DOI:
10.1039/D1RA07511J
(Paper)
RSC Adv., 2022,
12, 15980-15985
Synthesis and characterization of a Cu(II) coordination-containing TAM radical as a nitroxyl probe†
Received
11th October 2021
, Accepted 14th April 2022
First published on 27th May 2022
Abstract
Nitroxyl (HNO) has been identified as an important signaling molecule in biological systems, and it plays critical roles in many physiological processes. However, its detection remains challenging because of the limited sensitivity and/or specificity of existing detection methods. Low-frequency electron paramagnetic resonance (EPR) spectroscopy and imaging, coupled with the use of exogenous paramagnetic probes, have been indispensable techniques for the in vivo measurement of various physiological parameters owing to their specificity, noninvasiveness and good depth of magnetic field penetration in animal tissues. However, the in vivo detection of HNO levels by EPR spectroscopy and imaging is limited due to the need for improved probes. We report the first “turn on-response” EPR probe for HNO utilizing a Cu(II) coordination-containing TAM radical (denoted as CuII[TD1]). Upon reaction with HNO, CuII[TD1] shows a 16.1-fold turn-on in EPR signal with a low detection limit of 1.95 μM. Moreover, low-temperature EPR spectroscopic and ESI-MS studies showed that the sensing mechanism relies on the reduction of Cu(II) by HNO. Lastly, CuII[TD1] is selective for HNO over other reactive nitrogen and oxygen species except for some reductants (Cys and Asc). This new Cu(II) coordination-containing TAM radical shows great potential for in vivo EPR HNO applications in the absence of reducing agents and provides insights into developing improved and targeted EPR HNO probes for biomedical applications.
1. Introduction
Nitric oxide (NO) has received considerable attention due to its role as an active signal-inducing messenger biomolecule in immune systems.1 Nitroxyl (HNO) is the one-electron reduced and protonated product of nitric oxide (NO), which is a well-known signaling molecule in many physiological processes. Despite their very close structural similarity, it has recently been found that HNO shows different biological and chemical properties.2–5 HNO has biological activity. It has been theorized to be a potent cytotoxic agent that consumes cellular glutathione, causes double-stranded breaks in DNA,6 and elicitates smooth muscle relaxation.7 Recent studies on the biological role of HNO divulge that this species also has the ability to increase the cardiac output through the decrement of venous resistance, and as a result, HNO has been recognized to serve as a potential therapeutic agent for the treatment of myocardial ischemia–reperfusion injury4 and cardiovascular disorders.8 Although some progress regarding the biological chemistry of HNO has been achieved, the physiological and pathological effects of HNO still remain largely undiscovered. Thus, the sensitive and reliable detection of HNO in in vitro and in vivo systems is of paramount importance to understand its roles in normal physiology and disease.
Traditional methodologies for the detection of HNO are based on the analytical techniques of mass spectrometry,9 electrochemical analysis,10 high-performance liquid chromatography (HPLC),11 and the colorimetric method.12 Over the past decades, fluorescence methods have been widely used for HNO detection.13–16 Several fluorescent probes based on Cu(II) complexes with a tripodal dipicolylamine (DPA)-appended receptor have been recently developed for the detection of biological HNO.17–19 However, these methods are mostly limited to in vitro or ex vivo detection due to their invasiveness and/or insufficient light penetration into tissues.
In recent years, great progress in low frequency electron paramagnetic resonance (EPR) instrumentation20,21 has been achieved, which allows for the in vivo measurement and mapping of different physiological parameters such as oxygen,22 redox status,23,24 pH25 and reactive oxygen species26 in isolated tissues and living animals. However, the full potential of this technique is still far from being realized due in part to the lack of spin probes that are stable, sensitive to HNO, have narrow linewidth (ΔBpp), and have target specificity to penetrate and localize within cells.
Recently, tetrathiatriarylmethyl (TAM) radicals (OX063, CT-03, see Chart 1) have received wide attention as EPR probes owing to their high biostability and narrow singlet EPR signal at physiological pH, thereby providing more than 15-fold higher sensitivity and 200-fold improved time resolution for EPRI applications as compared to nitroxides.27–31 While the field of TAM probe development is still in its infancy, TAM radicals and their derivatives have been utilized to measure extracellular32 and intracellular28,33 oxygen levels, superoxide radical anions,34 pH,35,36 as well as redox status.37,38 Most recently, TAM radicals have shown great potential in proton electron double resonance imaging39 or PEDRI (also known as Overhauser magnetic resonance imaging or OMRI).40 In the present study, we propose a novel reaction-based “turn on-response” EPR probe for HNO utilizing a Cu(II) coordination-containing TAM radical (denoted as CuII[TD1], see Chart 1). We hypothesized that HNO could rapidly reduce Cu(II) to Cu(I), resulting in the disappearance of the strong intramolecular spin exchange interaction, which would restore the EPR signal. This probe was found to provide excellent sensitivity and specificity for HNO detection. Therefore, our results represent the first example for the detection of HNO by the EPR method through a TAM-based probe.
 |
| Chart 1 Molecular structures of HNO probes containing a tripodal dipicolylamine (DPA)-appended receptor. | |
2. Experimental section
2.1. EPR experiments and spectral simulation
EPR measurements were carried out on a Bruker EMX-plus X-band spectrometer at room temperature. The general instrumental settings were as follows: modulation frequency, 30–100 kHz; microwave power, 0.05–1 mW; modulation amplitude, 0.03–0.08 G. Measurements were performed in 50 μL capillary tubes. In addition, EPR measurements under anaerobic conditions were carried out using a gas-permeable Teflon tube (i.d. = 0.8 mm). Briefly, the experimental solution was transferred to the tube, which was then sealed at both ends. The sealed sample was placed inside a quartz EPR tube with open ends. Argon gas was bled into the EPR tube and the EPR spectrum was recorded after a 30 min equilibrium.
EPR spectral simulation was conducted by a home-made EPR simulation program (ROKI\EPR) developed by Antal Rockenbauer.41 The EPR experiments and spectral simulation technology were supported by Prof. Liu YP's group (Tianjin Key Laboratory on Technologies Enabling Development of Clinical Therapeutics and Diagnostics, School of Pharmacy, Tianjin Medical University, Tianjin 300070, P. R. China).
2.2. Stability studies towards biological oxidoreductants
Solutions of H2O2 (1 mM) in PBS buffer (pH 7.4, 50 mM) were used. ClO− was generated from NaClO. NO2− was generated from NaNO2. NO3− was generated from NaNO3. Alkylperoxyl radicals (ROO˙) were generated by the thermolysis of 2,2′-azobis-2-methylpropanimidamide dihydrochloride (AAPH, 1 mM) at 37 °C. Peroxynitrite (ONOO−) was generated by the decomposition of SIN-1 (1 mM) at 37 °C in the presence of SOD (50 U mL−1). Hydroxyl radicals (HO˙) were continuously generated from the system consisting of Fe(III)–NTA (0.1 mM) and H2O2 (1 mM). Superoxide (O2˙−) was generated using the xanthine (X)/xanthine oxidase (XO) system using XO (20 mU mL−1) and X (0.4 mM) in the presence of DTPA (0.1 mM). Nitric oxide (NO) was generated from a saturated NO aqueous solution (2 mM). Nitroxyl donor (HNO) was generated from sodium trioxodinitrate (Na2N2O3, Angeli's salt). Angeli's salt was prepared as described by King and Nagasawa, and was stored dry at −20 °C until needed.42 EPR spectra were recorded 30 min after mixing the TAM radical solution (20 μM) with various oxidoreductants. The effect of various reactive species on the TAM radical was expressed as a percentage of TAM radical remaining after exposure to the reactive species for 30 min, which was obtained by the double integral of the EPR signal. Each experiment was conducted three times.
3. Results and discussion
3.1. Synthesis
The synthesis of compound 2 proceeded as described in the literature with some minor changes (Scheme 1). Compound 1 was converted directly to the desired thioacetonide 2 by condensation with acetone in the presence of BF3·Et2O. Compound 1 was further converted into the intermediate thioacetonide 2 by heating under reflux with acetone. BF3·Et2O and chloroform were used as the catalyst and solvent, respectively, instead of HBF4 and toluene, which was recommended by the literature source.43 The treatment of arene 2 with 1 equiv. n-BuLi followed by 0.32 equiv. diethyl carbonate afforded trityl alcohol 3. Following the earlier method, trityl alcohol 3 was deprotonated with tert-BuLi and TMEDA, and the resultant anion was treated with excess di-tert-butyl dicarbonate (DIBOC) to afford the triester 4. The triester 4 was reduced partially by LiAlH4 to the corresponding benzyl alcohol 5. Then, the reaction of 5 with benzenesulfonyl chloride in the presence of N-methyl pyrrolidone led to the corresponding benzyl chloride 6. Subsequently, the SN2 reaction of 6 with di-(2-picolyl)amine afforded compound 7. Trityl TD1 was obtained by treatment of 7 with TFA/DCM. Finally, CuII[TD1] was generated by the addition of CuCl2 to TD1.
 |
| Scheme 1 Synthesis of CuII[TD1]. | |
3.2. EPR spectra of TD1
Fig. 1 shows the experimental and simulated EPR spectra of TD1 in PBS buffer under anaerobic conditions, which exhibit a symmetrical sextet signal with peak-to-peak line widths (56 mG) due to hyperfine splittings (hfs) from the two unequivalent protons on the methylene group and nitrogen nuclei on the DPA group. The simulation of the EPR spectra is in excellent agreement with the experimental spectra, which demonstrates the values of hfs for nitrogen and methylene hydrogen nuclei: aH1(CH2) = 2.69 G, aH2(CH2) = 0.06 G and aN = 0.91 G.
 |
| Fig. 1 Experimental (black solid line) and simulated (red dotted line) EPR spectra of TD1 (10 μM) in PBS buffer (50 mM, pH 7.4) under anaerobic conditions. Spectral parameters: microwave power, 0.63 mW; time constant, 20.48 ms; conversion time, 80.0 ms; sweep time, 81.92 s; frequency, 9.3 GHz; modulation amplitude, 0.1 G; sweep width, 8 G; number of points, 1024. Dotted lines represent the calculated EPR spectra with peak-to-peak line widths, ΔHL = 56 mG, and the following hfs constants: aH1(CH2) = 2.69 G, aH2(CH2) = 0.06 G and aN = 0.91 G. | |
3.3. Reaction of CuII[TD1] with HNO
To check if CuII[TD1] can function as a HNO detection probe, its reactivity with HNO was investigated by EPR. Compared with the EPR signal of TD1, CuII[TD1] showed dramatic EPR signal quenching (17.8-fold) (Fig. S1†). Firstly, the concentration-dependent EPR signal of CuII[TD1] towards HNO was investigated. The EPR signal intensity increased steadily with increase in the Angeli's salt (Na2N2O3, a HNO donor) concentration (Fig. 2) until it reached a plateau at 20 μM Na2N2O3, which corresponded to a 16.1-fold increase in the EPR signal intensity compared to that of blank [HNO]. This indicates that the complete reduction of CuII[TD1] occurred with 20 μM Na2N2O3. In addition, the EPR signal response of CuII[TD1] to HNO exhibited a linear relationship in the range of 0–20 μM HNO (Fig. S3†), and its detection limit was determined to be 1.95 μM (3s/k). Then, the time-dependent EPR signal of CuII[TD1] was further obtained by incubating CuII[TD1] (1 μM) with HNO (30 μM). The EPR intensity of CuII[TD1] increased steadily with an increase in the reaction time until it reached a maxima at 25 min, which corresponded to a 16.1-fold increase (Fig. 3). Moreover, the reaction of CuII[TD1] with HNO was corroborated by the ESI-MS spectra of the probe CuII[TD1] and CuI[TD1] (Fig. S15 and S16†). As shown in Fig. S15,† a major peak at m/z 1226.9742 (calcd. 1226.9797) corresponding to [TD1 + Cu(I)]+ was observed when 30 μM Na2N2O3 was added. By comparison, the probe CuII[TD1] without HNO only exhibited a peak at m/z 1263.7606 (calcd. 1263.7657), which corresponded to [TD1 + Cu(II) + Cl]+ (Fig. S16†). Low-temperature X-band EPR spectroscopy of CuII[TD1] provided further evidence for the reduction of the paramagnetic CuII[TD1] complex by HNO (Fig. S2†). In order to detect the EPR signal of CuII, the EPR signal of CuII[TD1] was measured under high mw power, high modulation amplitude and low temperature. As shown in Fig. S2,† the EPR spectra of CuII[TD1] in EtOH exhibit a rhombic signal (LW = 470 G), which disappeared upon treatment with 30 equiv. HNO, as expected for the reduction of Cu(II) to Cu(I). Thus, low-temperature EPR spectroscopic and ESI-MS studies showed that the sensing mechanism relies on the reduction of Cu(II) by HNO. Taken together, CuII[TD1] was sensitive to HNO and could be used to quantitate HNO.
 |
| Fig. 2 (A) Concentration-dependent EPR spectra obtained by incubating CuII[TD1] (1 μM) with different amounts of Angeli's salt (Na2N2O3, a HNO donor) in PBS buffer (50 mM, pH 7.4) at room temperature. (B) Variation of EPR signal double integration (triangle) with different concentrations of Angeli's salt. Ef/Ei represent the final (Ef) over the initial (Ei) double integral of the EPR signal. | |
 |
| Fig. 3 (A) Time-dependent EPR spectra obtained by incubating CuII[TD1] (1 μM) with HNO (30 μM) in PBS buffer (50 mM, pH 7.4) at room temperature. (B) Variation of EPR signal double integration (triangle) with time in the presence of HNO. Ef/Ei represents the final (Ef) over the initial (Ei) double integral of the EPR signal. | |
3.4. Selectivity of CuII[TD1] toward HNO
While CuII[TD1] was highly sensitive to HNO, it was important to investigate its selectivity with various ROS and RNS species (Fig. 4). The probe exhibited a 16-fold increase upon interaction with HNO. However, a much weaker response was observed with other biologically relevant ROS and RNS species, including NO3−, ClO−, H2O2, HO˙, O2˙−, ONOO−, ROO˙, and NO2−. With NO, a 2.1-fold increase in EPR intensity was observed, and this relative lack of induced fluorescence response could be used to discriminate between NO and HNO. These results indicate that CuII[TD1] shows high selectivity towards HNO over other ROS and RNS species. In addition, submillimolar cysteine and sodium ascorbate could also be used to restore the EPR signal intensity (12.5-fold and 14.2-fold, see Fig. S7 in the ESI†) because of the reduction of chelated Cu(II)–DPA. To improve the stability and selectivity of TAM-based HNO probes, the Cu(II)–DPA group was replaced with a Cu(II)–azamacrocyclic group in the following work.44
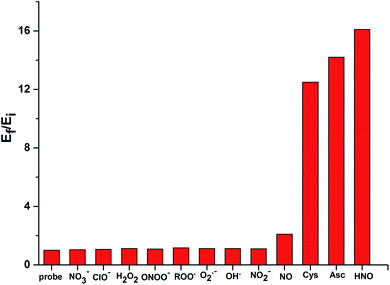 |
| Fig. 4 RPR responses of CuII[TD1] (1 μM) to various 30 μM ROS and RNS species. Bars represent the final (Ef) over the initial (Ei) double integral of the EPR signal. | |
4. Conclusions
In summary, a Cu(II) coordination-containing TAM radical (CuII[TD1]) was developed that acted as a turn on-response probe towards HNO for EPR detection. To the best of our knowledge, this is the first study showing the measurement of HNO by the EPR method using a TAM-based probe. However, the limitation of CuII[TD1] is that reducing agents of biological origin such as cysteine and ascorbic acid can induce EPR signal turn-on, which limits its application in vivo. For future in vivo applications, the stability and selectivity of TAM-based HNO probes have been improved by replacing the Cu(II)–DPA group with a Cu(II)–azamacrocyclic group; the biocompatibility needs to be further improved possibly through PEGylated dendritic encapsulation;45 and lastly, the replacement of the methylene group with amido linkage would make the EPR signal of this probe much simpler and sharper. Overall, this new probe provides a powerful tool for noninvasively measuring HNO in a wide variety of chemical and biological systems, and provides important insights into the design of TAM-based HNO probes with improved properties.
Conflicts of interest
There are no conflicts to declare.
Acknowledgements
This work was financially supported by the Science and Technology Plan Projects of Health Commission of Jiangxi Province (20201117), the National Natural Science Foundation of China (21967013, 21867013), the Natural Science Foundation of Jiangxi Province (20192BAB215043, 2019BAB205106, 20212BAB206082) and the Science and Technology Project of Jiangxi Administration of Traditional Chinese Medicine (2021Z017).
References
- J. M. Zimmet and J. M. Hare, Circulation, 2006, 114, 1531–1544 CrossRef PubMed.
- K. M. Miranda, N. Paolocci, T. Katori, D. D. Thomas, E. Ford, M. D. Bartberger, M. G. Espey, D. A. Kass, M. Feelisch, J. M. Fukuto and D. A. Wink, Proc. Natl. Acad. Sci. U. S. A., 2003, 100, 9196–9201 CrossRef CAS PubMed.
- N. Paolocci, W. F. Saavedra, K. M. Miranda, C. Martignani, T. Isoda, J. M. Hare, M. G. Espey, J. M. Fukuto, M. Feelisch, D. A. Wink and D. A. Kass, Proc. Natl. Acad. Sci. U. S. A., 2001, 98, 10463–10468 CrossRef CAS PubMed.
- J. C. Irvine, R. H. Ritchie, J. L. Favaloro, K. L. Andrews, R. E. Widdop and B. K. Kemp-Harper, Trends Pharmacol. Sci., 2008, 29, 601–608 CrossRef CAS PubMed.
- D. D. Thomas, L. A. Ridnour, J. S. Isenberg, W. Flores-Santana, C. H. Switzer, S. Donzelli, P. Hussain, C. Vecoli, N. Paolocci, S. Ambs, C. A. Colton, C. C. Harris, D. D. Roberts and D. A. Wink, Free Radical Biol. Med., 2008, 45, 18–31 CrossRef CAS PubMed.
- D. A. Wink, M. Feelisch, J. Fukuto, D. Chistodoulou, D. Jourd'heuil, M. B. Grisham, Y. Vodovotz, J. A. Cook, M. Krishna, W. G. DeGraff, S. Kim, J. Gamson and J. B. Mitchell, Arch. Biochem. Biophys., 1998, 351, 66–74 CrossRef CAS PubMed.
- J. M. Fukuto, K. Chiang, R. Hszieh, P. Wong and G. Chaudhuri, J. Pharmacol. Exp. Ther., 1992, 263, 546–551 CAS.
- J. L. Favaloro and B. K. Kemp-Harper, Cardiovasc. Res., 2007, 73, 587–596 CrossRef CAS PubMed.
- M. R. Cline, C. Tu, D. N. Silverman and J. P. Toscano, Free Radical Biol. Med., 2011, 50, 1274–1279 CrossRef CAS PubMed.
- S. A. Suarez, M. H. Fonticelli, A. A. Rubert, E. de la Llave, D. Scherlis, R. C. Salvarezza, M. A. Marti and F. Doctorovich, Inorg. Chem., 2010, 49, 6955–6966 CrossRef CAS PubMed.
- S. Donzelli, M. G. Espey, D. D. Thomas, D. Mancardi, C. G. Tocchetti, L. A. Ridnour, N. Paolocci, S. B. King, K. M. Miranda, G. Lazzarino, J. M. Fukuto and D. A. Wink, Free Radical Biol. Med., 2006, 40, 1056–1066 CrossRef CAS PubMed.
- F. Doctorovich, P. J. Farmer and M. A. Marti, HNO Generation From NO, Nitrite, Inorganic or Organic Nitrosyls, and Crosstalk With H2S[M], Elsevier, Amsterdam, 2017 Search PubMed.
- Z. Liu and Q. Sun, Spectrochim. Acta, Part A, 2020, 241, 118680 CrossRef CAS PubMed.
- R. Smulik-Izydorczyk, K. Debowska, J. Pieta, R. Michalski, A. Marcinek and A. Sikora, Free Radical Biol. Med., 2018, 128, 69–83 CrossRef CAS PubMed.
- B. Dong, X. Kong and W. Lin, ACS Chem. Biol., 2018, 13, 1714–1720 CrossRef CAS PubMed.
- P. Rivera-Fuentes and S. J. Lippard, Acc. Chem. Res., 2015, 48, 2927–2934 CrossRef CAS PubMed.
- Y. Zhou, K. Liu, J. Y. Li, Y. Fang, T. C. Zhao and C. Yao, Org. Lett., 2011, 13, 1290–1293 CrossRef CAS PubMed.
- J. Rosenthal and S. J. Lippard, J. Am. Chem. Soc., 2010, 132, 5536–5537 CrossRef CAS PubMed.
- H. J. Lv, R. F. Ma, X. T. Zhang, M. H. Li, Y. T. Wang, S. Wang and G. W. Xing, Tetrahedron, 2016, 72, 5495–5501 CrossRef CAS.
- M. Elas, B. B. Williams, A. Parasca, C. Mailer, C. A. Pelizzari, M. A. Lewis, J. N. River, G. S. Karczmar, E. D. Barth and H. J. Halpern, Magn. Reson. Med., 2003, 49, 682–691 CrossRef PubMed.
- A. Samouilov, G. L. Caia, E. Kesselring, S. Petryakov, T. Wasowicz and J. L. Zweier, Magn. Reson. Med., 2007, 58, 156–166 CrossRef PubMed.
- M. Elas, K. H. Ahn, A. Parasca, E. D. Barth, D. Lee, C. Haney and H. J. Halpern, Clin. Cancer Res., 2006, 12, 4209–4217 CrossRef CAS PubMed.
- F. Hyodo, K. H. Chuang, A. G. Goloshevsky, A. Sulima, G. L. Griffiths, J. B. Mitchell, A. P. Koretsky and M. C. Krishna, J. Cereb. Blood Flow Metab., 2008, 28, 1165–1174 CrossRef CAS PubMed.
- H. M. Swartz, N. Khan and V. V. Khramtsov, Antioxid. Redox Signaling, 2007, 9, 1757–1771 CrossRef CAS PubMed.
- V. V. Khramtsov, I. A. Grigor'ev, M. A. Foster and D. J. Lurie, Antioxid. Redox Signaling, 2004, 6, 667–676 CrossRef CAS PubMed.
- H. J. Halpern, C. Yu, E. Barth, M. Peric and G. M. Rosen, Proc. Natl. Acad. Sci. U. S. A., 1995, 92, 796–800 CrossRef CAS PubMed.
- I. Dhimitruka, M. Velayutham, A. A. Bobko, V. V. Khramtsov, F. A. Villamena, C. M. Hadad and J. L. Zweier, Bioorg. Med. Chem. Lett., 2007, 17, 6801–6805 CrossRef CAS PubMed.
- Y. Liu, F. A. Villamena, J. Sun, Y. Xu, I. Dhimitruka and J. L. Zweier, J. Org. Chem., 2008, 73, 1490–1497 CrossRef CAS PubMed.
- Y. Liu, F. A. Villamena and J. L. Zweier, Chem. Commun., 2008, 4336–4338, 10.1039/b807406b.
- A. A. Bobko, I. Dhimitruka, T. D. Eubank, C. B. Marsh, J. L. Zweier and V. V. Khramtsov, Free Radical Biol. Med., 2009, 47, 654–658 CrossRef CAS PubMed.
- I. Dhimitruka, O. Grigorieva, J. L. Zweier and V. V. Khramtsov, Bioorg. Med. Chem. Lett., 2010, 20, 3946–3949 CrossRef CAS PubMed.
- V. K. Kutala, N. L. Parinandi, R. P. Pandian and P. Kuppusamy, Antioxid. Redox Signaling, 2004, 6, 597–603 CrossRef CAS PubMed.
- Y. Liu, F. A. Villamena, J. Sun, T. Y. Wang and J. L. Zweier, Free Radical Biol. Med., 2009, 46, 876–883 CrossRef CAS PubMed.
- Y. Liu, Y. Song, F. De Pascali, X. Liu, F. A. Villamena and J. L. Zweier, Free Radical Biol. Med., 2012, 53, 2081–2091 CrossRef CAS PubMed.
- A. A. Bobko, I. Dhimitruka, J. L. Zweier and V. V. Khramtsov, J. Am. Chem. Soc., 2007, 129, 7240–7241 CrossRef CAS PubMed.
- I. Dhimitruka, A. A. Bobko, C. M. Hadad, J. L. Zweier and V. V. Khramtsov, J. Am. Chem. Soc., 2008, 130, 10780–10787 CrossRef CAS PubMed.
- Y. Liu, F. A. Villamena, A. Rockenbauer and J. L. Zweier, Chem. Commun., 2010, 46, 628–630 RSC.
- Y. Liu, F. A. Villamena, Y. Song, J. Sun, A. Rockenbauer and J. L. Zweier, J. Org. Chem., 2010, 75, 7796–7802 CrossRef CAS PubMed.
- Z. Y. Yang, Y. P. Liu, P. Borbat, J. L. Zweier, J. H. Freed and W. L. Hubbell, J. Am. Chem. Soc., 2012, 134, 9950–9952 CrossRef CAS PubMed.
- M. C. Krishna, S. English, K. Yamada, J. Yoo, R. Murugesan, N. Devasahayam, J. A. Cook, K. Golman, J. H. Ardenkjaer-Larsen and S. Subramanian, Proc. Natl. Acad. Sci. U. S. A., 2002, 99, 2216–2221 CrossRef CAS PubMed.
- A. Rockenbauer and L. Korecz, Appl. Magn. Reson., 1996, 10, 29–43 CrossRef CAS.
- S. B. King and H. T. Nagasawa, Methods Enzymol., 1999, 301, 211 CAS.
- O. Y. Rogozhnikova, V. G. Vasiliev, T. I. Troitskaya, D. V. Trukhin, T. V. Mikhalina, H. J. Halpern and V. M. Tormyshev, Eur. J. Org. Chem., 2013, 2013, 3347–3355 CrossRef CAS PubMed.
- K. Sasakura, K. Hanaoka, N. Shibuya, Y. Mikami, Y. Kimura, T. Komatsu, T. Ueno, T. Terai, H. Kimura and T. Naganot, J. Am. Chem. Soc., 2011, 133, 18003–18005 CrossRef CAS PubMed.
- W. Liu, J. Nie, X. Tan, H. Liu, N. Yu, G. Han, Y. Zhu, F. A. Villamena, Y. Song and J. L. Zweier, J. Org. Chem., 2016, 82, 588–596 CrossRef PubMed.
|
This journal is © The Royal Society of Chemistry 2022 |
Click here to see how this site uses Cookies. View our privacy policy here.