DOI:
10.1039/D1QO01175H
(Research Article)
Org. Chem. Front., 2022,
9, 12-18
Electrochemical oxidative cyclization of alkenes, boronic acids, and dichalcogenides to access chalcogenated boronic esters and 1,3-diols†
Received
7th August 2021
, Accepted 24th September 2021
First published on 28th September 2021
Abstract
A sustainable, environmentally benign electrochemical oxidative three-component cyclization of allylic alcohols, boronic acids, and dichalcogenides under metal-free and oxidant-free conditions has been developed, which provides an efficient approach for the formation of C–Se/S and C–O bonds together. A series of chalcogenated boronic esters were afforded with a broad substrate scope through a clean electrochemical system. The resulting chalcogenated cyclic boronic esters were easily converted to chalcogenated 1,3-diols in good yields under mild conditions.
Organochalcogen compounds are receiving increasing attention in chemical biology, pharmaceuticals, advanced functional molecules, and synthetic chemistry.1–4 Considering the easy accessibility, bench-stability, and operability of dichalcogenides as chalcogen reagents, utilizing them to introduce selenium and sulfur atoms into organic molecules proved to be an ideal approach. Due to the universality and convertibility of olefins, the difunctionalization of alkenes via an electrophilic or radical approach has become a practical and powerful tool for the construction of organochalcogen compounds.5,6 In general, conventional oxidants are necessary to oxidize dichalcogenides to produce free chalcogen radicals or electrophilic sulfonium/seleniranium ions (Scheme 1a).6 However, excessive oxidants will lead to lots of harmful wastes. As a sustainable and environment-friendly synthesis method, electrosynthesis follows the principles of green chemistry and is an effective alternative to traditional oxidants.7,8 Over the past decade, continuous endeavors have been made to develop efficient electrochemical methodologies to produce organochalcogen derivatives from alkenes.9,10 Recently, relevant achievements have been reported by Lei,9a,b Pan,9c,d Kim,9e and Xu.9f For example, Lei described the electrochemical oxidative aminoselenation and oxyselenation of styrenes without any acids or oxidants as additives in 2019.9a The electrochemical tandem cyclization of olefinic carbonyls with diselenides was proposed by the Lei group later. However, the preparation of chalcogenated cyclic boronic esters and 1,3-diols via electrosynthesis has not been studied. Therefore, it is urgent and appealing to develop an efficient strategy to achieve the diverse synthesis.
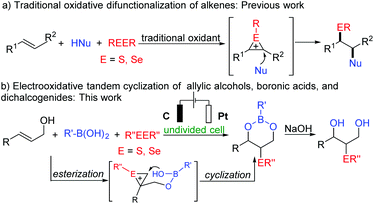 |
| Scheme 1 Electrochemical chalcogen (S/Se)-functionalization of alkenes. | |
The past two decades have witnessed the prosperous development of organoboron chemistry.11 Boron-containing compounds are widely used in many fields. Versatile boron complexes have the advantages of low cytotoxicity, positive bio-active functions, and air stability.12 High boron polymers can be used in the pharmaceutical polymer and electronic manufacturing industries.13 In addition, boronic acid and boron esters play an important role in synthesis and catalysis.14 Therefore, it is very attractive to develop effective methods to construct organoboron compounds. As part of our research interests,15 herein, we report our new discovery that chalcogenated cyclic boronic esters are easily accessed via electrochemical three-component tandem cyclization of allylic alcohols, boronic acids, and dichalcogenides under metal- and oxidant-free conditions (Scheme 1b). Chalcogenated 1,3-diols could also be obtained through the hydrolysis of the formed chalcogenated boronic esters.
Towards this aim, the electrochemical tandem cyclization reaction of cinnamyl alcohols, phenylboronic acids and diphenyl diselenides was selected as the model reaction (Table 1). Initially, with KBr as the electrolyte and CH3CN/H2O (v/v = 4/1) as the solvent, the desired cyclic boronic ester 4a was obtained in 60% yield under a constant current of 5 mA (entry 1). The effect of different additives on electrochemical cyclization reactions was investigated. We found that adding AcOH or TFE would reduce the formation of 4a (entries 2 and 3). In contrast, the addition of HFIP and TFA promoted the transformation, and TFA was proved to be more suitable for this reaction (64%–82% yields, entries 4 and 5). HFIP provides mildly acidic conditions for the cyclization. Subsequently, various electrolytes were tested under electrochemical conditions in the presence of TFA. The experimental results showed that a series of supporting electrolytes such as KI, NH4I, nBu4NBr, nBu4NBF4, and Et4NPF6 have lower efficiency than KBr (0–80% yields, entries 6–10). Moreover, only a trace amount of the target product was detected when NH4I or Et4NPF6 was used as the electrolyte. In order to further optimize the yield of the cyclic boronic ester, the influences of the solvent and current on the reaction were researched. When a mixed system THF/H2O (v
:
v = 4
:
1) was selected as the reaction solvent, we obtained the boronic ester product in 80% yield (entry 11). Substituting DMF/H2O or CH3CN for CH3CN/H2O led to a decrease in the production of 4a (entries 12 and 13). It was concluded that the solvent has a significant impact on the reaction. Furthermore, the product 4a was reduced sharply when the constant current was decreased from 5 mA to 3 mA (30% yield, entry 14). However, 81% yield of 4a was obtained at room temperature when the current was increased to 10 mA (entry 15). Thus, the cyclic boronic ester 4a could be efficiently synthesized under the condition of 5 mA constant current. Finally, no boronic ester product was observed in the absence of an electric current, which illustrates the necessity of electrochemical reactions (entry 16).
Table 1 Optimization of the reaction conditionsa

|
Entry |
Solvent |
Electrolyte |
Additive |
Yieldb (%) |
Reaction conditions: vitreous carbon plate anode, platinum plate cathode, constant current = 5 mA, 1a (0.3 mmol), 2a (0.3 mmol), 3a (0.3 mmol), electrolyte (1.2 mmol), additive (0.6 mmol), solvents (5.0 mL, v/v = 4 : 1), undivided cell, air, r.t., 12 h.
Isolated yield, n.d. = not detected.
Constant current = 3 mA.
Constant current = 10 mA.
No electric current. TFE = 2,2,2-trifluoroethanol, HFIP = 1,1,1,3,3,3-hexafluoro-2-propanol, TFA = trifluoroacetic acid. |
1 |
CH3CN/H2O |
KBr |
— |
60 |
2 |
CH3CN/H2O |
KBr |
AcOH |
48 |
3 |
CH3CN/H2O |
KBr |
TFE |
27 |
4 |
CH3CN/H2O |
KBr |
HFIP |
64 |
5 |
CH3CN/H2O |
KBr |
TFA |
82 |
6 |
CH3CN/H2O |
KI |
TFA |
28 |
7 |
CH3CN/H2O |
NH4I |
TFA |
Trace |
8 |
CH3CN/H2O |
n
Bu4NBr |
TFA |
68 |
9 |
CH3CN/H2O |
n
Bu4NBF4 |
TFA |
80 |
10 |
CH3CN/H2O |
Et4NPF6 |
TFA |
Trace |
11 |
THF/H2O |
KBr |
TFA |
80 |
12 |
DMF/H2O |
KBr |
TFA |
Trace |
13 |
CH3CN |
KBr |
TFA |
15 |
14c |
CH3CN/H2O |
KBr |
TFA |
30 |
15d |
CH3CN/H2O |
KBr |
TFA |
81 |
16e |
CH3CN/H2O |
KBr |
TFA |
n.d. |
With the optimized reaction conditions for generating the boronic esters established (Table 1, entry 5), the scopes and limitations of various substrates were explored (Scheme 2). First of all, we evaluated the substrate scope in regard to boronic acids. Not only phenylboronic acids with single substituents of electron-donating or electron-withdrawing groups at the para- or ortho-position, including –Me, –Br, and –Ome, all converted to boronic esters in moderate to good yields (4a–4d, 68%–82%), but also double substituent phenylboronic acids could react smoothly, delivering oxyselenation products with 52%–78% yields (4e–4g). To our satisfaction, boronic acids with heteroaromatic rings were well tolerated too. For example, the target product 4h could be synthesized in 65% yield under electrochemical conditions from 1-naphthylboronic acid. Moreover, phenanthreneboronic acid and 3-(9H-carbazol-9-yl) phenylboronic acid were reliable candidates, which reacted successfully and 4i and 4j were produced in good yields. It is noteworthy that the generation of boronic esters was not limited to arylboronic acids. Boronic acids bearing alkyl groups, such as n-propyl and cyclopropyl, were also transformed to the corresponding boronic esters (4k and4l, 65% and 72%, respectively).
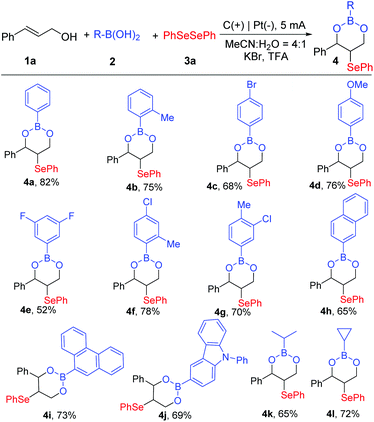 |
| Scheme 2 Substrate scope of boronic acids. Reaction conditions: 1a (0.3 mmol), 2 (0.3 mmol), 3a (0.3 mmol), KBr (1.2 mmol), TFA (0.6 mmol), MeCN/H2O = 4 mL/1 mL, 5 mA, r.t., 12 h. Isolated yield. | |
Next, we tested the reactivity of miscellaneous selenium compounds and allyl alcohols, as shown in Scheme 3. Ortho-substituted diphenyl diselenides with substituents such as –F, –Me, –Br, and –Cl were tolerated to provide the boronic ester products (5a–5d, 61%–80%). We could access the corresponding tandem oxyselenation derivatives 5e–5g in satisfactory yields from para-substituted diphenyl diselenides with electron-donating (–Me and –OMe) or electron-withdrawing (–Cl) groups on the benzene ring. When 1,2-bis(3-chlorophenyl)diselane and 1,2-bis(3,4-dimethylphenyl)diselane were chosen as the substrates, cyclic boronic esters were detected with yields of 69% and 80%, respectively. Interestingly, the transformations proceeded efficiently with dithiophene diselenide, dimethyl diselenide and dibenzyl diselenide, affording the target products in 54%–66% yields (5j–5l). In addition, our electrochemical esterification conditions were successfully applied to alkyl acrylols, which resulted in moderate to good yields of the corresponding boronic esters (5m–5p, 60%–78%). Cl- and MeO-substituted-aryls of cinnamyl alcohols proved to be efficient substrates, giving the desired products 5q and 5r in acceptable yields.
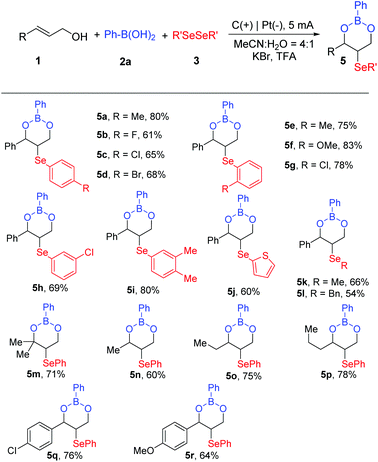 |
| Scheme 3 Substrate scope of alkenes and diselenides. Reaction conditions: 1 (0.3 mmol), 2a (0.3 mmol), 3 (0.3 mmol), KBr (1.2 mmol), TFA (0.6 mmol), MeCN/H2O = 4 mL/1 mL, 5 mA, r.t., 12 h. Isolated yield. | |
Disulfides were also successfully applied to this electrochemical cascade esterification reaction by using KBr as the electrolyte and AcOH as an additive under 10 mA constant current (Scheme 4). Efficacy studies were conducted to reveal the suitability of different boronic acids, allyl alcohols, and disulfides. On the one hand, these results indicated the good tolerance of boronic acids to construct cyclic sulfide boronic esters. When electron-donating groups, such as –Me and –OMe, were attached to the benzene ring, many target products could be afforded (6b, 6c, 6g–6i). Relatively less boronic ester products were obtained when electron-withdrawing groups like –F, –Cl, and –Br were introduced into the benzene ring (6d–6f, 6j). Double substituent phenylboronic acids could also give the products 6k–6n (60%–83%). What is noteworthy is that arylheterocyclic and alkyl boric acids were also suitable to react with allylic alcohols and disulfides, providing access to the desired products with satisfactory yields (6o–6r, 66%–75%).
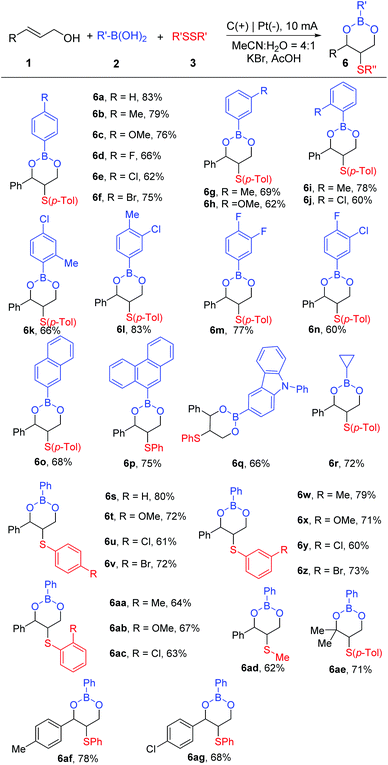 |
| Scheme 4 Substrate scope for electrochemical oxysulfenylation of alkenes. Reaction conditions: 1 (0.3 mmol), 2 (0.3 mmol), 3 (0.3 mmol), KBr (1.2 mmol), AcOH (0.6 mmol), MeCN/H2O = 4 mL/1 mL, 10 mA, r.t., 12 h. Isolated yield. | |
We tested the substrate scope of disulfides too. Various disulfides could efficiently convert into the target products 6s–6ac with yields of 60%–80%. In the same way, dimethyl disulfide could undergo the electrochemical reaction, giving the corresponding esterification product 6ad in a satisfactory yield. Then, this protocol was expanded to 3-methyl-2-buten-1-ol and the target product 6ae was produced in 71% yield. Finally, (E)-3-(p-tolyl)prop-2-en-1-ol and (E)-3-(4-chlorophenyl)prop-2-en-1-ol could also participate in the transformation and provide the cyclization products 6af and 6ag.
The resulting cyclic boronic esters were easily converted to chalcogenated 1,3-diols under mild conditions. For example, the cyclic boronic ester product 6a could be hydrolyzed to sulfide 1,3-diol 7 in 88% yield in the presence of a base at 120 °C (Scheme 5, eqn (1)). To our delight, the formed sulfide 1,3-diol 7 was further oxidized by H2O2 and converted to sulfone-containing 1,3-diol 8 in 67% yield (Scheme 5, eqn (2)).
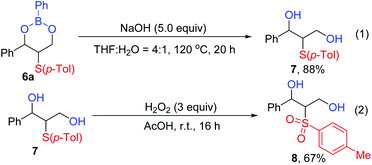 |
| Scheme 5 Further applications. | |
To gain more insights into the mechanism of this electrochemical three-component tandem cyclization, several radical scavengers were added to the model reaction (Scheme 6). It was found that the yield of 3a decreased when 2 equiv. of radical scavengers TEMPO, BHT, and p-benzoquinone were added to the system, respectively.
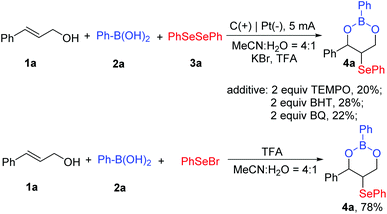 |
| Scheme 6 Control experiments. | |
Based on our experiments and the literature reports,9,10 two plausible reaction mechanisms for the electrochemical three-component cyclization have been presented in Scheme 7. In pathway I, Br+ was formed via electrochemical oxidation at the surface of the anode, which subsequently reacted with diselenide 3a to give active species PhSeBr. At the same time, cinnamyl alcohol reacted with phenylboronic acid in the presence of an acid to form intermediate I, which combined with active PhSeBr to produce intermediate II. Next, the target three-component cyclization product 4a was obtained via an intramolecular cyclization process. To give more support to the mechanism, we performed the cyclization of allylic alcohols, boronic acids, and PhSeBr without electricity, and as we expected, the desired product 4a was obtained in 78% yield (Scheme 6). In pathway II, diphenyl diselenide (3a) was oxidized to produce a cationic radical intermediate at the anode, which was quickly converted to a phenylselenium radical and the phenyl selenium cation PhSe+. The phenylselenium radical was captured by the in situ formed intermediate I to provide the carbon-centered radical III. Radical III underwent anodic oxidation to afford cation IV. Finally, intramolecular cyclization led to the formation of the desired product 4a.
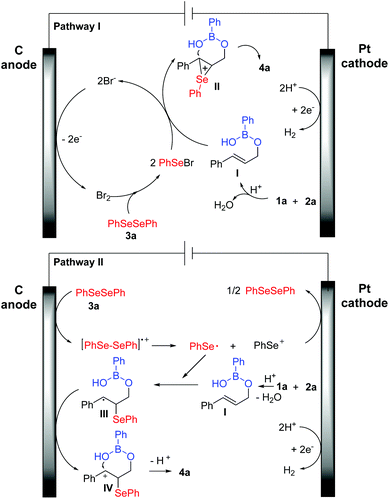 |
| Scheme 7 Proposed mechanism pathway. | |
Conclusions
In conclusion, we have disclosed efficient and environmentally friendly electrochemical oxidative oxyselenation and oxysulfenylation of allylic alcohols. Various chalcogenated boronic esters were directly accessed via electrochemical three-component tandem cyclization under undivided electrolytic conditions. In addition, the formed chalcogenated cyclic boronic esters were easily derived, especially converted to chalcogenated 1,3-diols under basic conditions. Further development of electrochemical oxidative reactions is currently underway in our laboratory.
Conflicts of interest
There are no conflicts to declare.
Acknowledgements
This work was supported by the National Natural Science Foundation of China (22001045), the Outstanding Youth Project of Guangdong Natural Science Foundation (2020B1515020026), the Innovation and Strong School Project of Guangdong Pharmaceutical University (2019KQNCX061, 2021ZDZX2028), the Guangdong Cosmetics Engineering & Technology Research Center, the Provincial Experimental Teaching Demonstration Center of Chemistry & Chemical Engineering, and Special Funds of Key Disciplines Construction from Guangdong and Zhongshan Cooperating.
Notes and references
-
(a)
Organoselenium Compounds in Biology and Medicine, ed. V. K. Jain and K. I. Priyadarsini, Royal Society of Chemistry, Cambridge, 2017 Search PubMed;
(b) L. Zhao, J. Li, Y. Li, J. Liu, T. Wirth and Z. Li, Selenium-containing naphthalimides as anticancer agents: Design, synthesis and bioactivity, Bioorg. Med. Chem., 2012, 20, 2558–2563 CrossRef CAS;
(c) Z. Wen, J. Xu, Z. Wang, H. Qi, Q. Xu, Z. Bai, Q. Zhang, K. Bao, Y. Wu and W. Zhang, 3-(3,4,5-Trimethoxyphenylselenyl)-1H-indoles and their selenoxides as combretastatin A-4 analogs: Microwave-assisted synthesis and biological evaluation, Eur. J. Med. Chem., 2015, 90, 184–194 CrossRef CAS.
-
(a) J. Min, P. Wang, S. Srinivasan, J. C. Nwachukwu, P. Guo, M. Huang, K. E. Carlson, J. A. Katzenellenbogen, K. W. Nettles and H. B. Zhou, Thiophene-Core Estrogen Receptor Ligands Having Superagonist Activity, J. Med. Chem., 2013, 56, 3346–3366 CrossRef CAS PubMed;
(b) S. Kumar, N. Sharma, I. K. Maurya, A. K. K. Bhasin, N. Wangoo, P. Brandão, V. Félix, K. K. Bhasin and R. K. Sharma, Facile synthesis, structural evaluation, antimicrobial activity and synergistic effects of novel imidazo[1,2-a]pyridine based organoselenium compounds, Eur. J. Med. Chem., 2016, 123, 916–924 CrossRef CAS;
(c) P. K. Sahu, G. Kim, J. Yu, J. Y. Ahn, J. Song, Y. Choi, X. Jin, J. H. Kim, S. K. Lee, S. Park and L. S. Jeong, Stereoselective Synthesis of 4′-Selenonucleosides via Seleno-Michael Reaction as Potent Antiviral Agents, Org. Lett., 2014, 16, 5796–5799 CrossRef CAS;
(d) Q. Jin, Z. Fu, L. Guan and H. Jiang, Syntheses of Benzo[d]Thiazol-2(3H)-One Derivatives and Their Antidepressant and Anticonvulsant Effects, Mar. Drugs, 2019, 17, 430–440 CrossRef CAS.
-
(a) D. S. Rampon, F. S. Rodembusch, J. M. F. M. Schneider, I. H. Bechtold, P. F. B. Goncalves, A. Merlo and P. H. Schneider, Novel selenoesters fluorescent liquid crystalline exhibiting a rich phase polymorphism, J. Mater. Chem., 2010, 20, 715–722 RSC;
(b) I. Samb, J. Bell;, P. Y. Toullec, V. Michelet and I. Leray, Fluorescent Phosphane Selenide As Efficient Mercury Chemodosimeter, Org. Lett., 2011, 13, 1182–1185 CrossRef CAS PubMed;
(c) D. Tanini, B. Lupori, G. Malevolti, M. Ambrosi, P. L. Nostro and A. Capperucci, Direct biocatalysed synthesis of first sulfur-, selenium- and tellurium- containing l-ascorbyl hybrid derivatives with radical trapping and GPx-like properties, Chem. Commun., 2019, 55, 5705–5708 RSC.
-
(a) D. M. Freudendahl, S. Santoro, S. A. Shahzad, C. Santi and T. Wirth, Green Chemistry with Selenium Reagents: Development of Efficient Catalytic Reactions, Angew. Chem., Int. Ed., 2009, 48, 8409–8411 CrossRef CAS PubMed;
(b) S. Ortgies and A. Breder, Oxidative Alkene Functionalizations via Selenium-π-Acid Catalysis, ACS Catal., 2017, 7, 5828–5840 CrossRef CAS;
(c) R. Guo, L. Liao and X. Zhao, Electrophilic Selenium Catalysis with Electrophilic N-F Reagents as the Oxidants, Molecules, 2017, 22, 835–848 CrossRef.
-
(a) K. Sun, X. Wang, C. Li, H. Wang and L. Li, Recent advances in tandem selenocyclization and tellurocyclization with alkenes and alkynes, Org. Chem. Front., 2020, 7, 3100–3119 RSC;
(b) W. Guo, K. Tao, W. Tan, M. Zhao, L. Zheng and X. Fan, Recent Advances in Photocatalytic C-S/P-S Bond Formation via the Generation of Sulfur Centered Radicals
and Functionalization, Org. Chem. Front., 2019, 6, 2048–2066 RSC.
-
(a) G. Perin, P. Santoni, A. M. Barcellos, P. C. Nobre, R. G. Jacob, E. J. Lenardao and C. Santi, Selenomethoxylation of Alkenes Promoted by Oxone®, Eur. J. Org. Chem., 2018, 1224 CrossRef CAS;
(b) S. Kodama, T. Saeki, K. Mihara, S. Higashimae, S. I. Kawaguchi, M. Sonoda, A. Nomoto and A. Ogawa, A Benzoyl Peroxide/Diphenyl Diselenide Binary System for Functionalization of Alkynes Leading to Alkenyl and Alkynyl Selenides, J. Org. Chem., 2017, 82, 12477–12484 CrossRef CAS;
(c) K. Sun, X. Wang, F. Fu, C. Zhang, Y. Chen and L. Liu, Metal-free selenosulfonylation of alkynes: rapid access to β-(seleno)vinyl sulfones via a cationic-species-induced pathway, Green Chem., 2017, 19, 1490–1493 RSC;
(d) X. Wang, H. Li, M. Zhu and J. Yan, Convenient iodine-mediated aminoselenation of alkenes using benzotriazoles as nitrogen sources, RSC Adv., 2017, 7, 15709–15714 RSC;
(e) K. Sun, X. Wang, C. Zhang, S. Zhang, Y. Chen, H. Jiao and W. Du, Amidoselenation and Amidotelluration of Alkenes using Oxygen as Terminal Oxidant, Chem. – Asian J., 2017, 12, 713–717 CrossRef CAS;
(f) K. Sun, X. Wang, Y. Lv, G. Li, H. Jiao, C. Dai, Y. Li, C. Zhang and L. Liu, Peroxodisulfate-mediated selenoamination of alkenes yielding amidoselenide-containing sulfamides and azoles, Chem. Commun., 2016, 52, 8471–8474 RSC;
(g) A. A. Vieira, J. B. Azeredo, M. Godoi, C. Santi, E. N. da Silva and A. L. Braga, Catalytic Chalcogenylation under Greener Conditions: A Solvent-Free Sulfur- and Seleno-functionalization of Olefins via I2/DMSO Oxidant System, J. Org. Chem., 2015, 80, 2120–2127 CrossRef CAS PubMed;
(h) Y. Zheng, Y. He, G. Rong, X. Zhang, Y. Weng, K. Dong, X. Xu and J. Mao, NaI-Mediated Acetamidosulphenylation of Alkenes with Nitriles as the Nucleophiles: A Direct Access to Acetamidosulfides, Org. Lett., 2015, 17, 5444–5447 CrossRef CAS;
(i) H. Shi, C. Yu, M. Zhu and J. Yan, Novel and convenient acetoxyselenenylation of alkenes catalyzed by potassium iodide, J. Organomet. Chem., 2015, 776, 117–122 CrossRef CAS;
(j) L. Yu, B. Chen and X. Huang, Multicomponent reactions of allenes, diaryl diselenides, and nucleophiles in the presence of iodosobenzene diacetate: direct synthesis of 3-functionalized-2-arylselenyl substituted allyl derivatives, Tetrahedron Lett., 2007, 48, 925–927 CrossRef CAS;
(k) N. Taniguchi, Copper-Catalyzed 1,2-Hydroxysulfenylation of Alkene Using Disulfide via Cleavage of the S-S Bond, J. Org. Chem., 2006, 71, 7874–7876 CrossRef CAS PubMed.
-
(a) Y. Yuan and A. Lei, Is electrosynthesis always green and advantageous compared to traditional methods?, Nat. Commun., 2020, 11, 802–805 CrossRef CAS PubMed;
(b) Y. Yuan and A. Lei, Electrochemistry of Atomically Precise Metal Nanoclusters, Acc. Chem. Res., 2019, 52, 3309–3324 CrossRef CAS PubMed;
(c) J. C. Siu, N. Fu and S. Lin, Catalyzing Electrosynthesis: A Homogeneous Electrocatalytic Approach to Reaction Discovery, Acc. Chem. Res., 2020, 53, 547–560 CrossRef CAS PubMed;
(d) M. Yan, Y. Kawamata and P. S. Baran, Synthetic Organic Electrochemical Methods Since 2000: On the Verge of a Renaissance, Chem. Rev., 2017, 117, 13230–13319 CrossRef CAS PubMed;
(e) R. Francke and R. D. Little, Redox catalysis in organic electrosynthesis: basic principles and recent developments, Chem. Soc. Rev., 2014, 43, 2492–2521 RSC.
-
(a) J. Yoshida, K. Kataoka, R. Horcajada and A. Nagaki, Modern Strategies in Electroorganic Synthesis, Chem. Rev., 2008, 108, 2265–2299 CrossRef CAS;
(b) S. R. Waldvogel and B. Janza, Renaissance of Electrosynthetic Methods for the Construction of Complex Molecules, Angew. Chem., Int. Ed., 2014, 53, 7122–7123 CrossRef CAS;
(c) M. K. Kärkäs, Electrochemical strategies for C-H functionalization and C-N bond formation, Chem. Soc. Rev., 2018, 47, 5786–5865 RSC.
-
(a) L. Sun, Y. Yuan, M. Yao, H. Wang, D. Wang, M. Gao, Y. H. Chen and A. Lei, Electrochemical Aminoselenation and Oxyselenation of Styrenes with Hydrogen Evolution, Org. Lett., 2019, 21, 1297–1300 CrossRef CAS PubMed;
(b) Z. P. Guan, Y. K. Wang, H. M. Wang, Y. G. Huang, S. Y. Wang, H. D. Tang, H. Zhang and A. Lei, Electrochemical oxidative cyclization of olefinic carbonyls with diselenides, Green Chem., 2019, 21, 4976–4980 RSC;
(c) X. Y. Wang, Y. F. Zhong, Z. Y. Mo, S. H. Wu, Y. L. Xu, H. T. Tang and Y. M. Pan, Synthesis of Seleno Oxindoles via Electrochemical Cyclization of N-arylacrylamides with Diorganyl DiSelenides, Adv. Synth. Catal., 2021, 363, 208–214 CrossRef CAS;
(d) X. J. Meng, P. F. Zhong, Y. M. Wang, H. S. Wang, H. T. Tang and Y. M. Pan, Electrochemical Difunctionalization of Olefines: Access to Selenomethyl–Substituted Cyclic Ethers or Lactones, Adv. Synth. Catal., 2020, 362, 506–511 CrossRef CAS;
(e) Y. J. Kim and D. Y. Kim, Electrochemical Radical Selenylation/1,2-Carbon Migration and Dowd-Beckwith-Type Ring-Expansion Sequences of Alkenylcyclobutanols, Org. Lett., 2019, 21, 1021–1025 CrossRef CAS;
(f) W. Gao, B. Li, L. Zong, L. Yu, X. Li, Q. Li, X. Zhang, S. Zhang and K. Xu, Electrochemical Tandem Cyclization of Unsaturated Oximes with Diselenides: A General Approach to Seleno Isoxazolines Derivatives with Quaternary Carbon Center, Eur. J. Org. Chem., 2021, 2431–2435 CrossRef CAS.
-
(a) P. N. Makhal, A. Nandi and V. R. Kaki, Insights into the Recent Synthetic Advances of Organoselenium Compounds, ChemistrySelect, 2021, 6, 663–679 CrossRef CAS;
(b) G. M. Martins, A. G. Meirinho, N. Ahmed, A. L. Braga and S. R. Mendes, Recent Advances in Electrochemical Chalcogen (S/Se)-Functionalization of Organic Molecules, ChemElectroChem, 2019, 6, 5928–5940 CrossRef CAS;
(c) G. M. Martins, G. C. Zimmer, S. R. Mendes and N. Ahmed, Electrifying green synthesis: Recent advances in electrochemical annulation reactions, Green Chem., 2020, 22, 4849–4870 RSC;
(d) S. Mallick, M. Baidya, K. Mahanty, D. Maiti and S. D. Sarkar, Electrochemical chalcogenation of β,γ-unsaturated amides and oximes to corresponding oxazolines and isoxazolines, Adv. Synth. Catal., 2020, 362, 1046–1052 CrossRef CAS.
-
(a) A. Stubelius, S. Lee and A. Almutairi, The Chemistry of Boronic Acids in Nanomaterials for Drug Delivery, Acc. Chem. Res., 2019, 52, 3108–3119 CrossRef CAS PubMed;
(b) N. D. J. Hiller, N. A. do Amaral e Silva, T. A. Tavares, R. X. Faria, M. N. Eberlin and D. de Luna Martins, Arylboronic Acids and their Myriad of Applications Beyond Organic Synthesis, Eur. J. Org. Chem., 2020, 4841–4877 CrossRef CAS;
(c) Q. Xia, J. Zhang, X. Chen, C. Cheng, D. Chu, X. Tang, H. Li and Y. Cui, Synthesis, structure and property of boron-based metal-organic materials, Coord. Chem. Rev., 2021, 435, 213783 CrossRef CAS;
(d) A. J. J. Lennox and G. C. Lloyd-Jones, Selection of boron reagents for Suzuki-Miyaura coupling, Chem. Soc. Rev., 2014, 43, 412–443 RSC.
-
(a)
D. G. Hall, Boronic Acids, Wiley-VCH Verlag Gmbh, Weinheim, 2005, pp. 1–4 CrossRef;
(b) M. M. Alcaide, F. M. F. Santos, V. F. Pais, J. I. Carvalho, D. Collado, E. Perez-Inestrosa, J. F. Arteaga, F. Bosca, P. M. P. Gois and U. Pischel, Electronic and Functional Scope of Boronic Acid Derived Salicylidenehydrazone (BASHY) Complexes as Fluorescent Dyes, J. Org. Chem., 2017, 82, 7151–7158 CrossRef CAS;
(c) J. Chen, R. A. Lalancette and F. Jäkle, Synthesis and Lewis acid properties of a ferrocene-based planar-chiral borenium cation, Chem. Commun., 2013, 49, 4893–4895 RSC.
-
(a) F. Y. Qiu, M. Zhang, R. Ji, F. S. Du and Z. C. Li, Oxidation-Responsive Polymer-Drug Conjugates with a Phenylboronic Ester Linker, Macromol. Rapid Commun., 2015, 36, 2012–2018 CrossRef CAS PubMed;
(b) E. A. Garcia, D. Pessoab and M. Herrera-Alonso, Oxidative instability of boronic acid-installed polycarbonate nanoparticles, Soft Matter, 2020, 16, 2473–2479 RSC;
(c) W. Xiao, N. Suby, K. Xiao, T. Y. Lin, N. A. Awwad, K. S. Lam and Y. Li, Extremely long tumor retention, multi-responsive boronate crosslinked micelles with superior therapeutic efficacy for ovarian cancer, J. Controlled Release, 2017, 264, 169–179 CrossRef CAS PubMed.
-
(a) D. G. Hall, Boronic acid Catalysis, Chem. Soc. Rev., 2019, 48, 3475–3496 RSC;
(b) J. Plescia and N. Moitessier, Design and discovery of boronic acid drugs, Eur. J. Med. Chem., 2020, 195, 112270–112293 CrossRef CAS;
(c) J. A. L. da Silva, Borate esters of polyols: Occurrence, applications and implications, Inorg. Chim. Acta, 2021, 520, 120307 CrossRef CAS.
-
(a) J. Li, X. Liu, J. Deng, Y. Huang, Z. Pan, Y. Yu and H. Cao, Electrochemical diselenylation of indolizines via intermolecular C–Se formation with 2-methylpyridines, a-bromoketones and diselenides, Chem. Commun., 2020, 56, 735–738 RSC;
(b) J. Zhou, W. Li, H. Zheng, Y. Pei, X. Liu and H. Cao, Visible Light-Induced Cascade Cyclization of 3–Aminoindazoles, Ynals, and Chalcogens: Access to Chalcogen-Containing Pyrimido[1,2–b]–indazoles, Org. Lett., 2021, 23, 2754–2759 CrossRef;
(c) X. Liu, Y. Wang, D. Song, Y. Wang and H. Cao, Electrochemical regioselective selenylation/oxidation of N-alkylisoquinolinium salts via double C(sp2)–H bond functionalization, Chem. Commun., 2020, 56, 15325–15328 RSC.
Footnote |
† Electronic supplementary information (ESI) available. See DOI: 10.1039/d1qo01175h |
|
This journal is © the Partner Organisations 2022 |