm.
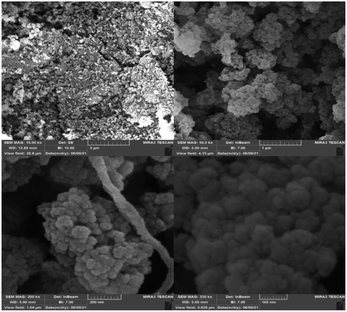 |
| Fig. 6 FE-SEM photographs of Fe3O4@SiO2-L-tryptophan nanoparticles. | |
The thermal behavior of Fe3O4@SiO2-L-tryptophan nanoparticles was estimated by TGA analysis (Fig. 7). Results depict appropriate thermal stability with no significant decline in the weight. The weight loss at low temperatures (<∼100 °C) can be either due to the removal of surface –OH groups or physically adsorbed solvent molecules trapped in the SiO2 layer. According to the curve, the observed weight loss of about 11.1 (%) above 250 °C can be because of the decomposition of L-tryptophan group, which is attached to the silica layer. Therefore, the TGA curve reveals that Fe3O4@SiO2-L-tryptophan NPs is stable up to 250 °C and is certainly fit for the synthesis of spiro[indene-2,2′-naphthalene]-4′-carbonitrile compounds.
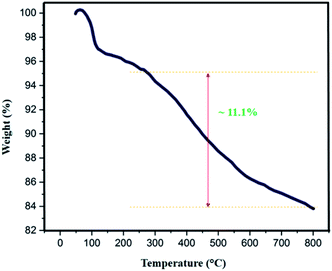 |
| Fig. 7 TGA curve of Fe3O4@SiO2-L-tryptophan NPs. | |
In order to calculate the extent of the functionalization per SiO2 group, weight loss values were employed together with the molecular weight of diverse moieties, and eqn (1) was used for the calculation.36 In this equation, X stands for the number of sample SiO2 groups per each covalent functional group (L-tryptophan), R (%) is the residual mass at 800 °C in the TGA plot, L (%) is the weight loss in the range of 100–800 °C, and Mw is the molecular weight of the desorbed functional groups. Taking into account that the covalently L-tryptophan measurements depicted one functionality every ∼25 SiO2 groups in the Fe3O4@SiO2-L-tryptophan.
|
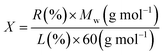 | (1) |
2.2 Comparison of the efficiency of solvents, amount of Fe3O4@SiO2-L-tryptophan NPs, and other catalysts on the synthesis of spiro[indene-2,2′-naphthalene]-4′-carbonitriles
The tandem one-pot reaction of malononitrile, cyclohexanone, 4-nitro benzaldehyde, and 1,3-indandione was chiefly selected as a model reaction in order to observe the catalytic activity of the prepared Fe3O4@SiO2-L-tryptophan. To optimize the reaction, we utilized different conditions. At first, the effect of the various electron-pair donor (EPD) and electron-pair acceptor (EPA) solvents were studied to assess the best reaction conditions. According to the results displayed in Table 1, EPD and protic solvents (acting as Lewis bases) showed better performance in the reaction. Based on the reaction mechanism, protic solvents surrounded carboanion and carbocations, hence, the activation energy was decreased.37 Satisfactory result were observed in terms of the yield and reaction time in the EtOH solvent. Besides, when the reaction was carried out using Fe3O4@SiO2-L-tryptophan NPs as the catalyst in EtOH, the product was obtained in excellent yields in a very short time (Table 1, entry 7).
Table 1 Optimization of reaction conditions for the formation of spiro[indene-2,2′-naphthalene]-4′-carbonitrilesa
Entry |
Solvent |
Catalyst |
Time (min) |
Yieldb (%) |
Reaction conditions: malononitrile (1 mmol), cyclohexanone (1 mmol) p-nitro benzaldehyde (1 mmol), and 1,3-indandione (1 mmol) in the presence of Fe3O4@SiO2-L-tryptophan under reflux conditions. Isolated yields. |
1 |
H2O |
Nano Fe3O4@SiO2@L-tryp (20 mg) |
180 |
45 |
2 |
EtOH/H2O |
Nano Fe3O4@SiO2@L-tryp (20 mg) |
150 |
64 |
3 |
DMF |
Nano Fe3O4@SiO2@L-tryp (20 mg) |
180 |
41 |
4 |
THF |
Nano Fe3O4@SiO2@L-tryp (20 mg) |
180 |
— |
5 |
CH3CN |
Nano Fe3O4@SiO2@L-tryp (20 mg) |
180 |
39 |
6 |
MeOH |
Nano Fe3O4@SiO2@L-tryp (20 mg) |
50 |
82 |
7 |
EtOH |
Nano Fe3O4@SiO2@L-tryp (20 mg) |
30 |
91 |
8 |
EtOH |
Morpholine |
50 |
78 |
9 |
EtOH |
TEA |
180 |
30 |
10 |
EtOH |
PTSA |
180 |
— |
As a remarkable point, when we utilized a strong base catalyst, the obtained product had a low yield (Table 1, entry 9). In addition, in the presence of the acid catalyst, no product was observed in the period of 180 min (Table 1, entry 10). According to the data, conducting the model reaction without any catalyst gave only 21% after 180 min (Table 2, entry 1). We also investigated the catalytic activity of Fe3O4@SiO2, L-tryptophan and Fe3O4@SiO2-L-tryptophan. Based on our empirical experiments, L-tryptophan, which was modified on the surface of Fe3O4@SiO2 indicated the best catalytic performance in the reaction. Moreover, it was discovered that in the presence of Fe3O4@SiO2-L-tryptophan at 20 mg, the reaction rate and yield were considerably increased (Table 2, entry 5).
Table 2 Reported catalytic systems for the formation of spiro[indene-2,2′-naphthalene]-4′-carbonitrilesa
Entry |
Catalyst |
Time (min) |
Temp. (°C) |
Yieldb (%) |
Ref. |
Reaction conditions: malononitrile (1 mmol), cyclohexanone (1 mmol) p-nitro benzaldehyde (1 mmol), and 1,3-indandione (1 mmol) in the presence of Fe3O4@SiO2-L-tryptophan in EtOH under reflux conditions. Isolated yields. |
1 |
— |
180 |
Reflux |
21 |
This work |
2 |
Fe3O4@SiO2 (20 mg) |
300 |
Reflux |
42 |
This work |
3 |
L-Tryptophan (20 mg) |
300 |
Reflux |
68 |
This work |
4 |
Nano Fe3O4@SiO2@L-tryp (20 mg) |
80 |
rt |
90 |
This work |
5 |
Nano Fe3O4@SiO2@L-tryp (20 mg) |
30 |
Reflux |
91 |
This work |
6 |
Nano Fe3O4@SiO2@L-tryp (15 mg) |
80 |
Reflux |
90 |
This work |
7 |
Nano Fe3O4@SiO2@L-tryp (30 mg) |
30 |
Reflux |
91 |
This work |
8 |
L-Proline (15 mol%) |
180 |
rt |
89 |
18 |
9 |
Ethylene glycol (3.3 g) |
150 |
100 |
84 |
19 |
10 |
DABCO (10 mol%) |
90 |
rt |
88 |
20 |
To investigate the scope of this protocol, various aromatic aldehydes (4), possessing both electron-donating and withdrawing groups were reacted with 1,3-indandione, malononitrile, and cyclohexanone (Table 3). The results were ascertained that aromatic aldehydes with electron-withdrawing groups reacted faster compared to those with electron-donating groups. Furthermore, the electron-withdrawing groups at the para position of benzaldehyde (5a, 5d, and 5j) resulted in excellent yields (Table 3).
Table 3 Fe3O4@SiO2@L-tryptophan NPs catalyzed synthesis of spiro[indene-2,2′-naphthalene]-4′-carbonitrile derivativesa
2.3 Reusability of the Fe3O4@SiO2-L-tryptophan MNPs
The reusability of the catalyst is well known as key characteristic properties. Herein, we have investigated the retrievability of Fe3O4@SiO2-L-tryptophan MNPs using the model reaction of malononitrile, cyclohexanone, 4-nitro benzaldehyde, and 1,3-indandione. As it can be viewed from Fig. 8, the catalytic activity of Fe3O4@SiO2-L-tryptophan declined from 98% in the fresh run to 80% after the completion of seven runs.
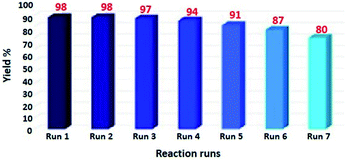 |
| Fig. 8 Recycling of Fe3O4@SiO2-L-tryptophan MNPs as the catalyst. | |
The prepared nanocatalyst was conveniently separated at the end of the reaction using a strong magnet, washed with EtOH and water, then dried at 60 °C, and reused seven times without excess purification.
The heterogeneous process was also checked for the model reaction. For this purpose, the catalyst was separated from the reaction mixture after 10 minutes and the reaction yield was estimated to be around 64%. Then, the reaction continued without the catalyst for another 20 minutes. The obtained product had no significant increase in the yield (∼67%). According to the above-mentioned results, the presence of the catalyst until at the end of the reaction is necessary to reach the best yield.
The amount of the base in the catalyst before and after the cyclic test was quantitatively evaluated through ion-exchange pH analysis.39 Based on the acid–base titration measurement, the number of basic sites of the catalyst was approximately 1.250 mmol g−1 after being recycled for seven cycles, while the amount of basic sites in the fresh Fe3O4@SiO2-L-tryptophan nanocatalyst were estimated at 1.406 mmol g−1. Further, the chemical structure of the recovered catalyst after 7 cycles was confirmed using the FT-IR spectrum. As can be observed in Fig. 9, there is no considerable difference between FT-IR spectra of the fresh and reused magnetic nanocatalyst.
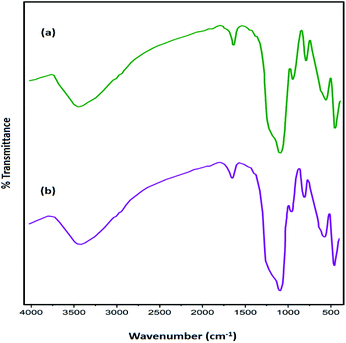 |
| Fig. 9 FT-IR spectra of Fe3O4@SiO2-L-tryptophan MNPs (a) before and (b) after seven reaction cycles. | |
2.4 A plausible mechanism for the preparation of spiro[indene-2,2′-naphthalene]-4′-carbonitriles
A feasible mechanism and catalytic cycle are illustrated in Scheme 3 for the preparation of spiro[indene-2,2′-naphthalene]-4′-carbonitriles. Initially, the reaction begins through acidic sites (electrostatic attraction) of Fe3O4@SiO2 nanocatalyst binds with the oxygen atom of the carbonyl group. Simultaneously, the acidic hydrogen of malononitrile is removed by lone pairs of the amino group of L-tryptophan. Afterward, the carbonyl group (C
O) of cyclohexanone is attacked by carbanion. The Knoevenagel condensation occurs to produce the intermediate (I). The same conditions have taken place between two other compounds. In other words, 1,3-indanedione and aromatic aldehydes underwent the Knoevenagel condensation and afforded intermediate (II). In continuing, basic sites of the nanocatalyst (amino group) removed the γ-proton of cyclohexylidene malononitrile (I) to afford the cyclohexylidene malononitrile carbanion. In the next step, the carbanion attacks the activated double bond of the intermediate (II) via the Michael addition to produce the intermediate (III). Then, intermediate (IV) is furnished by an intramolecular nucleophilic addition reaction. Ultimately, an isomerization results to form the final product.
3. Experimental
3.1 General
All solvents and reagents were purchased commercially and were utilized without any further purification. Fourier transform infrared (FT-IR) spectroscopy was performed using a Nicolet Magna-400 spectrometer (KBr pellets). 1H NMR was recorded in DMSO-d6 solvent using a Bruker DRX-400 spectrometer with tetramethylsilane (TMS) as the internal reference. XRD patterns were recorded using a Philips diffractometer and monochromatized Cu Kα radiation (k = 1.5406 Å). The morphological study of the nanoparticles was conducted using field emission scanning electron microscopy (FE-SEM) (model MIRA3). The electron dispersive X-ray (EDX) analysis of the catalyst was performed using an Oxford instrument company. Thermogravimetric analysis (TGA) was performed on a Mettler TA4000 system TG-50 at a heating rate of 10 K min−1 under N2 atmosphere. The magnetic properties of samples were measured using a magnetometer (VSM, PPMS-9T) at 300 K in Iran (Kashan University). Melting points were obtained using a Yanagimoto micromelting point apparatus and are uncorrected. The purity determination of the substrates and reaction monitoring were accomplished using TLC on silica-gel polygram SILG/UV 254 plates (from Merck Company).
3.2 General procedure for the synthesis of Fe3O4 nanoparticles
Fe3O4 nanoparticles were prepared using the chemical co-precipitation method according to our previous work with some modifications.38,39 Briefly, FeCl3·6H2O (16 mmol, 4.32 g) and FeCl2·4H2O (8 mmol, 1.6 g) were dissolved in 100 mL of deionized water under N2 protection. Then, the reaction temperature was increased to 80 °C and 25 mL NH4OH (25%) was added to the solution dropwise (pH = 12). After adding NH4OH to the solution, the color of the solution turned black. The reaction was stirred at 80 °C for 1 h under reflux conditions. The magnetic nanoparticles were separated from the solution using an external magnet and washed several times with deionized water and ethanol and then dried at 70 °C for 12 h in an oven.
3.3 Preparation of Fe3O4@SiO2
Fe3O4@SiO2 nanoparticles were obtained according to the reported method in the literature with some modifications.40,41 Magnetic nanoparticles (1 g) were dispersed in a solution of ethanol and water (40
:
10 mL) in an ultrasonic bath for 30 min. The pH was adjusted to 10 with an ammonia solution and 0.5 mL tetraethylorthosilicate (TEOS), which was added dropwise into the mixture over a period of 1 h. The resulting solution was stirred at 35–40 °C for 12 h. Fe3O4@SiO2 MNPs were separated from the solution by using an external magnet and washed with ethanol (3 × 15 mL) and dried at room temperature.
3.4 Preparation of Fe3O4@SiO2@L-tryptophan
Firstly, Fe3O4@SiO2 MNPs (1 g) were dispersed in dry ethanol (10 mL) using an ultrasonic bath for 30 min. Subsequently, H2SO4 (0.5 mL) and L-tryptophan (1.5 g) were added to the solution and heated under reflux conditions at 90 °C for 12 h. The resulting MNPs were collected by magnetic separation followed by washing with ethanol and water several times before being dried in an oven at 60 °C to give Fe3O4@SiO2@L-tryptophan as a light brown powder.
3.5 A common procedure for the synthesis of (5a–l)
Typically, malononitrile (1) (1 mmol) and cyclohexanone (2) (1 mmol) were stirred in EtOH in the presence of Fe3O4@SiO2@L-tryptophan at room temperature (intermediate A). In the other test tube, 1,3-indanedione (3) (1 mmol) and various aromatic aldehydes (4) (1 mmol) were mixed and stirred under equal conditions (intermediate B). Then, intermediate A was added to intermediate B and the reaction was continued for the right time to achieve the desired product (Scheme 4). Upon completion of the time, the reaction was monitored by TLC (n-hexane/ethyl acetate: 6
:
4) the crude precipitate was filtered off and purified by recrystallization from ethanol to obtain the pure product. All products were characterized by melting point (m.p.), FT-IR, 1H NMR, 13C NMR and elemental microanalysis.
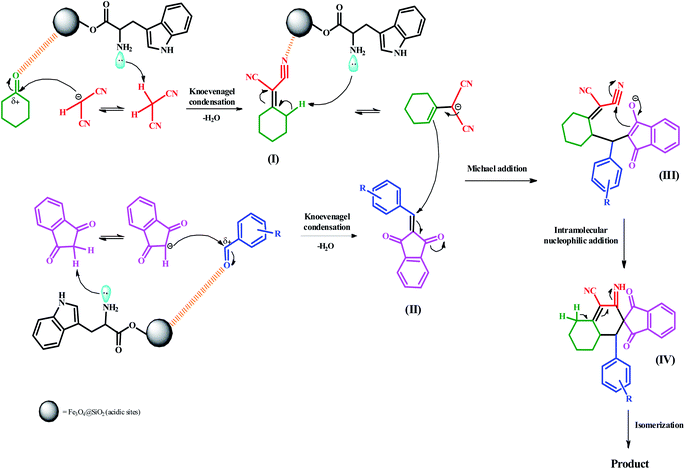 |
| Scheme 3 The probable mechanism for the preparation of spiro[indene-2,2′-naphthalene]-4′-carbonitrile compounds. | |
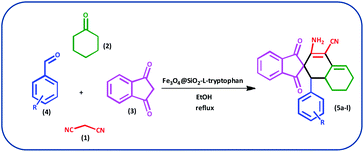 |
| Scheme 4 Preparation of spiro[indene-2,2′-naphthalene]-4′-carbonitrile derivatives by using Fe3O4@SiO2-L-tryptophan as the catalyst. | |
3.5.1 3′-Amino-1′-(4-nitrophenyl)-1,3-dioxo-1,3,6′,7′,8′,8a′-hexahydro-1′H-spiro[indene-2,2′-naphthalene]-4′-carbonitrile (5a). Yellow solid: m.p.: 210–240 (Decompose). IR (cm−1): 3431, 3353, 3254, 2941, 2849, 2201, 1700, 1645, 1590, 1349, 1238. 1H NMR (400 MHz, DMSO-d6) δ (ppm): 7.87–7.83 (m, 2H), 7.76–7.74 (m, 3H), 7.67 (d, 1H, J = 7.6 Hz), 7.16 (d, 1H, J = 9.2 Hz), 7.07 (d, 1H, J = 8 Hz), 6.27 (s, 2H, NH2, D2O exchangeable), 5.64 (s, 1H, vinylic), 3.26 (d, 1H, J = 12.8 Hz, –CH), 3.14 (m, 1H, CH), 2.22–2.17 (m, 1H, CH2), 2.07–2.04 (m, 1H, CH2), 1.64 (m, 1H, CH2), 1.43 (m, 1H, CH2), 1.28–1.25 (m, 1H, CH2), 0.82 (q, 1H, J = 12.4 Hz, CH2). Anal. calcd for C25H19N3O4: C, 70.58; H, 4.50; N, 9.88; O, 15.04; found: C, 70.55; H, 4.47; N, 9.89; O, 15.03.
3.5.2 3′-Amino-1′-(2,4-dichlorophenyl)-1,3-dioxo-1,3,6′,7′,8′,8a′-hexahydro-1′H-spiro[indene-2,2′-naphthalene]-4′-carbonitrile (5b). Yellow solid: m.p.: 245–250 (Decompose). IR (cm−1): 3459, 3366, 3084, 2923, 2864, 2208, 1703, 1632, 1588, 1241. 1H NMR (400 MHz, DMSO-d6) δ (ppm): 7.84–7.78 (m, 3H), 7.74 (d, 1H, J = 6.8 Hz), 7.35 (d, 1H, J = 1.6 Hz), 7.07 (dd, 1H, J = 1.6 Hz, J = 8.4 Hz), 6.96 (d, 1H, J = 8.4 Hz), 6.29 (s, 2H, NH2, D2O exchangeable), 5.63 (s, 1H, vinylic), 3.72 (d, 1H, J = 12.4 Hz, –CH), 2.97 (m, 1H, –CH), 2.19–2.07 (m, 2H, CH2), 1.62 (m, 1H, CH2), 1.36 (m, 1H, CH2), 1.17–1.14, (m, 1H, CH2), 0.70 (q, 1H, J = 12.8 Hz, CH2). Anal. calcd for C25H18Cl2N2O2: C, 66.83; H, 4.04; Cl, 15.78; N, 6.23; O, 7.12; found: C, 66.82; H, 4.02; Cl, 15.77; N, 6.20; O, 7.10.
3.5.3 3′-Amino-1,3-dioxo-1′-(p-tolyl)-1,3,6′,7′,8′,8a′-hexahydro-1′H-spiro[indene-2,2′-naphthalene]-4′-carbonitrile (5c). Yellow solid: m.p: 225–228 (Decompose). IR (cm−1): 3409, 3345, 3244, 3020, 2922, 2201, 1704, 1657, 1587, 1243. 1H NMR (400 MHz, DMSO-d6) δ (ppm): 7.75 (m, 3H), 7.66 (d, 1H, J = 7.2 Hz), 6.77–6.73 (m, 3H), 6.60 (d, 1H, J = 8 Hz), 6.15 (s, 2H, NH2, D2O exchangeable), 5.59 (s, 1H, vinylic), 3.07 (m, 1H, –CH), 2.97 (d, 1H, J = 12.4 Hz, –CH), 2.19–2.15 (m, 2H, CH2), 1.99 (s, 3H, –CH3), 1.63 (m, 1H, CH2), 1.38–1.31 (m, 2H, CH2), 0.71 (q, 1H, J = 13.2 Hz, CH2). Anal. calcd for C26H22N2O2: C, 79.16; H, 5.62; N, 7.10; O, 8.11; found: C, 79.17; H, 5.63; N, 7.11; O, 8.09.
3.5.4 3′-Amino-1′-(4-fluorophenyl)-1,3-dioxo-1,3,6′,7′,8′,8a′-hexahydro-1′H-spiro[indene-2,2′-naphthalene]-4′-carbonitrile (5d). Yellow solid: m.p.: 265–275 (Decompose). IR (cm−1): 3448, 3361, 2911, 2198, 1736, 1695, 1591, 1234. 1H NMR (400 MHz, DMSO-d6) δ (ppm): 7.76 (d, 3H, J = 8.4 Hz), 7.67 (d, 1H, J = 6.4 Hz), 6.87–6.86 (m, 1H), 6.79 (d, 3H, J = 8.4 Hz), 6.20 (s, 2H, NH2, D2O exchangeable), 5.61 (s, 1H, vinylic), 3.07 (m, 2H, 2CH), 2.20–2.16 (m, 1H, CH2), 2.07–2.04 (m, 1H, CH2), 1.65 (m, 1H, CH2), 1.40 (m, 1H, CH2), 1.34–1.31 (m, 1H, CH2), 0.72 (q, 1H, J = 11.6 Hz, CH2). 13C NMR (100 MHz, DMSO-d6) δ (ppm): 199.60, 199.18, 166.33, 159.78, 151.25, 142.87, 142.08, 136.22, 136.11, 132.89, 132.81, 131.93, 131.15, 128.36, 123.28, 122.70, 117.01, 115.00, 80.98, 63.15, 51.20, 32.99, 27.31, 24.93, 21.56. Anal. calcd for C25H19FN2O2: C, 75.36; H, 4.81; F, 4.77; N, 7.03; O, 8.03; found: C, 75.36; H, 4.80; F, 4.75; N, 7.04; O, 8.01.
3.5.5 3′-Amino-1′-(4-(methylthio)phenyl)-1,3-dioxo-1,3,6′,7′,8′,8a′-hexahydro-1′H-spiro[indene-2,2′-naphthalene]-4′-carbonitrile (5e). Yellow solid: m.p.: 240–248 (Decompose). IR (cm−1): 3422, 3339, 2928, 2202, 1703, 1635, 1589, 1242. 1H NMR (400 MHz, DMSO-d6) δ (ppm): 7.76 (m, 3H), 7.69 (m, 1H), 6.87–6.83 (m, 2H), 6.77 (d, 1H, J = 7.6 Hz), 6.67 (d, 1H, J = 7.6 Hz), 6.17 (s, 2H, NH2, D2O exchangeable), 5.60 (s, 1H, vinylic), 3.05 (m, 1H, –CH), 2.99 (d, 1H, J = 12.8 Hz, –CH), 2.24 (s, 3H, –SCH3), 2.15 (m, 1H, CH2), 2.07 (m, 1H, CH2), 1.64 (m, 1H, CH2), 1.39–1.30 (m, 2H, CH2), 0.72 (q, 1H, J = 11.6 Hz, CH2). 13C NMR (100 MHz, DMSO-d6) δ (ppm): 200.13, 199.68, 151.84, 143.37, 142.66, 137.65, 136.67, 136.54, 132.75, 132.02, 131.76, 127.33, 126.28, 125.87, 123.83, 123.22, 118.08, 117.47, 82.93, 63.61, 52.01, 33.52, 27.88, 25.44, 22.08, 15.01. Anal. calcd for C26H22N2O2S: C, 73.21; H, 5.20; N, 6.57; O, 7.50; S, 7.52; found: C, 73.22; H, 5.25; N, 6.51; O, 7.47; S, 7.51.
3.5.6 3′-Amino-1′-(4-isopropylphenyl)-1,3-dioxo-1,3,6′,7′,8′,8a′-hexahydro-1′H-spiro[indene-2,2′-naphthalene]-4′-carbonitrile (5f). Yellow solid: m.p: 232–240 (Decompose). IR (cm−1): 3432, 3343, 3248, 2954, 2197, 1702, 1648, 1586, 1241. 1H NMR (400 MHz, DMSO-d6) δ (ppm): 7.70–7.69 (m, 3H), 7.63 (m, 1H), 6.79–6.76 (m, 3H), 6.61 (d, 1H, J = 7.6 Hz), 6.18 (s, 2H, NH2, D2O exchangeable), 5.61 (s, 1H, vinylic), 3.08 (m, 1H, CH), 2.99 (d, 1H, J = 12.8 Hz, CH), 2.57 (m, 1H, CH(CH3)2), 2.21–2.17 (m, 1H, CH2), 2.09 (m, 1H, CH2), 1.67 (m, 1H, CH2), 1.44–1.42 (m, 2H, CH2), 0.90 (d, 6H, 2CH3), 0.75 (q, 1H, J = 11.6 Hz, CH2). Anal. calcd for C28H26N2O2: C, 79.59; H, 6.20; N, 6.63; O, 7.57; found: C, 79.57; H, 6.22; N, 6.58; O, 7.56.
3.5.7 3′-Amino-1′-(4-methoxyphenyl)-1,3-dioxo-1,3,6′,7′,8′,8a′-hexahydro-1′H-spiro[indene-2,2′-naphthalene]-4′-carbonitrile (5g). Yellow solid: m.p.: 250–255 (Decompose). IR (cm−1): 3424, 3341, 3245, 2920, 2199, 1701, 1654, 1510, 1242. 1H NMR (400 MHz, DMSO-d6) δ (ppm): 7.75–7.74 (m, 2H), 7.69–7.66 (m, 2H), 6.74 (d, 1H, J = 8.8 Hz), 6.64 (d, 1H, J = 8.4 Hz), 6.54–6.49 (m, 2H), 6.14 (s, 2H, NH2, D2O exchangeable), 5.59 (s, 1H, vinylic), 3.50 (s, 3H, –OCH3), 3.02 (m, 1H, CH), 2.97 (d, 1H, J = 12.4 Hz, CH), 2.20–2.15 (m, 1H, CH2), 2.07 (m, 1H, CH2), 1.63 (m, 1H, CH2), 1.34–1.28 (m, 2H, CH2), 0.71 (q, 1H, J = 12.8 Hz, CH2). Anal. calcd for C26H22N2O3: C, 76.08; H, 5.40; N, 6.82; O, 11.69; found: C, 76.06; H, 5.41; N, 6.80; O, 11.67.
3.5.8 3′-Amino-1,3-dioxo-1′-(m-tolyl)-1,3,6′,7′,8′,8a′-hexahydro-1′H-spiro[indene-2,2′-naphthalene]-4′-carbonitrile (5h). Yellow solid: m.p.: 210–219 (Decompose). IR (cm−1): 3442, 3345, 2915, 2196, 1700, 1636, 1593, 1240. 1H NMR (400 MHz, DMSO-d6) δ (ppm): 7.68–7.60 (m, 4H), 6.86–6.80 (m, 1H), 6.69–6.64 (m, 1H), 6.62–6.60 (m, 1H), 6.51–6.49 (m, 1H), 6.18 (d, 2H, NH2, D2O exchangeable), 5.64 (s, 1H, vinylic), 3.05 (m, 1H, CH), 2.98 (d, 1H, J = 11.6 Hz, CH), 2.20 (m, 1H, CH2), 2.15 (m, 1H, CH2), 2.04 (d, 3H, –CH3), 1.64 (m, 1H, CH2), 1.39–1.35 (m, 2H, CH2), 0.73 (q, 1H, J = 12.4 Hz, CH2). 13C NMR (100 MHz, DMSO-d6) δ (ppm): 200.16, 199.54, 152.01, 143.47, 142.89, 137.58, 136.47, 136.28, 132.05, 132.13, 131.83, 128.74, 128.37, 127.89, 123.49, 122.83, 118.15, 117.40, 82.89, 63.92, 52.84, 33.43, 27.95, 25.46, 21.30, 18.21. Anal. calcd for C26H22N2O2: C, 79.16; H, 5.62; N, 7.10; O, 8.11; found: C, 79.18; H, 5.63; N, 7.08; O, 8.10.
3.5.9 3′-Amino-1′-(3-chlorophenyl)-1,3-dioxo-1,3,6′,7′,8′,8a′-hexahydro-1′H-spiro[indene-2,2′-naphthalene]-4′-carbonitrile (5i). Yellow solid: m.p.: 235–243 (Decompose). IR (cm−1): 3447, 3354, 3245, 2913, 2198, 1696, 1635, 1585, 1239. 1H NMR (400 MHz, DMSO-d6) δ (ppm): 7.81–7.72 (m, 4H), 6.98 (m, 1H), 6.80 (m, 1H), 6.72 (d, 2H, J = 5.2 Hz), 6.23 (d, 2H, NH2, D2O exchangeable), 5.61 (s, 1H, vinylic), 3.10 (m, 1H, CH), 3.05 (d, 1H, J = 12 Hz, CH), 2.20–2.16 (m, 1H, CH2), 2.06 (m, 1H, CH2), 1.65 (m, 1H, CH2), 1.43 (m, 1H, CH2), 1.34–1.31 (m, 1H, CH2), 0.74 (q, 1H, J = 11.6 Hz, CH2). 13C NMR (100 MHz, DMSO-d6) δ (ppm): 200.79, 199.34, 151.59, 143.31, 142.54, 138.72, 136.68, 133.51, 131.07, 130.46, 127.70, 126.70, 125.69, 123.80, 123.24, 122.91, 118.03, 117.64, 82.92, 63.54, 51.95, 33.31, 27.72, 25.41, 21.98. Anal. calcd for C25H19ClN2O2: C, 72.37; H, 4.62; Cl, 8.55; N, 6.75; O, 7.71; found: C, 72.34; H, 4.63; Cl, 8.54; N, 6.76; O, 7.72.
3.5.10 3′-Amino-1′-(4-chlorophenyl)-1,3-dioxo-1,3,6′,7′,8′,8a′-hexahydro-1′H-spiro[indene-2,2′-naphthalene]-4′-carbonitrile (5j). Yellow solid: m.p.: 250–260 (Decompose). IR (cm−1): 3449, 3357, 3247, 2913, 2199, 1737, 1696, 1635, 1588, 1241. 1H NMR (400 MHz, DMSO-d6) δ (ppm): 7.77 (d, 3H, J = 9.6 Hz), 7.69 (d, 1H, J = 7.2 Hz), 7.04 (d, 2H, J = 8.4 Hz), 6.86 (d, 1H, J = 8 Hz), 6.76 (d, 1H, J = 7.2 Hz), 6.22 (s, 2H, NH2, D2O exchangeable), 5.61 (s, 1H, vinylic), 3.06 (m, 2H, 2CH), 2.20–2.15 (m, 1H, CH2), 2.07 (m, 1H, CH2), 1.65 (m, 1H, CH2), 1.42–1.40 (m, 1H, CH2), 1.32–1.29 (m, 1H, CH2), 0.73 (q, 1H, J = 11.6 Hz, CH2). Anal. calcd for C25H19ClN2O2: C, 72.37; H, 4.62; Cl, 8.55; N, 6.75; O, 7.71; found: C, 72.30; H, 4.65; Cl, 8.54; N, 6.73; O, 7.72.
3.5.11 3′-Amino-1,3-dioxo-1′-(thiophen-2-yl)-1,3,6′,7′,8′,8a′-hexahydro-1′H-spiro[indene-2,2′-naphthalene]-4′-carbonitrile (5k). Yellow solid: m.p.: 255–260 (Decompose). IR (cm−1): 3406, 3346, 3245, 2917, 2201, 1703, 1657, 1586, 1245. 1H NMR (400 MHz, DMSO-d6) δ (ppm): 7.82–7.77 (m, 4H), 7.09 (s, 1H), 6.61 (s, 1H), 6.54 (s, 1H), 6.18 (s, 2H, NH2, D2O exchangeable), 5.60 (s, 1H, vinylic), 3.45 (d, 1H, J = 12 Hz, CH), 2.96 (m, 1H, CH), 2.21–2.16 (m, 1H, CH2), 2.09 (m, 1H, CH2), 1.69 (m, 1H, CH2), 1.46 (m, 2H, CH2), 0.84 (q, 1H, J = 12 Hz, CH2). Anal. calcd for C23H18N2O2S: C, 71.48; H, 4.69; N, 7.25; O, 8.28; S, 8.30; found: C, 71.47; H, 4.69; N, 7.27; O, 8.25; S, 8.27.
3.5.12 3′-Amino-1,3-dioxo-1′-phenyl-1,3,6′,7′,8′,8a′-hexahydro-1′H-spiro[indene-2,2′-naphthalene]-4′-carbonitrile (5l). Yellow solid: m.p.: 245–258 (Decompose). IR (cm−1): 3451, 3363, 2910, 2199, 1696, 1635, 1590, 1242. 1H NMR (400 MHz, DMSO-d6) δ (ppm): 7.73 (s, 3H), 7.65–7.64 (m, 1H), 6.95–6.90 (m, 3H), 6.85 (d, 1H, J = 8.4 Hz), 6.72 (d, 1H, J = 7.6 Hz), 6.18 (s, 2H, NH2, D2O exchangeable), 5.60 (s, 1H, vinylic), 3.09–3.07 (m, 1H, CH), 3.02 (d, 1H, J = 12 Hz, CH), 2.21–2.16 (m, 1H, CH2), 2.08 (m, 1H, CH2), 1.64 (m, 1H, CH2), 1.41–1.33 (m, 2H, CH2), 0.73 (q, 1H, J = 13.2 Hz, CH2). 13C NMR (100 MHz, DMSO-d6) δ (ppm): 199.63, 199.21, 166.44, 151.41, 142.88, 142.17, 136.07, 135.93, 135.68, 131.00, 128.53, 128.08, 127.32, 126.31, 123.22, 122.64, 117.64, 116.91, 80.99, 63.19, 52.07, 32.92, 27.40, 24.95, 21.59. Anal. calcd for C25H20N2O2: C, 78.93; H, 5.30; N, 7.36; O, 8.41; found: C, 78.91; H, 5.29; N, 7.37; O, 8.40.
4. Conclusion
In conclusion, Fe3O4@SiO2-L-tryptophan magnetic nanocatalyst as green, magnetically recyclable, and environmentally friendly, was prepared and fully characterized. We applied this catalyst for the synthesis of spiro[indene-2,2′-naphthalene]-4′-carbonitrile derivatives via a four-component reaction of malononitrile, cyclohexanone, aromatic aldehydes and 1,3-indandione. Excellent yields in short reaction time, facile, soft reaction conditions, and high atom economy are some of the advantages of this procedure.
Conflicts of interest
There are no conflicts to declare.
Acknowledgements
The authors are so grateful to Kashan University for the financial support to run this project.
Notes and references
- H. A. Younus, M. Al-Rashida, A. Hameed, M. Uroos, U. Salar, S. Rana and K. M. Khan, Expert Opin. Ther. Pat., 2021, 31, 267–289 CrossRef CAS PubMed.
- E. Ruijter and R. V. A. Orru, Drug Discovery Today: Technol., 2013, 10, e15–e20 CrossRef PubMed.
- J. Luo, G. S. Chen, S. J. Chen, Z. D. Li and Y. L. Liu, Chem.–Eur. J., 2021, 27, 6598–6619 CrossRef CAS.
- C. M. R. Volla, I. Atodiresei and M. Rueping, Chem. Rev., 2014, 114, 2390–2431 CrossRef CAS PubMed.
- A. N. Komogortsev, V. G. Melekhina, B. V. Lichitsky and M. E. Minyaev, Tetrahedron Lett., 2020, 61, 152384 CrossRef CAS.
- A. Chaudhary, Mol. Diversity, 2021, 25, 1211–1245 CrossRef CAS PubMed.
- L. Biesen and J. J. Muller, Adv. Synth. Catal., 2020, 363, 980–1006 CrossRef.
- P. Dhokne, A. P. Sakla and N. Shankaraiah, Eur. J. Med. Chem., 2021, 216, 113334 CrossRef CAS PubMed.
- S. Sampath, M. Vadivelu, R. Ravindran, P. T. Perumal, V. Velkannan and K. Karthikeyan, ChemistrySelect, 2020, 5, 2130–2134 CrossRef CAS.
- A. Toumi, S. Boudriga, K. Hamden, M. Sobeh, M. Cheurfa, M. Askri, M. Knorr, C. Strohmann and L. Brieger, Bioorg. Chem., 2020, 106, 104507 CrossRef PubMed.
- C. Yan, J. Sun and C. G. Yan, Chin. Chem. Lett., 2021, 32, 1253–1256 CrossRef CAS.
- B. P. Kaur, J. Kaur and S. Singh Chimni, RSC Adv., 2021, 11, 2126–2140 RSC.
- K. S. Mani, W. Kaminsky and S. P. Rajendran, New J. Chem., 2018, 42, 301–310 RSC.
- S. C. Zhan, R. J. Fang, J. Sun and C. G. Yan, J. Org. Chem., 2021, 86, 8726–8741 CrossRef CAS PubMed.
- C. S. Sankara and I. N. N. Namboothiri, Org. Lett., 2021, 23, 4618–4623 CrossRef PubMed.
- N. Kaur, P. Singh and P. Banerjee, Adv. Synth. Catal., 2021, 363, 2813–2824 CrossRef CAS.
- S. Nagaraju, K. Sathish, N. Satyanarayana, B. Paplal and D. Kashinath, J. Heterocycl. Chem., 2020, 57, 469–476 CrossRef CAS.
- N. Sudhapriya, P. T. Perumal, C. Balachandran, S. Ignacimuthu, M. Sangeetha and M. Doble, Eur. J. Med. Chem., 2014, 83, 190–207 CrossRef CAS PubMed.
- G. Khanna, P. Saluja and J. M. Khurana, Tetrahedron Lett., 2016, 57, 5852–5855 CrossRef CAS.
- T. Lohar, A. Kumbhar, A. Patil, S. Kamat and R. Salunkhe, Res. Chem. Intermed., 2019, 45, 1639–1651 CrossRef CAS.
- I. Fatimah, G. Fadillah and S. P. Yudha, Arabian J. Chem., 2021, 14, 103301 CrossRef CAS.
- X. Li, W. Li, M. Wang and Z. Liao, J. Controlled Release, 2021, 335, 437–448 CrossRef CAS PubMed.
- C. Sun, J. S. H. Lee and M. Zhang, Adv. Drug Delivery Rev., 2008, 60, 1252–1265 CrossRef CAS PubMed.
- R. B. N. Baig and R. S. Varma, Chem. Commun., 2013, 49, 752–770 RSC.
- M. M. Yallapu, S. F. Othman, E. T. Curtis, B. K. Gupta, M. Jaggi and S. C. Chauhan, Biomaterials, 2011, 32, 1890–1905 CrossRef CAS PubMed.
- S. Liu, B. Yu, S. Wang, Y. Shen and H. Cong, Adv. Colloid Interface Sci., 2020, 281, 102165 CrossRef CAS PubMed.
- G. C. Lavorato, R. Das, J. A. Masa, M. H. Phan and H. Srikanth, RSC Adv., 2021, 3, 867–888 CAS.
- J. Deng, L. P. Mo, F. Y. Zhao, Z. H. Zhang and S. X. Liu, ACS Comb. Sci., 2012, 14, 335–341 CrossRef CAS PubMed.
- H. Sepehrmnsourie, M. Zarei, M. A. Zolfigol, S. Babaee and S. Rostamnia, Sci. Rep., 2021, 11, 5279 CrossRef PubMed.
- S. Liu, B. Yu, S. Wang, Y. Shen and H. Cong, Adv. Colloid Interface Sci., 2020, 281, 102165 CrossRef CAS PubMed.
- Z. Jiang, H. Yang, X. Han, J. Luo, M. W. Wong and Y. Lu, Org. Biomol. Chem., 2010, 8, 1368–1377 RSC.
- S. Luo, X. Mi, L. Zhang, S. Liu, H. Xu and J.-P. Cheng, Angew. Chem., Int. Ed., 2006, 45, 3093–3097 CrossRef CAS PubMed.
- M. Gruttadauria, F. Giacalone, A. M. Marculescu and R. Notoa, Adv. Synth. Catal., 2008, 350, 1397–1405 CrossRef CAS.
- A. Zamboulis, N. J. Rahier, M. Gehringer, X. Cattoën, G. Niel, C. Bied, J. J. E. Moreau and M. W. C. Man, Tetrahedron: Asymmetry, 2009, 20, 2880–2885 CrossRef CAS.
- A. Ghorbani-Choghamarani and G. Azadi, Appl. Organomet. Chem., 2016, 30, 247–252 CrossRef CAS.
- J. M. Gonzalez-Domínguez, M. Gonzalez, A. Anson-Casaos, A. M. Díez-Pascual, M. A. Gomez and M. T. Martínez, J. Phys. Chem. C, 2011, 115, 7238–7248 CrossRef.
- Solvents and Solvent Effects in Organic Chemistry, C. Reichardt, WILEY-VCH Verlag GmbH & Co. KGaA, copyright 8, third edn, 2003, Weinheim, ISBN: 3-527-30618-8 Search PubMed.
- F. Alemi-Tameh, J. Safaei-Ghom, M. Mahmoudi-Hashemi and H. Shahbazi-Alavi, RSC Adv., 2016, 6, 74802–74811 RSC.
- H. S. Oboudatian, H. Naeimi and M. Moradian, RSC Adv., 2021, 11, 7271–7279 RSC.
- J. Safaei-Ghom, R. Masoomi, M. Hamadanian and S. Naseh, New J. Chem., 2016, 40, 3289–3299 RSC.
- E. Eidi, M. Z. Kassaee, Z. Nasresfahani and P. T. Cummings, Appl. Organomet. Chem., 2018, 32, e4573 CrossRef.
Footnote |
† Electronic supplementary information (ESI) available: FT-IR, 1H-NMR, and 13C NMR spectroscopy data. See DOI: 10.1039/d1ra07654j |
|
This journal is © The Royal Society of Chemistry 2022 |