DOI:
10.1039/D1RA06488F
(Paper)
RSC Adv., 2022,
12, 1228-1237
Improvement of cytotoxicity and necrosis activity of ganoderic acid a through the development of PMBN-A.Her2-GA as a targeted nano system
Received
28th August 2021
, Accepted 11th December 2021
First published on 5th January 2022
Abstract
The targeting nano carriers have been promptly proposed to overcome the current obstacles in conventional chemotherapy approaches for cancer. Currently, PMBN (poly[MPC-co-(BMA)-co-(MEONP)]), is considered as a promising amphiphilic polymer that could be easily targeted and conjugated to hydrophobic substances with low bioavailability. To target breast cancer cells overexpressing human epidermal receptor 2 (HER2) receptors, anti-HER2 monoclonal antibody (A.Her2) was conjugated to PMBN and afterward loaded with ganoderic acid A (GA-A) as an anti-cancer metabolite. The efficacy of conjugation and loading was reasonably favourable. The rod shape of the polymer with a size of approximately 160 ± 30 nm was confirmed. Our results indicated that PMBN-A.Her2-GA is an anionic nanostructure with an appropriate form and capable of being applied in cancer therapy. Subsequently, cytotoxicity analysis revealed an improved anti-proliferative effect of GA-A.
1. Introduction
Cancer is considered one of the world's leading causes of death.1 Recent reports estimate the total global cost associated with cancer therapy to be a hundred billion US$.2 Due to the growing side effects of conventional chemotherapeutic approaches, there is more attention to finding alternative ways for improving the immune system to target the advanced stages of tumors.3 Several natural herbal components have been conventionally applied to treat various disorders for a long time. Current investigations have been focused on natural extracts of fungi, particularly those with low cellular toxicity for normal tissues. It should be noted that not only efficacy but also the cost-effectiveness of any investigation for a new therapeutic approach should be considered in cancer therapy to determine the cancer-related health care cost. Ganoderma lucidum (G. lucidum), normally raised as Lingzhi in China, is a fungal material that has been applied extensively in Asian nations for routine treatments.4 Its pharmacological properties include immune-modulating, anti-inflammatory, anti-cancer, anti-diabetic, anti-oxidative, radical-scavenging, and anti-aging effects.5–7 G. lucidum, contains more than 90% water, carbohydrate, crude fat, fibre, and protein8. Moreover, it consists of diverse bioactive components such as terpenoids, steroids, phenols, glycoproteins, and polysaccharides.9 It has been reported that triterpenes and polysaccharides are the main physiologically active ingredients of G. lucidum.10 The growth of cell lines such as the human prostate PC-3 and bladder cancers has been reported to be inhibited following the exposure to the G. lucidum extract.11–13 Furthermore, ganoderic acid inhibits cell proliferation and induced apoptosis in the human colon and breast carcinoma cell lines.14–21
Breast cancer is one of the most common cancers in the world. Recently, targeted therapy with antibodies or in combination with nano carriers has been regarded as a novel therapeutic approach to specifically target tumour markers.22 Trastuzumab (Herceptin) is the first recombinant human-specific IgG1 monoclonal antibody applied in HER2 overexpressed breast cancer patients.23 HER2 is a tyrosine kinase from the family of epidermal growth factor receptors or EGFRs24 involved in various signalling pathways and considered promising targets in breast cancer therapeutic approaches.25 HER2 plays a vital role in controlling growth, survival, and cell differentiation.26 Recombinant mAbs such as Trastuzumab and Pertuzumab are commercially approved for targeting Her2 in breast cancer therapy.27,28 Despite all advantages in the clinic, frequent mutations and subsequent monoclonal antibody resistance through activation of alternative pathways might occur.27,29,30 Hence, there is a crucial need for improved clinical efficiency of anti-Her2 mAb. Targeted therapy with nanotechnology as drug delivery has been considered a promising approach.31,32 Following this technology, the drug dose and toxicity in healthy tissues will be reduced, whereas anti-tumour efficacy will be enhanced.33–35 In drug delivery systems (DDSs), biopolymers or biocompatible polymers have been extensively applied.36–40 2-Methacryloyloxyethyl phosphorylcholine (MPC) polymer is a biocompatible polymer with the same polar group (phosphorylcholine group) as biomembranes and thus lacks any protein absorption.41 MPC polymers have been applied as surface modifiers in numerous medical apparatus to develop biocompatibility.42 The MPC polymers are extremely hydrophilic due to their n-butyl methacrylate units; therefore, copolymers such as MPC could be water-soluble. As a result, hydrophobic monomer units might be involved in the solubilisation of hydrophobic agents and improve their water solubility and bioavailability.43 Due to the incorporation of hydrophobic substances by MPC, the possibility of their application as a carrier for non-soluble substances in aqueous media has been investigated.41,44 Poly[MPC-co-(BMA)-co-(MEONP)] (PMBN) is a part of MPC polymer with active ester groups capable of being conjugated to various proteins under moderate physiological conditions.45 PMBN might be a promising tool for targeted therapy through proper ligands or antibody conjugation. In the current study, growth inhibition and antitumor characteristics of GA-A in combination with anti-Her2 mAb-conjugated PMBN (PMBN-A.Her2-GA) on HER2 overexpressed SKBR3 cell lines in comparison to Her2 negative MCF7 cell lines were investigated. Briefly, PMBN as an amphiphilic and targetable polymer was conjugated with anti-Her2 mAb and loaded with GA-A to target Her2 overexpressed breast cancer cell lines directly and deliver GA-A as a fungal anti-tumor agent (Fig. 1).
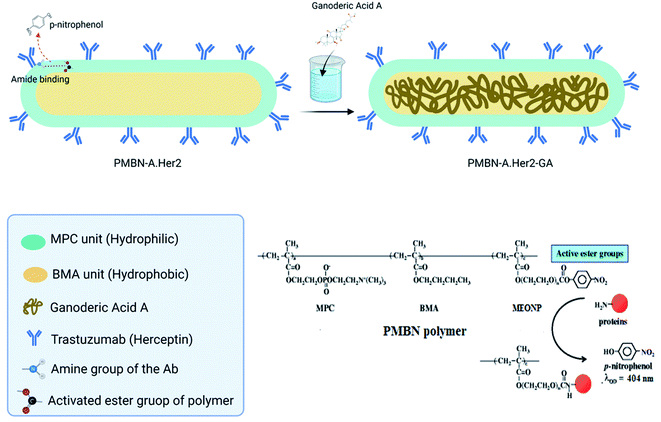 |
| Fig. 1 The conjugation of PMBN to anti-Her2 mAb, loaded with GA-A, a fungal anti-tumour agent. Anti-Her2 mAbs are conjugated to MPC units as hydrophilic units and GA-A is attached to the BMA units due to its hydrophobicity. | |
2. Materials and methods
2.1. Materials
RPMI 1640 cell culture and Fetal Bovine Saline (FBS) were provided from Sigma-Aldrich Co., Germany. Amicon filter was procured from Merck Millipore Co., USA, ganoderic acid A from BOC Science Co., USA, and anti-HER2 mAb Trastuzumab (Herceptin®) was purchased from Aryogen Pharmed (Karaj, Iran).
3. Methods
3.1. Targetable amphiphilic nano polymer, PMBN
PMBN polymer has been developed in our previous study with the composition of 40 mol% MPC, 50 mol% BMA, and 10 mol% of MEONP.46 The structure of the polymer was confirmed by Fourier transform infrared spectroscopy (FT-IR MB102, BOMEM Inc., Switzerland) using the KBr pellet technique. Proton nuclear magnetic resonance device (H-NMR DRX 300, Bruker Avance Co., USA) was applied by CDCI3 as solvent and tetramethylsilane (TMS) as standard internal material. Moreover, dynamic light scattering measurement (DLS, Nano-ZS, Malvern Co., UK) was applied to calculate the size of nanoparticles (NPs), size distribution, and zeta potential of the NPs' surface. Subsequently, a scanning electron microscope (SEM VEGA II, Tescan Co., Czech Republic) and transmission electron microscope imaging system (TEM EM10C-80 KV, Zeiss Co., Germany) evaluated the shape and size of the developed NPs.
3.2. Conjugation of PMBN-antiHer2mAb
2 mg of polymer with 30 μl concentration of Trastuzumab (Herceptin®) Aryogen Pharmed (Karaj, Iran) was added to PBS at pH ∼7.4, and thoroughly mixed at 4 °C for 48 h. Afterward, the obtained mixture was centrifuged (rpm: 5000 g, time: 13 min) using a 30 KDa Amicon falcon filter (Amicon® Ultra-15 device, Merck Millipore Ltd, USA) to remove unbound antibodies and p-nitrophenol groups. The efficiency of filtration was checked via absorption spectroscopy at 280 and 404 nm for anti-Her2 mAb and p-nitrophenol, respectively, via a UV-Vis spectrophotometer (Nanodrop 2000C, Thermo Scientific Co, USA). In that case, anti-her2 mAb would make an amide binding with the active ester group of MEONP monomer through the free –NH2 group, and p-nitrophenol will be released. To determine the amide binding and efficiency, the following solutions were prepared:
(1) 1 mg of PMBN in 1 ml of NaOH (0.2 mol ml−1), (2) 1 mg of PMBN in 1 ml of water, and (3) 1 mg of PMBN-A.Her2 in 1 ml of water. The absorbance of each solution was measured at 404 nm to evaluate the efficiency of conjugation by a spectrophotometer, and the amide binding was analysed by FT-IR measurement.
3.3. Incorporation of conjugated PMBN-antiHer2mAb with GA-A
The dissolved GA-A (1 ml) in ethanol was added to PMBN-A.Her2-GA conjugates and mixed gently at room temperature. Amicon falcon filtered the solution, and the loading efficiency was analysed through the absorbance measurement at 255 nm. The size and zeta potential of PMBN-A.Her2-GA were determined by DLS, and also the structure and size of the nano system were investigated by field emission scanning electron microscope (FESEM MIRA3, Tescan Co., Czech Republic) with a magnification of 700
000.
3.4. Functional activity of conjugated PMBN-A.Her2-GA
3.4.1. Cytotoxicity. The functional activity of our developed nano system on SKBR3 cells (HER2 over-expressing breast cancer cell line) was evaluated in comparison to the MCF7 cell line as the negative control. Briefly, the SKBR3 and MCF7 cells (University of Tehran, Tehran, Iran) were (5 × 103 cells per well) seeded into 96-well plates and incubated for 24 h. Next, free GA-A, PMBN-GA, and our developed PMBN-A.Her2-GA was suspended within the 10% v/v serum added, and the cells were incubated at 37 °C. Cell viability was investigated through cell staining (dead cells in red and live cells in green) (Life Technologies, USA) following days 1 and 7 using 1 μM calcein AM and 2 μM ethidium homodimer-3 in culture medium stained cells, which were observed under fluorescent microscopy (Leica DM IRB).
3.4.2. Metabolic activity of cells. Metabolic activity (cells proliferation) was evaluated using the Alamar Blue assay (Resazurin Cell Viability Assay Kit, Biotium, USA) following days 1 and 7. In brief, 10% v/v resazurin solution was added to each sample mentioned above and incubated at 37 °C for 3 h. Then, 100 μl of supernatant of incubated samples was transferred to a 96-well plate, and fluorescence emission intensity was quantified using a micro-plate fluorescence reader (λex 560 nm, λem 590 nm, BioTek Instruments Inc., Synergy 4, USA).
3.4.3. Cell apoptosis analysis. SKBR3 and MCF7 cell lines were cultured in RPMI medium supplemented with 10% fetal bovine serum at 37 °C. Briefly, cells were seeded at 106 cells per ml to 96-well plates with 10% FBS containing 5% CO2 and incubated overnight at 37 °C. The next day, free GA-A, PMBN-GA-A, and our developed PMBN-A.Her2-GA were added to each well. After 24 and 48 h of incubation, the apoptosis rate of cells in response to cognate treatment was analyzed using Annexin V Apoptosis Detection Kit (KeyGEN Biotech Co., Ltd, Nanjing, China) according to the instruction. Subsequently, the cells were analyzed by FACS caliber (FACScan, BD Biosciences, Franklin Lakes, NJ, USA) programmed with CellQuest software (BD Biosciences, Franklin Lakes, NJ, USA).
3.4.4. Statistical analysis. Statistical analysis was performed using GraphPad Prism 5 software. One-way ANOVA and Tukey's multiple comparison tests were used to compare multiple groups. P < 0.05 was considered to be statistically significant. All experiments were performed at least in triplicate.
4. Results and discussions
4.1. Characterization of PMBN polymer
First, the polymer was analysed by FT-IR and H-NMR methods. The specific peak at 1727 cm−1 was related to the carbonyl group, the peak at 1086 cm−1 was for (–POCH2–), and the 964 cm−1 for (N+(CH3)3) group. Furthermore, the MPC monomer peaks at 1.81 ppm for (CH3–C), at 4–4.3 ppm for (N+CCH2−, POCH2C, –OCH2CH2OP–), and at 3.5 ppm for (N+CH3) were determined by H-NMR analysis. BMA block peaks were at 1.0 ppm for (CH3–C), at ∼4 ppm for (O(CH2)3–C), and at 1.3 ppm for (C–CH3). Finally, the MEONP monomer was confirmed by peaks at ∼0.8 ppm for (CH3–C), 3.1 ppm for (OCH2CH2O), and 7.0 and 8.1 ppm for protons of the aromatic ring. All the desired structures of the polymer were as expected as confirmed. In the next step, the size and zeta potential of the polymer were analyzed by DLS. The analysis revealed −8.7 mV for the surface charge, which indicates an anionic polymer. Following conjugation and incorporation, the enhanced or reduced values should be measured to evaluate the quality of the constructed nano system. Moreover, the size of 52.18 nm and the Poly Disparity Index (PDI) of 0.11 confirmed the polymer as a nano polymer with “monodisperse” characteristics. The size and structure of the polymer were checked with SEM and TEM microscopy. Results indicate the dimensions of 40 nm for polymer. The variance between DLS, SEM, and TEM data was probably due to the larger hydrodynamic diameter in DLS analysis, which was typically larger than the diameters determined by SEM and TEM microscopy as a capping agent. The synthesized polymer was notably rod-shaped. Rouslahti et al. reported that in contrast to spherical NPs, rod-shaped NPs (nano rods) show high endothelial preference in both in vitro and in vivo.47 Furthermore, such particles seem to be superior for targeting in tumor xenografts.48 Thus, this type of structure might be a promising candidate to design an appropriate nano carrier for DDS.39,40
4.2. Conjugation of polymer and mAb
After the conjugation of the polymer with anti-Her2 mAb, the solution was filtered. The absorbance of flow-through was measured at 280 and 404 nm to evaluate any unbound antibody and p-nitrophenol, respectively, which confirmed the accuracy of our filtration step. The results confirmed that no free p-nitrophenol or anti-Her2 in the solution that could interfere with subsequent studies or possibly have toxic effects were found (not shown). To measure the efficiency of conjugation, three solutions were prepared, as mentioned before. The polymer in NaOH solution, leading to the total release of the p-nitrophenol group, can be considered as a reference value. However, due to hydrolysis, fewer groups will be released from the polymer in water. Consequently, the remaining p-nitrophenol after conjugation will be released following the hydrolysis. After the absorbance measurement at 404 nm (specific for free p-nitrophenol) according to Fig. 1, and by the calculation 1 below, the efficacy of conjugation was calculated to be 72.1% and confirmed (Fig. 2). |
 | (1) |
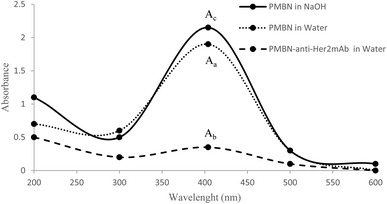 |
| Fig. 2 The absorbance of free p-nitrophenol in three different solutions, Aa, Ab, and Ac, to measure the efficiency of the PMBN-anti-Her2mAb conjugate. | |
In addition, the FT-IR analysis of conjugation showed a peak at 1637 cm−1, which is specific for amide binding and indicates the interaction between active ester groups of MEONP monomer and free –NH2 of anti-Her2mAb (Fig. 3). Hence, it can be concluded that the polymer can be conjugated with any biomolecules with free –NH2, and it is considered a potential candidate to target desired cancerous cells.
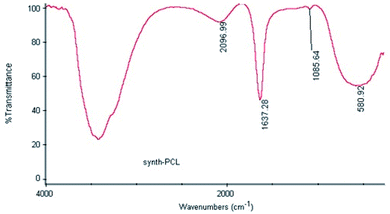 |
| Fig. 3 FT-IR analysis showed a specific amide-binding peak at 1637 cm−1 and confirmed the interaction of the MEONP monomer with anti-Her2mAb. | |
4.3. GA-A loading to PMBN-anti Her2 mAb conjugate
To investigate the efficiency of incorporation, the concentration of GA-A in the solution of PMBN-A.Her2 was analyzed at 255 nm in comparison to GA-A before incorporation. As shown in Fig. 4, and through the following eqn (2), the efficacy of loading was approximately 77.5%. According to this result, GA-A as a hydrophobic agent with less than 1% water solubility, became soluble in combination with the amphiphilic polymer. As a result, this polymer might be applied as an appropriate candidate for incorporating hydrophobic drugs or agents.49,50 |
 | (2) |
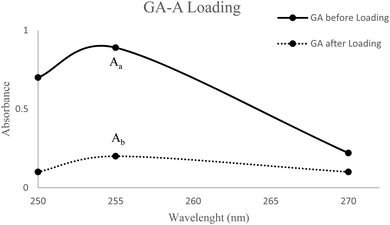 |
| Fig. 4 Evaluation of GA-A incorporation into conjugated NPs. The absorbance of conjugated NPs before and subsequent GA-A loading was measured at 255 nm, as shown, and the reduced absorbance confirmed the accuracy of incorporation. | |
4.4. Characterization of synthesized nano system, PMBN-A.Her2-GA
The synthesized nano system was analyzed by DLS. The specifications of this nano carrier were compared with PMBN, as depicted in Table 1. Following the conjugation and loading, results obtained from the FESEM microscope indicate the enhanced size of the polymer from 52 nm to 280 nm. Furthermore, the PDI was still under 0.5, which is acceptable for a nano system. It should be noted that not only does the polymer remain anionic, but also the zeta potential (ZP) increased, remarkably. Due to electrostatic interactions, more cellular uptake of nanoparticles (NPs) with ZP-43 mV compared to NPs with lower ZP or with positive ZP has been demonstrated in various studies.51 Generally, cellular absorption of these particles is because of (1) non-specific absorption of NPs on cell membrane (2) formation of NPs cluster. Limbach et al. found that the human lung fibroblast cells rapidly absorb NPs with negative ZP.52 The absorption of negative NPs on the positive sites of the cell membrane by electrostatic interactions leads to neutralization and subsequently makes the plasma membrane induce the endocytosis phenomena.53 It has been demonstrated that aggregation of NP with negative surface charge and approximate size of 150 nm at the tumor sites is superior. These results prompted us to develop nano carriers for pharmaceutical applications with improved therapeutic effects.54 Comparison of two positive and negative NPs with the same values of ZP demonstrated the preference of phagocytosis systems to cationic particles regardless of their constructions.55 In another investigation, a comparison of cerium oxide NPs with positive and negative surface charges confirmed the high tendency of protein absorption with the positive ones,51 which could be an excellent challenge for DDSs.
Table 1 Comparison of size, zeta potential, and PDI of NPs before and after incorporation with GA-A
|
PMBN |
PMBN-A.Her2-GA |
Size |
52.18 nm |
279.8 nm |
Zeta potential |
−8.47 mV |
−43.8 mV |
PDI |
0.116 |
0.436 |
On the other hand, the zeta potential is a critical factor for the stability of NPs solutions. Due to the repulsive forces, the high surface electrical charge of NPs leads to no agglomeration in buffer solutions.56,57 According to the theory of DLVO, the stability of solutions and colloids results from the balance between attraction and repulsion of van der walls forces of surface net charges. If the ZP value is below the specific amount, the particles tend to be agglomerated. In contrast, for ZP above ±30 mv, the system tends to be stable.58,59 The ZP is an important physicochemical parameter for the stability of nano solutions, and the minimum acceptable ZP value for static and electrostatic stability is ±20 mv.
Furthermore, the results of FESEM microscopy are shown in Fig. 5. The rod-shaped structure of the nano system confirmed the intact polymer conformation subsequent to conjugation and loading. As mentioned before, rod-shaped structures are more effective and successful than other nano structures.47,48 In addition, the size of the targeted nano system was approximately 160 ± 30 nm, which is a desirable size for a nano system in drug delivery applications. Our result is consistent with DLS analysis (the difference relates to hydrodynamic diameter, as mentioned).
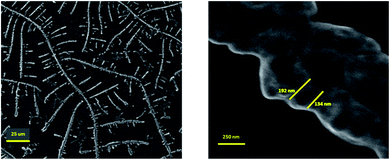 |
| Fig. 5 The results of the FESEM microscopy. The rod-shaped structure of the constructed PMBN-A.Her2-GA confirmed the intact polymer conformation subsequent to conjugation and loading. The left and the right magnification is 1k× and 100k×, respectively, and confirmed the rod-shaped structure with the size of 160 ± 30 nm. | |
4.5. Cytotoxicity analysis of the nano system
According to IC50 of PMBN-A.Her2-GA compared to GA-A solution as shown in Fig. 6, it was concluded that targeting the polymer with anti-Her2mAb leads to enhanced cytotoxicity of the fungal metabolite up to 75%. Reduced IC50 of PMBN-A.Her2-GA compared to each agent at SKBR3 as positive cell line was more intuitive than MCF7 as negative cell line due to potent targeted DDS. Furthermore, to evaluate the role of targeting, the efficiency of PMBN-A.Her2-GA and PMBN-GA-A were compared to other studies.30,60 No improvement in toxicity of PMBN-GA was shown compared to the targeted nano system. Two crucial factors for improved toxicity of the conjugate system include: (1) enhanced solubility and bioavailability of hydrophobic GA-A metabolite, following its incorporation in PMBN. (2) Specific targeting of desired cells after the conjugation of anti-Her2mAb with PMBN. In another study by Motamed et al., the toxicity of GA-A was reported to be similar to Paclitaxel (PTX) as a conventional chemotherapeutic drug.61
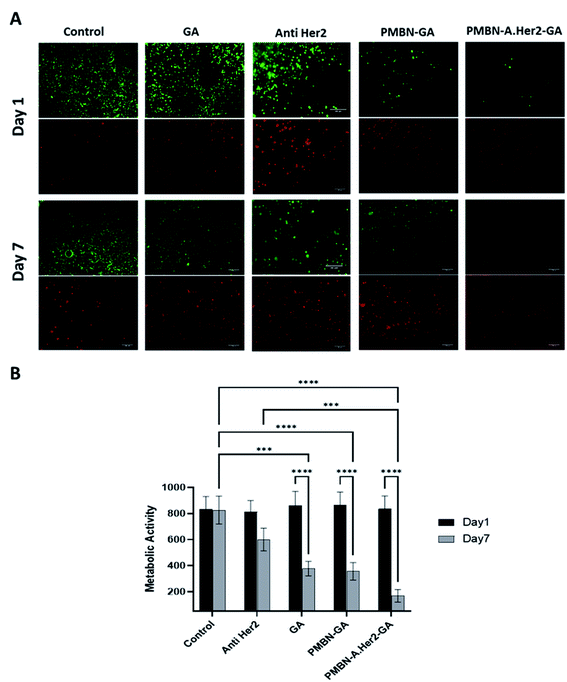 |
| Fig. 6 Cytotoxicity analysis of nano system. (A) Cell viability via fluorescent microscopic images. Following days 1 and 7 under 4 different conditions as shown in the figure. Dead and live cells stained in red and green, respectively (Life Technologies, USA), subsequent to the addition of 1 μM calcein AM and 2 μM ethidium homodimer-3 in the culture medium. (B) Alamar Blue assay, the metabolic activity of cells evaluated at days 1 and 7 (Resazurin Cell Viability Assay Kit, Biotium, USA) (Mean ± SD), (ns: not significant, ***p < 0.001, ****p < 0.0001). | |
The cytotoxicity assays of PMBN and PMBN-A.Her2-GA (Fig. 6 and 7) indicate no toxicity effect of unconjugated PMBN on both cell lines, consistent with previous studies,50 and confirmed the biocompatibility of polymer and nano systems. In addition, our results indicate that anti-Her2 monoclonal antibody (A.Her2) plays an inhibitory role in the proliferation of Her2+ cells. Cancer cell (SKBR3) death is shown in the cell line treated with anti Her2 (Trastuzumab) and decreased metabolic activity and cancer cell proliferation at day 7, but in less extent in comparison with cell line treated with PMBN-A.Her2-GA. Previous studies also showed an increase in the efficiency of Trastuzumab when combined with anti-tumor drugs.62,63 Similarly, PMBN-A.Her2-GA and PMBN-GA demonstrate higher SKBR3 cell apoptosis than Anti Her2, GA, and control groups, as shown in Fig. 7. In particular, PMBN-A.Her2-GA shows ∼30 and 40% early and late apoptosis, respectively, indicating higher cancer cell killing efficiency of the nano system than other groups.
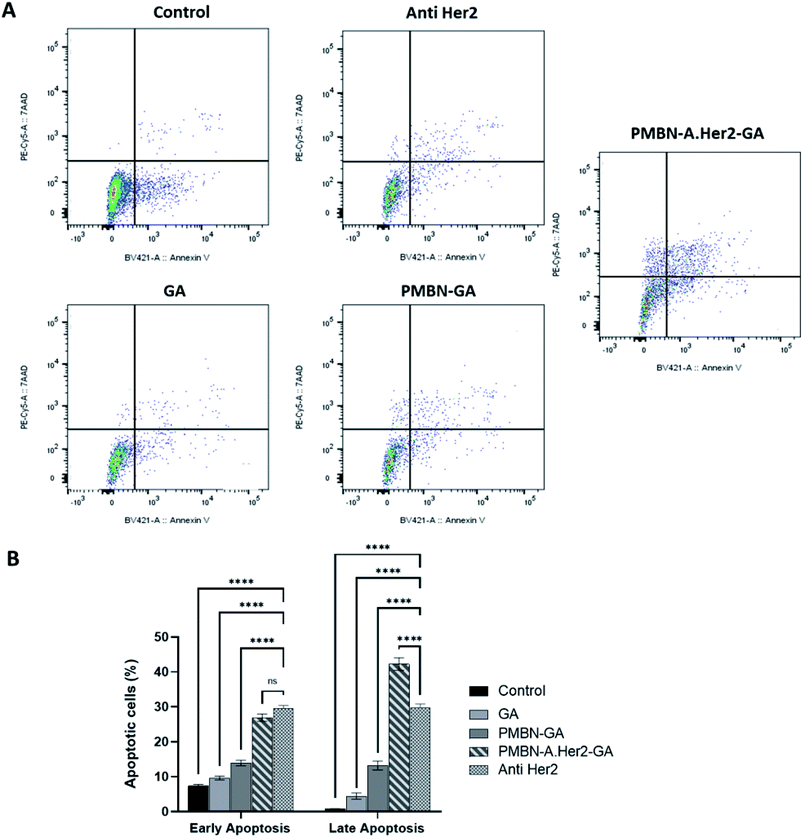 |
| Fig. 7 Cell apoptosis assay. (A) Raw data obtained from flow cytometry of SKBR3 cell line under GA-A, PMBN-GA, and PMBN-A.Her2-GA treatments in comparison to control (MCF7 treated with PMBN-A.Her2-GA). (B) Early and late apoptosis rate of cells (mean ± SD), (****p < 0.0001). As depicted in the figure, PMBN-A.Her2-GA leads to enhanced apoptosis compared to others. | |
One of the limitations in this study is that other toxicities such as reproductive toxicity are essential to investigate. However, GA-A is inherently not toxic since this compound has been a natural material used in Asian countries for many years. In combination with the PMBN nano carrier, to date, no reproductive toxicity has been reported for GA-A. Motamed et al. reported that PMBN is not toxic individually, and there are no side effects.46 Ishihara et al. also noted that this polymer is biocompatible with potential bioapplications.50
5. Conclusion
In our study, the amphiphilic PMBN was conjugated with anti-Her2mAb to directly target HER2+ breast cancer cell line, and anti-tumoral fungal metabolite GA-A was incorporated with this targeted nano DDS for the first time. Following characterization, the rod-shaped structure with an approximate size of 160 ± 30 nm and anionic zeta potential of −43.8 mv was confirmed. The toxicity of PMBN-A.Her2-GA was studied on positive and negative cell lines, which showed more than 50% improved toxicity compared to GA-A. Overall, our developed nano system might be considered a promising candidate for an alternative to conventional chemotherapeutic approaches. In a similar work by Gharbavi et al., a similar system based on PMBN polymer loaded with Paclitaxel agent was used for drug delivery, and in vitro drug release, blood biocompatibility, as well as the cytotoxicity and apoptosis were analyzed that cleared great features of this nano polymer.64
In this regard, further investigation in the animal model of tumors is essential and remains the goal of our future work. The system's stability, like a stress stability test, must be performed to figure out the lifetime of the designed system for further works.
Conflicts of interest
The authors declare no conflict of interest in this study.
Acknowledgements
There is no financial support for this research. Fig. 1 was prepared using http://www.biorender.com.
References
- C. Mattiuzzi and G. Lippi, Current cancer epidemiology, J Epidemiol Glob Health., 2019, 9(4), 217 CrossRef PubMed.
- J. Cortes, J. M. Perez-García, A. Llombart-Cussac, G. Curigliano, N. S. El Saghir and F. Cardoso, et al. Enhancing global access to cancer medicines, CA: Cancer J. Clin., 2020, 70(2), 105–124 Search PubMed.
- S. Poorhosseini, M. Hashemi, O. N. Alipour, A. Izadi, E. Moslemi and Z. Ravesh, et al. New Gene Profiling in Determination of Breast Cancer Recurrence and Prognosis in Iranian Women, Asian Pac J Cancer Prev, 2015, 17, 155–160 CrossRef PubMed.
- Q. Liu and L. Tie. Preventive and therapeutic effect of Ganoderma (Lingzhi) on diabetes. Ganoderma and Health. 2019, pp. 201–15 Search PubMed.
- N. Laçin, S. B. İzol, F. İpek and M. C. Tuncer, Ganoderma lucidum, a promising agent possessing antioxidant and anti-inflammatory effects for treating calvarial defects with graft application in rats1, Acta Cir. Bras., 2019, 34(9), e201900904 CrossRef PubMed.
- P. Rossi, R. Difrancia, V. Quagliariello, E. Savino, P. Tralongo and C. L. Randazzo, et al. B-glucans from Grifola frondosa and Ganoderma lucidum in breast cancer: an example of complementary and integrative medicine, Oncotarget, 2018, 9(37), 24837 CrossRef PubMed.
- D. Sohretoglu and S. Huang, Ganoderma lucidum polysaccharides as an anti-cancer agent, Anti-Cancer Agents Med. Chem., 2018, 18(5), 667–674 CrossRef CAS PubMed.
- S. Sudheer, I. Alzorqi, A. Ali, P. G. Cheng, Y. Siddiqui and S. Manickam, Determination of the biological efficiency and antioxidant potential of Lingzhi or Reishi medicinal mushroom, Ganoderma lucidum (Agaricomycetes), cultivated using different agro-wastes in Malaysia, Int. J. Med. Mushrooms, 2018, 20(1), 89–100 CrossRef PubMed.
- A. Bhardwaj and K. Misra. Therapeutic medicinal mushroom (Ganoderma lucidum): A review of bioactive compounds and their applications. Plant-and Marine-Based Phytochemicals for Human Health. 2018:pp. 191–242 Search PubMed.
- Y. Yang, H. Zhang, J. Zuo, X. Gong, F. Yi and W. Zhu, et al. Advances in research on the active constituents and physiological effects of Ganoderma lucidum, Biomed. Dermatol., 2019, 3(1), 1–17 CrossRef.
- X. Wang, B. Wang, L. Zhou, X. Wang, V. P. Veeraraghavan and S. K. Mohan, et al. Ganoderma lucidum put forth anti-tumor activity against PC-3 prostate cancer cells via inhibition of Jak-1/STAT-3 activity, Saudi J. Biol. Sci., 2020, 27(10), 2632–2637 CrossRef CAS PubMed.
- X. Wang, G. Fang and Y. Pang, Chinese medicines in the treatment of prostate cancer: From formulas to extracts and compounds, Nutrients, 2018, 10(3), 283 CrossRef PubMed.
- J. Yuen, D. Mak, E. Chan, M. Gohel and C. Ng, Tumor inhibitory effects of intravesical Ganoderma lucidum instillation in the syngeneic orthotopic MB49/C57 bladder cancer mice model, J. Ethnopharmacol., 2018, 223, 113–121 CrossRef CAS PubMed.
- J. Jiang, V. Slivova, K. Harvey, T. Valachovicova and D. Sliva, Ganoderma lucidum suppresses growth of breast cancer cells through the inhibition of Akt/NF-κB signaling, Nutr. Cancer, 2004, 49(2), 209–216 CrossRef CAS PubMed.
- H. Hu, N. S. Ahn, X. Yang, Y. S. Lee and K. S. Kang, Ganoderma lucidum extract induces cell cycle arrest and apoptosis in MCF-7 human breast cancer cell, Int. J. Cancer, 2002, 102(3), 250–253 CrossRef CAS PubMed.
- C. I. Müller, T. Kumagai, J. O'Kelly, N. P. Seeram, D. Heber and H. P. Koeffler, Ganoderma lucidum causes apoptosis in leukemia, lymphoma and multiple myeloma cells, Leuk. Res., 2006, 30(7), 841–848 CrossRef PubMed.
- D. Elumalai, T. Suman, M. Hemavathi, C. Swetha, R. Kavitha and C. Arulvasu, et al. Biofabrication of gold nanoparticles using Ganoderma lucidum and their cytotoxicity against human colon cancer cell line (HT-29), Bull. Mater. Sci., 2021, 44(2), 1–6 Search PubMed.
- K. Na, K. Li, T. Sang, K. Wu, Y. Wang and X. Wang, Anticarcinogenic effects of water extract of sporoderm-broken spores of Ganoderma lucidum on colorectal cancer in vitro and in vivo, Int. J. Oncol., 2017, 50(5), 1541–1554 CrossRef CAS PubMed.
- X. Ji, Q. Peng and M. Wang, Anti-colon-cancer effects of polysaccharides: A mini-review of the mechanisms, Int. J. Biol. Macromol., 2018, 114, 1127–1133 CrossRef CAS PubMed.
- A. Barbieri, V. Quagliariello, V. Del Vecchio, M. Falco, A. Luciano and N. J. Amruthraj, et al. Anticancer and anti-inflammatory properties of Ganoderma lucidum extract effects on melanoma and triple-negative breast cancer treatment, Nutrients, 2017, 9(3), 210 CrossRef PubMed.
- Y. Zhang, Ganoderma lucidum (Reishi) suppresses proliferation and migration of breast cancer cells via inhibiting Wnt/β-catenin signaling, Biochem. Biophys. Res. Commun., 2017, 488(4), 679–684 CrossRef CAS PubMed.
- K. Barzaman, J. Karami, Z. Zarei, A. Hosseinzadeh, M. H. Kazemi and S. Moradi-Kalbolandi, et al. Breast cancer: Biology, biomarkers, and treatments, Int. Immunopharmacol., 2020, 84, 106535 CrossRef CAS PubMed.
- J. Molnar Analysis of Organotropic Metastasis in Relation to Herceptin Response of HER2 Positive Breast Cancer Cells, California State University, Northridge; 2017 Search PubMed.
- C. A. Russell, Personalized medicine for breast cancer: it is a new day!, Am. J. Surg., 2014, 207(3), 321–325 CrossRef PubMed.
- R. Butti, S. Das, V. P. Gunasekaran, A. S. Yadav, D. Kumar and G. C. Kundu, Receptor tyrosine kinases (RTKs) in breast cancer: signaling, therapeutic implications and challenges, Mol. Cancer, 2018, 17(1), 1–18 CrossRef PubMed.
- K. Araki and Y. Miyoshi, Mechanism of resistance to endocrine therapy in breast cancer: the important role of PI3K/Akt/mTOR in estrogen receptor-positive, HER2-negative breast cancer, Breast Cancer, 2018, 25(4), 392–401 CrossRef PubMed.
- S. Moradi-Kalbolandi, A. Hosseinzade, M. Salehi, P. Merikhian and L. Farahmand, Monoclonal antibody-based therapeutics, targeting the epidermal growth factor receptor family: from herceptin to Pan HER, J. Pharm. Pharmacol., 2018, 70(7), 841–854 CrossRef CAS PubMed.
- S. Yu, J. Zhang, Y. Yan, X. Yao, L. Fang and H. Xiong, A novel asymmetrical anti-HER2/CD3 bispecific antibody exhibits potent cytotoxicity for HER2-positive tumor cells, J. Exp. Clin. Cancer Res., 2019, 38(1), 1–16 CrossRef CAS PubMed.
- A. Elamir, S. Ajith, N. Al Sawaftah, W. Abuwatfa, D. Mukhopadhyay and V. Paul, Ultrasound-triggered herceptin liposomes for breast cancer therapy, Sci. Rep., 2021, 11(1), 7545 CrossRef CAS PubMed.
- H. H. Oberg, C. Kellner, D. Gonnermann, S. Sebens, D. Bauerschlag and M. Gramatzki, Tribody [(HER2) 2xCD16] is more effective than trastuzumab in enhancing γδ T cell and natural killer cell cytotoxicity against HER2-expressing cancer cells, Front. Immunol., 2018, 9, 814 CrossRef PubMed.
- S. K. Singh, S. Singh, J. W. Licard Jr and R. Singh, Drug delivery approaches for breast cancer, Int. J. Nanomed., 2017, 12, 6205 CrossRef CAS PubMed.
- Y. Yan, X. Cheng, L. Li, R. Zhang, Y. Zhu and Z. Wu, A Novel Small Molecular Antibody, HER2-Nanobody, Inhibits Tumor Proliferation in HER2-Positive Breast Cancer Cells In Vitro and In Vivo, Front. Oncol., 2021, 11, 1724 Search PubMed.
- B. Kim, J. Shin, J. Wu, D. T. Omstead, T. Kiziltepe and L. E. Littlepage, et al. Engineering peptide-targeted liposomal nanoparticles optimized for improved selectivity for HER2-positive breast cancer cells to achieve enhanced in vivo efficacy, J. Controlled Release, 2020, 322, 530–541 CrossRef CAS PubMed.
- R. K. Dhritlahre and A. Saneja, Recent advances in HER2-targeted delivery for cancer therapy, Drug Discovery Today, 2021, 5, 1319–1329 CrossRef PubMed.
- G. Rinnerthaler, S. P. Gampenrieder and R. Greil, HER2 directed antibody-drug-conjugates beyond T-DM1 in breast cancer, Int. J. Mol. Sci., 2019, 20(5), 1115 CrossRef CAS PubMed.
- H. Danafar, A. Sharafi, H. Kheiri Manjili and S. Andalib, Sulforaphane delivery using mPEG–PCL co-polymer nanoparticles to breast cancer cells, Pharmaceutical development and technology, 2016, pp. 1–10 Search PubMed.
- H. K. Manjili, A. Sharafi, H. Danafar, M. Hosseini, A. Ramazani and M. H. Ghasemi, Poly(caprolactone)–poly(ethylene glycol)–poly(caprolactone)(PCL–PEG–PCL) nanoparticles: a valuable and efficient system for in vitro and in vivo delivery of curcumin, RSC Adv., 2016, 6(17), 14403–14415 RSC.
- S. Al-MGA Awi, H. Naderi-Manesh, Z. Mohammad Hassan, H. Yeganeh, S. Nikzad and H. Kheiri Manjili, Construction of Polyurethane Polymeric-based Nano-carriers for Curcumin in Cancer Therapy, Modares Med. Sci.: Pathobiol., 2014, 17(4), 25–39 Search PubMed.
- H. Danafar, H. Manjili and M. Najafi, Study of Copolymer Composition on Drug Loading Efficiency of Enalapril in Polymersomes and Cytotoxicity of Drug Loaded Nanoparticles, Drug Res., 2016, 66(09), 495–504 CrossRef CAS PubMed.
- X. Fang, J. Cao and A. Shen, Advances in anti-breast cancer drugs and the application of nano-drug delivery systems in breast cancer therapy, J. Drug Delivery Sci. Technol., 2020, 57, 101662 CrossRef CAS.
- K. Ishihara, M. Mu and T. Konno, Water-soluble and amphiphilic phospholipid copolymers having 2-methacryloyloxyethyl phosphorylcholine units for the solubilization of bioactive compounds, J. Biomater. Sci., Polym. Ed., 2018, 29(7–9), 844–862 CrossRef CAS PubMed.
- Y. Liu, M. C. Munisso, A. Mahara, Y. Kambe and T. Yamaoka, Anti-platelet adhesion and in situ capture of circulating endothelial progenitor cells on ePTFE surface modified with poly(2-methacryloyloxyethyl phosphorylcholine) (PMPC) and hemocompatible peptide 1 (HCP-1), Colloids Surf., B., 2020, 193, 111113 CrossRef CAS PubMed.
- K. Ishihara, Revolutionary advances in 2-methacryloyloxyethyl phosphorylcholine polymers as biomaterials, J. Biomed. Mater. Res., Part A, 2019, 107(5), 933–943 CrossRef CAS PubMed.
- K. Yoshie, S. Yada, S. Ando and K. Ishihara, Effects of inner polarity and viscosity of amphiphilic phospholipid polymer aggregates on the solubility enhancement of poorly water-soluble drugs, Colloids Surf., B, 2020, 195, 111215 CrossRef CAS PubMed.
- T. Konno, J. Watanabe and K. Ishihara, Conjugation of enzymes on polymer nanoparticles covered with phosphorylcholine groups, Biomacromolecules, 2004, 5(2), 342–347 CrossRef CAS PubMed.
- P. Motamed Fath, F. Yazdian, R. Jamjah, B. Ebrahimi Hosseinzadeh, M. Rahimnezhad and R. Sahraeian, et al. Synthesis and Characterization of PMBN, As a Biocompatible Nano Polymer for Bio-Applications, Cell J., 2017, 19(2), 269–277 Search PubMed.
- P. Kolhar, A. C. Anselmo, V. Gupta, K. Pant, B. Prabhakarpandian and E. Ruoslahti, et al. Using shape effects to target antibody-coated nanoparticles to lung and brain endothelium, Proc. Natl. Acad. Sci., 2013, 110(26), 10753–10758 CrossRef CAS PubMed.
- K. S. Chu, W. Hasan, S. Rawal, M. D. Walsh, E. M. Enlow and J. C. Luft, et al. Plasma, tumor and tissue pharmacokinetics of Docetaxel delivered via nanoparticles of different sizes and shapes in mice bearing SKOV-3 human ovarian carcinoma xenograft, Nanomed.: Nanotechnol. Biol. Med., 2013, 9(5), 686–693 CrossRef CAS PubMed.
- T. Shimada, M. Ueda, H. Jinno, N. Chiba, M. Wada and J. Watanabe, et al. Development of targeted therapy with paclitaxel incorporated into EGF-conjugated nanoparticles, Anticancer Res., 2009, 29(4), 1009–1014 CAS.
- N. Chiba, M. Ueda, T. Shimada, H. Jinno, J. Watanabe and K. Ishihara, et al. Novel immunosuppressant agents targeting activated lymphocytes by biocompatible MPC polymer conjugated with interleukin-2, Eur. Surg. Res., 2007, 39(2), 103–110 CrossRef CAS PubMed.
- S. Patil, A. Sandberg, E. Heckert, W. Self and S. Seal, Protein adsorption and cellular uptake of cerium oxide nanoparticles as a function of zeta potential, Biomaterials, 2007, 28(31), 4600–4607 CrossRef CAS PubMed.
- L. K. Limbach, Y. Li, R. N. Grass, T. J. Brunner, M. A. Hintermann and M. Muller, et al. Oxide nanoparticle uptake in human lung fibroblasts: effects of particle size, agglomeration, and diffusion at low concentrations, Environ. Sci. Technol., 2005, 39(23), 9370–9376 CrossRef CAS PubMed.
- S. Honary and F. Zahir, Effect of zeta potential on the properties of nano-drug delivery systems-a review (Part 1), Trop. J. Pharm. Res., 2013, 12(2), 255–264 Search PubMed.
- C. He, Y. Hu, L. Yin, C. Tang and C. Yin, Effects of particle size and surface charge on cellular uptake and biodistribution of polymeric nanoparticles, Biomaterials, 2010, 31(13), 3657–3666 CrossRef CAS PubMed.
- S. Honary and F. Zahir, Effect of zeta potential on the properties of nano-drug delivery systems-a review (Part 2), Trop. J. Pharm. Res., 2013, 12(2), 265–273 Search PubMed.
- U. Kedar, P. Phutane, S. Shidhaye and V. Kadam, Advances in polymeric micelles for drug delivery and tumor targeting, Nanomed.: Nanotechnol. Biol. Med., 2010, 6(6), 714–729 CrossRef CAS PubMed.
- K. K. Sawant and S. S. Dodiya, Recent advances and patents on solid lipid nanoparticles, Recent Pat. Drug Delivery Formulation, 2008, 2(2), 120–135 CrossRef CAS PubMed.
- A. Prokop, E. Kozlov, G. Carlesso and J. M. Davidson. Hydrogel-based colloidal polymeric system for protein and drug delivery: physical and chemical characterization, permeability control and applications. Filled Elastomers Drug Delivery Systems: Springer; 2002. pp. 119–73 Search PubMed.
- M. Beck-Broichsitter, C. Ruppert, T. Schmehl, A. Guenther, T. Betz and U. Bakowsky, et al. Biophysical investigation of pulmonary surfactant surface properties upon contact with polymeric nanoparticles in vitro, Nanomed.: Nanotechnol. Biol. Med., 2011, 7(3), 341–350 CrossRef CAS PubMed.
- J. O. Eloy, R. Petrilli, D. L. Chesca, F. P. Saggioro, R. J. Lee and J. M. Marchetti, et al. Anti-HER2 immunoliposomes for co-delivery of paclitaxel and rapamycin for breast cancer therapy, Eur. J. Pharm. Biopharm., 2017, 115, 159–167 CrossRef CAS PubMed.
- F. Alambin, P. M. Fath, B. E. Hosseinzadeh, A. H. Zarmi, R. Sahraeian and F. Yazdian, Leukemia stem cells, direct targeting of CD123 based on the nano-smart polymer PMBN, RSC Adv., 2016, 6(98), 96138–96146 RSC.
- Y. Fujimoto, T. Y. Morita, A. Ohashi, H. Haeno, Y. Hakozaki and M. Fujii, Combination treatment with a PI3K/Akt/mTOR pathway inhibitor overcomes resistance to anti-HER2 therapy in PIK3CA-mutant HER2-positive breast cancer cells, Sci. Rep., 2020, 10(1), 21762 CrossRef CAS PubMed.
- S. Pernas and S. M. Tolaney, HER2-positive breast cancer: new therapeutic frontiers and overcoming resistance, Ther. Adv. Med. Oncol., 2019, 11, 1758835919833519 CrossRef CAS PubMed.
- M. Gharbavi, A. Sharafi, P. Motamed Fath, S. Oruji, H. Pakzad and H. Kheiri Manjili, Formulation and Biocompatibility of Microemulsion-Based PMBN as an Efficient System for Paclitaxel Delivery, J. Appl. Biotechnol. Rep., 2021, 8(1), 51–62 CAS.
|
This journal is © The Royal Society of Chemistry 2022 |
Click here to see how this site uses Cookies. View our privacy policy here.