DOI:
10.1039/D1QI01169C
(Research Article)
Inorg. Chem. Front., 2022,
9, 83-89
Electronic configuration modulation of tin dioxide by phosphorus dopant for pathway change in electrocatalytic water oxidation†
Received
15th September 2021
, Accepted 3rd November 2021
First published on 3rd November 2021
Abstract
The oxygen evolution process is a crucial part determining the total reaction kinetics of electrocatalytic overall water splitting for clean hydrogen production. However, for some semiconductor materials, e.g. tin dioxide (SnO2) and tungsten oxide (WO3), the water oxidation process generally proceeds following the two-electron pathway with rather high driving potential for the generation of hydrogen peroxide (H2O2 or HO2−). In this work, efficient electronic configuration modulation of SnO2 nanoparticles was realized by non-metallic phosphorus (P) doping, giving rise to the considerable transformation into a four-electron transfer-governed water oxidation reaction with the electrocatalytic activity even comparable to the benchmark RuO2 nanoparticles. These results validate the great potential in remarkably tuning the electrocatalytic performance of transition metal compound electrocatalysts with non-metallic element dopants.
1. Introduction
With the ever-increasing energy crisis as well as the environmental issues, the development of alternative sustainable and clean energy resource candidates to conventional fossil fuels is urgently desired. Due to the clean combustion and relatively high energy density, hydrogen (H2) fuel shows great potential in the future of renewable energy.1,2 Among current industrial approaches for H2 generation, electrochemical overall water splitting (2H2O → 2H2 + O2) using a reproducible electricity resource is highly appreciated; however, it is held back by the kinetically sluggish water oxidation part.3,4 As a matter of fact, water oxidation is categorized into three types.5,6 (i) Four-electron-transfer water oxidation, i.e. oxygen evolution reaction (simplified as OER), is described as 2H2O → O2 + 4H+ + 4e with a theoretical potential (E°) of 1.23 V. (ii) Two-electron-transfer water oxidation for H2O2 generation proceeds as 2H2O → H2O2 + 2H+ + 2e with an E° value of 1.76 V. (iii) One-electron-transfer pathway to form the hydroxyl radical (˙OH) follows the equation: H2O → ˙OH + H+ + e (E° = 2.38 V). To realize the energetic overall water splitting, advanced electrochemical materials catalyzing the OER efficiently are imminently anticipated on account of the lowest applied potential driving water oxidation. Currently, the state-of-the-art electrocatalyst benchmarks are usually noble metal-based materials such as IrO2 and RuO2.7 Nevertheless, they suffer from severe scarcity as well as high cost. It is, therefore, significant to develop high-performance OER electrocatalysts based on cheap and Earth-abundant resources for large-scale H2 production.
Tin dioxide (SnO2), a typical semiconductor material of transition metal oxide compounds, has been widely used in sensors, electronic devices, Li-ion batteries, photo(electro)chemistry, etc.8–12 In very recent years, materials based on SnO2 have also been demonstrated as efficient electrocatalysts for CO2 reduction.13,14 When it comes to electrocatalytic water oxidation, SnO2 materials generally induce the two-electron-transfer pathway although it is a promising approach for the synthesis of H2O2, a prominent chemical product.5,15,16 On the other hand, composition optimization is regarded as a rational route modulating the electronic configuration and thus significantly regulating the electrocatalytic performance. Among the various composition tuning techniques, element doping through atomic substitution in host lattices by guest atoms/ions is one of the most effective methods.17,18 To modify the function of SnO2 materials in catalyzing water oxidation, numerous efforts have been made in previous studies. For instance, Abbou et al. proposed that Sb and/or Ta dopants could markedly enhance the activity of SnO2 in water oxidation.19 Moreover, Ullah and co-workers demonstrated an evolution of water oxidation from a typical two-electron-transfer pathway to the conventional OER through W doping on SnO2 nanoparticles.20 These metal element dopants have verified the considerable influences on SnO2 materials in tuning their water oxidation performance. On the other hand, doping with non-metallic elements has also been reported to be effective in modulating the physicochemical properties of transition metal oxides.21–23 Early literature has predicted that P doping could dramatically regulate the electronic configuration of SnO2 and improve the electroconductivity theoretically,24 which might be favorable for the performance optimization in catalyzing the water oxidation reaction. However, few related experimental works have verified this assumption.
Herein, SnO2 nanoparticles modified with non-metallic P dopant were prepared. As the merit of P doping, the electrocatalytic water oxidation proceeded following a four-electron transfer-governed OER process rather than the typical two-electron pathway for the doped SnO2 product (denoted as P–SnO2) with the activity even comparable to the RuO2 benchmark. In addition, theoretical calculations also provided a piece of strong support for both the electronic structure modulation and reaction pathway change. The results may further verify the role of non-metallic dopants in the careful design of advanced electrocatalysts.
2. Experimental section
2.1. Synthesis of SnO2 nanoparticles
First, a CoSn(OH)6 precursor was synthesized by the co-precipitation of stoichiometric Co2+ and Sn4+ ions in the presence of OH− at room temperature according to a previous work.25 Then the precursor was calcined at 650 °C in an air environment for 1 h. After cooling down naturally, the as-calcined powder was dispersed in a concentrated nitric acid solution (2 M) and transferred into a Teflon-lined autoclave (40 cm3) and maintained at 180 °C for 2 h. A brown product was harvested via separating the hydrothermal precipitate and being washed with deionized water several times. Finally, after drying at 60 °C, SnO2 nanoparticles were obtained.
2.2. Synthesis of P–SnO2 nanoparticles
To realize the successful preparation of P–SnO2 nanoparticles, the as-obtained SnO2 counterparts were thermally treated by a phosphorus source at an elevated temperature. In a typical procedure, hydrated sodium hypophosphite (NaH2PO2·H2O) and the as-obtained SnO2 powder with a weight ratio of 1
:
10 were placed separately with the former positioned at the upstream side, and then heated to 350 °C and maintained for 2 h under an Ar flow. After cooling down naturally, a grey powder was obtained.
2.3. Synthesis of RuO2 nanoparticles
RuO2 nanoparticles were prepared following the previous report.26 Typically, 1 mmol of hydrated ruthenium chloride (RuCl3·xH2O) was dissolved in 20 mL of deionized water. Then the mixture was heated to 100 °C and 1 M NaOH solution (0.1 mL) was added dropwise. After continuous agitation for 1 h, the precipitate was collected by high-speed centrifugation, washed several times and dried under vacuum. RuO2 nanoparticles were attained by calcining the co-precipitate product at 200 °C for 3 h.
2.4. Material characterization
The crystal phases and composition information of the as-prepared samples were recorded using an X-ray diffractometer (XRD, λ = 1.54178 Å, D/max 2500VB+), energy dispersive X-ray spectroscope (EDX) and inductively coupled plasma optical emission spectrometer (ICP-OES, SPECTRO BLUE SOP), respectively. Morphological and structural data were investigated through a scanning electron microscope (SEM, FEI Helios Nanolab 600i) and a transmission electron microscope (TEM, JEOL 3100). The chemical bonding energy information was recorded using an X-ray photoelectron spectroscope (XPS, ESCALAB250Xi).
2.5. Electrochemical measurements
All the electrochemical measurements were conducted on a CH Instruments model 760E workstation coupled with a conventional three-electrode setup using a graphite rod as the counter electrode. Hg/HgO and Hg/Hg2SO4 electrodes acted as the reference in alkaline KOH (0.1 M) and acidic H2SO4 (1.0 M) electrolytes, respectively. A catalyst ink was prepared by dispersing the catalyst powder homogeneously in a mixture of 2-propanol, deionized water and Nafion solution (10 wt%) at a volume ratio of 25/75/1 under ultrasonic treatment. Then the ink was dripped onto the polished clean glassy carbon electrode and dried at 105 °C to ensure the catalyst loading as ∼0.20 mg cm−2. A rotation rate of 1600 rpm and a scan rate of 10 mV s−1 with 95% iR compensation were adopted for the polarization curve tests. All the measured potentials in the current work were calibrated to the reversible hydrogen electrode (RHE) scale according to the equation: E(RHE) = E(vs. Ref.) + Est. + 0.059 × pH, where E(vs. Ref.) represents the metric potential versus the reference electrode and Est. was 0.098 V and 0.658 V for Hg/HgO and Hg/Hg2SO4 electrodes, respectively. Electrochemical impedance spectroscopy (EIS) data were investigated at a potential of 1.75 V vs. RHE in the frequency range of 1000–0.1 Hz with an AC voltage amplitude of 5 mV. Rotating ring-disk electrode (RRDE) voltammograms setting the ring potential as 1.50 V vs. RHE were used to determine the electron transfer number (N) according to the equation: N = 4 × Id/(Id + Ir/Nc),27,28 where Id represents the disk current, Ir represents the ring current and Nc represents the current collection efficiency. The electrochemical surface area (ECSA) was estimated from the cyclic voltammetry (CV) curves at incremental rates of 10, 20, 30, 40 and 50 mV s−1.
2.6. Calculations
All calculations were performed using the Vienna Ab initio Simulation Package (VASP) based on the first principles calculations.29,30 For the exchange–correlation functional, the Perdew–Burke–Ernzerhof functional of generalized gradient approximation was adopted. In the calculation, a kinetic energy cutoff of 450 eV for the plane wave expansion and 5 × 6 × 1 grid of k points were set for the heterostructures in this work. The energy and force convergence criteria are 10−5 eV and 10−2 eV Å−1 in the relaxation, respectively. Given the periodic boundary conditions, a vacuum layer of 15 Å was used to avoid the image interaction.
3. Results and discussion
SnO2 nanoparticles were prepared by hydrothermal acid treatment of the calcined product of the CoSn(OH)6 precursor which was synthesized via the co-precipitation of stoichiometric Co2+ and Sn4+ ions in the presence of OH−, as depicted in Fig. 1. For the P–SnO2 counterparts, they were synthesized by treating SnO2 nanoparticles with NaH2PO2·H2O at an elevated temperature (e.g. 350 °C).
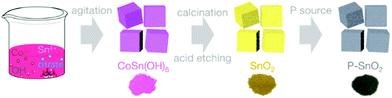 |
| Fig. 1 Scheme for the preparation of SnO2 and P–SnO2 nanoparticles. | |
To verify the phase composition, all the samples were examined by XRD. Fig. S1a† shows the XRD trace of the pink co-precipitation product, which matched well with the cubic CoSn(OH)6 simulation (space group: Pn
m, PDF file no. 13-0356). When the pink product was calcined at 650 °C, a mixture of cubic Co3O4 (space group: Fd
m, PDF file no. 42-1467) and tetragonal SnO2 (space group: P42/mnm, PDF file no. 41-1445) phases was obtained, as evidenced by the XRD pattern in Fig. S1b.† After sequential acid treatment, a brown product was obtained. Fig. 2a shows the XRD plots of both the samples before and after hypophosphite treatment, which were readily indexed as a tetragonal SnO2 phase (space group: P42/mnm, PDF file no. 41-1445). No other diffraction peaks of any possible impurity were detected, implying the high phase purity of the as-prepared samples. Moreover, magnified patterns at the 2theta range of 24°–36°, as can be seen in Fig. 2b, further showed an obvious shift to higher angles for both (110) and (101) peaks after the hypophosphite treatment. In addition, the EDX result, as shown in Fig. S2,† confirmed the existence of the P element in the P–SnO2 product. Moreover, the atomic ratio of P/Sn in the P–SnO2 product was determined to be 0.128 using ICP-OES. According to the diffraction data, lattice parameters of the samples were refined using the Appleman method based on the least-squares refinement.31,32 As plotted in Fig. 2c and d, the lattice parameters decreased from initial a = 4.749(2) Å and c = 3.191(4) Å for SnO2 to a = 4.695(3) Å and c = 3.167(1) Å for the P-containing counterpart, suggesting the presence of the P dopant in the SnO2 lattice.
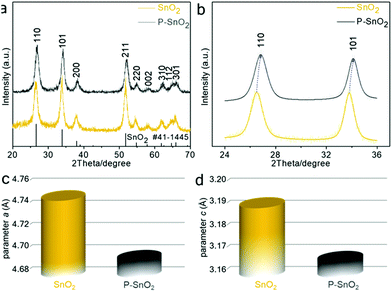 |
| Fig. 2 XRD results. (a) XRD patterns of SnO2 and P–SnO2 products. (b) Magnification of (a) in the 2theta range of 24–36°. Lattice parameters (c) a and (d) c for the tin oxide samples. | |
SEM and TEM observations were carried out on the as-prepared products to investigate the morphological and nanostructural details. As can be seen from Fig. S3a and b,† the co-precipitated product, i.e. CoSn(OH)6, consists of uniform nanocubes with sizes of 250–300 nm. After the harsh calcination and subsequent acid treatment, the cube-like morphology was almost maintained, respectively (Fig. S3c and d†). Fig. 3a displays the SEM image of the P–SnO2 sample. It was worth noting that even after the further thermal treatment in the presence of hypophosphite, the cubic nanoarchitecture was still well maintained. Fig. 3b shows the corresponding TEM result. Consistent with the SEM observation, regular nanocubes were also observed. It should be noted that the nanocubes were porous, as indicated by the area labelled by the brown arrows, which should be ascribed to the removal of the Co3O4 phase in the as-calcined product during the acid treatment under hydrothermal conditions. Fig. 3c shows the HRTEM image, which was recorded at the marked circle in Fig. 3b. Defined lattice fringes with an interplanar distance of 0.264 nm were observed, which were readily indexed to the (101) planes. The SAED pattern, as presented in the inset of Fig. 3c, further revealed the polycrystalline nature of the P–SnO2 product. The composition of the doped product was also investigated using a high-resolution scanning transmission electron microscope coupled with EDX mapping, as plotted in Fig. 3d. All of the Sn, O and P elements were homogeneously dispersed over an individual particle. It was notable that although P was in a relatively low content, the EDX signal was still sufficient to delineate a well-defined elemental map in accordance with the cubic shape, indicating a homogeneous doping result.
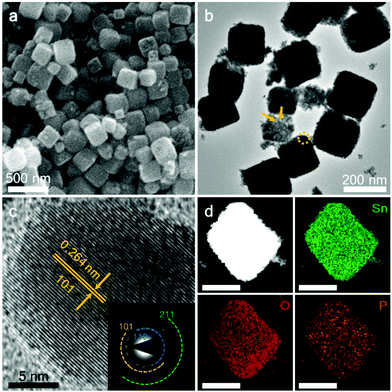 |
| Fig. 3 Morphological and nanostructural characterization on P–SnO2 nanoparticles. (a) SEM, (b) TEM and (c) HRTEM images and (d) elemental distribution map. The inset of (c) shows the corresponding SAED pattern and the scale bar in (d) is 200 nm. | |
To investigate the chemical states as well as the possible charge transfer originating from the doping behavior, XPS analyses were conducted on the as-prepared oxides. The survey spectrum, as shown in Fig. S4,† clearly indicated the co-existence of the Sn, O and P atoms in the P–SnO2 product. Fig. 4a shows the high-resolution patterns of Sn 3d orbits for both pristine and doped SnO2 samples. With respect to that of the undoped SnO2, a clear shift to a higher binding energy was detected for the signal of the doped product, which might be ascribed to the introduction of P atoms into the lattice on account of the more negative chemical state of P. A similar shift phenomenon was also demonstrated by P-doped hematite materials.33Fig. 4b displays the P 2p spectrum of the P–SnO2 product. The peak located at the lower binding energy (BE) corresponded to typical P–metal bonds, i.e. P–Sn herein (while the one at higher BE might be attributed to the possible oxidation of P species),34,35 further providing a piece of strong evidence for the doping behavior of P atoms in the SnO2 lattice.
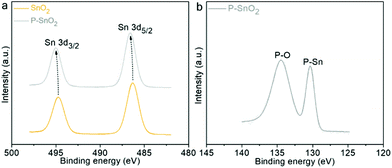 |
| Fig. 4 XPS spectra of tin oxide products: (a) Sn 3d and (b) P 2p. | |
To gain a deep insight into the doping effects of non-metallic P on transition metal oxides, the density of states (DOS) calculation analyses were performed, as shown in Fig. 5a. Electron distribution originating from the interaction among Sn, P and O atoms was presented around the Fermi level for the P–SnO2 phase compared to that of its pristine counterpart, implying the electroconductivity improvement after P doping. It was generally accepted that superior conductivity features would promote electron transfer during electrocatalysis. Thus, the P dopant might bring an advantageous effect on the SnO2 phase in electrochemical applications. Furthermore, as can be seen in Fig. 5b, the calculated Bader charge of +2.45e for Sn in P–SnO2, significantly larger than +1.98e for that in the pristine oxide phase, revealed the electron migration from Sn after the doping process, providing strong support for the XPS result in Fig. 4a.36 This electron transfer might be of advantage to the improvement of OH− affinity, favorable for the water oxidation process.
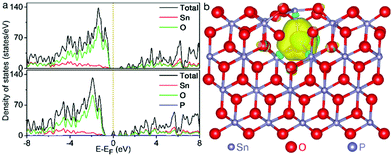 |
| Fig. 5 (a) DOS of the SnO2 and P–SnO2 phases. (b) Bader charge distribution of the P–SnO2 phase, wherein purple, red and blue balls stand for Sn, O and P atoms, respectively. | |
The electrocatalytic water oxidation performance of the as-prepared catalysts was evaluated in an alkaline system. Fig. 6a shows the linear sweep voltammetry (LSV) curves of the as-prepared catalysts. It could be seen that no obvious current response was detected for pristine SnO2 nanoparticles in the potential range of 1.2–1.8 V vs. RHE, in accordance with the reported literature.5,15 On the other hand, the P–SnO2 product achieved current densities of 10 and 63.1 mA cm−2 at 1.69 and 1.80 V vs. RHE, respectively, which were much superior to that of the CoSn(OH)6 precursor and even comparable to that of RuO2 nanoparticles prepared following the previous reports.26,37 In view of the onset potential location as well as the high current response at such a potential region, the water oxidation process catalyzed by P–SnO2 nanoparticles should be ascribed to the energetic OER. In contrast to the negligible current response by the pristine SnO2 product, this high activity indicated a considerable transformation from the two-electron-transfer water oxidation for H2O2 generation into a four-electron-involving pathway originating from P doping. Fig. 6b shows a Tafel slope of ∼39.7 mV dec−1 for P–SnO2 nanoparticles in comparison with 60.9 mV dec−1 for the CoSn(OH)6 precursor, suggesting much faster electrocatalytic reaction kinetics of the P–SnO2 product. Moreover, such a value, close to 2.3 × RT/2F (i.e. 30 mV dec−1; herein R, T and F were the gas constant, temperature and faradaic constant, respectively), might imply the formation of *OOH (* represents the catalytic sites) as the rate determining step.36–38 In addition, such favorable reaction kinetics could also be inferred by the EIS results, as displayed in Fig. 6c. On the basis of the EIS data, an equivalent circuit model shown in the inset of Fig. 6c was established. The intersections of the spectra with the real axis, i.e. Rs in the circuit with the value of ∼14.0 Ω, corresponded to the electrolyte resistance value. The charge-transfer resistance, represented by Rct, was estimated to be ∼72.5 Ω, much smaller than 154.8 Ω for the pristine SnO2, revealing significant electroconductivity improvement. Moreover, this resistance was also smaller compared to 106.3 Ω for the hydroxide precursor, indicating much more favorable reaction kinetics. According to the LSV curves, the turnover frequency (TOF) was also evaluated to be 0.125 s−1 for the P–SnO2 product, markedly surpassing 0.085 s−1 for CoSn(OH)6 nanoparticles at an overpotential of 0.57 V based on the assumption that all the catalytic sites were accessible. In addition, based on the double-layer capacitance (Cdl), as depicted in Fig. 6d and Fig. S5,† ECSA was estimated to be 2.27, 1.96 and 2.92 m2 g−1 for the hydroxide precursor, pristine and doped SnO2 products, respectively,39 suggesting that the doping treatment made the active sites of SnO2 sample much more accessible. Through normalizing the performance by ECSA, the intrinsic activity of the three samples could be attained, as plotted in Fig. 7a. It could be seen that in the OER-active region, especially 1.60–1.80 V vs. RHE, the intrinsic activity of the P–SnO2 product was still permanently superior to the CoSn(OH)6 precursor. For instance, the normalized current response was more than twice that of the hydroxide sample at 1.8 V vs. RHE. The electron transfer number, as plotted in Fig. S6† and the inset of Fig. 7a, was rather close to 4, confirming the doped SnO2 nanoparticle-catalyzed water oxidation process as the OER. The reaction process was also uncovered using theoretical simulations. Fig. 7b displays the free energy chart of a typical OER on the P–SnO2 product. It should be noted that the Gibbs free energy for the formation of *O was 0.77 eV, far lower than the typical formation energy of H2O2 (3.5 eV).40,41 This implied that a more favorable OER rather than H2O2 generation occurred on the P–SnO2 catalyst, providing strong theoretical support for the transition from a two-electron pathway to the OER during the water oxidation process induced by P doping. Moreover, a wide energy barrier existed for the formation of *OOH, which indicated Step III to be the rate-determining step, consistent with the Tafel slope analysis. In addition, the chronopotentiometry measurement at 10 mA cm−2 (Fig. S7†), the comparison between the LSV curves after the 1st and 50th CV scans (Fig. S8†) and XPS analysis after electrocatalysis (Fig. S9†) further confirmed that the P–SnO2 product showed satisfactory durability and stability during the continuous electrocatalytic process. In addition, the reaction pathway evolution of water oxidation was found in the acid electrolyte (Fig. S10†), further confirming the role of the P dopant in regulating the reaction pathway.
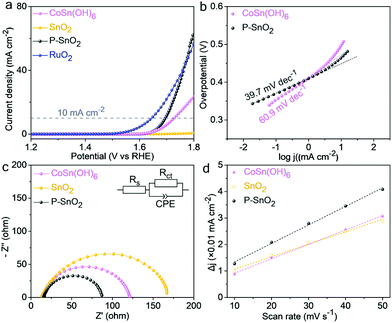 |
| Fig. 6 Electrocatalytic performance of tin oxide and CoSn(OH)6 samples in 0.1 M KOH solution. (a) LSV curves at a scan rate of 5 mV s−1, (b) Tafel slopes and (c) EIS plot. (d) The plots of differences between the anodic and cathodic current densities at 1.15 V vs. RHE as a function of scan rate. Based on the slopes of the plots, Cdl was estimated to be 27.3, 23.5 and 35.0 mF cm−2 for the hydroxide precursor, pristine and doped SnO2 products, respectively. | |
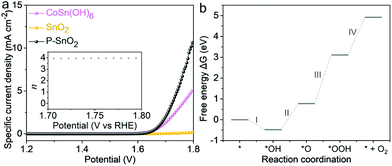 |
| Fig. 7 Electrocatalytic activity for water oxidation. (a) Normalized LSV curves by ECSA, and the inset shows the estimated electron transfer number (n) of the reaction catalyzed by the P–SnO2 sample. (b) Gibbs free energy change during the typical oxygen evolution process catalyzed by the P–SnO2 product. | |
4. Conclusions
In summary, P–SnO2 nanoparticles were prepared through the thermal treatment of a P-containing resource (NaH2PO2·H2O) on its pristine SnO2 counterpart. Theoretical calculations predicted both the electronic configuration modulation of the SnO2 phase and its pathway regulation in electrocatalytic water oxidation originating from the P dopant. As a result, P–SnO2 nanoparticles achieved a current density of 10 mA cm−2 at an overpotential of 0.46 V with a low Tafel slope of 39.7 mV dec−1 in 0.1 M KOH solution, realizing the reaction pathway change in electrocatalytic water oxidation and even comparable to the benchmark RuO2 nanoparticles. This pathway evolution uncovered the significant role of the P dopant in tuning the physicochemical properties of SnO2 materials, offering a promising route for developing advanced transition metal compound electrocatalysts through considerably modulating their electronic structures with non-metallic dopants.
Author contributions
H. W., X. L. and R. M. designed the experimental study. H. W. and X. X. carried out the materials preparation, characterization and electrocatalytic measurements. Y. L. completed the theoretical calculations. The manuscript was written through contributions of all authors. All authors have given approval to the final version of the manuscript.
Conflicts of interest
There are no conflicts to declare.
Acknowledgements
This work was financially supported by the National Natural Science Foundation of China (51874357, 51872333, and U20A20123), the Hunan Provincial Natural Science Foundation of China (2019JJ10006), and the China Postdoctoral Science Foundation (2020M682352). H. W. is thankful for the support from the Youth Talent Program of Zhengzhou University and Henan Provincial Key Technology R&D Program (212102210597). R. M. acknowledges support from JSPS KAKENNHI (18H03869).
Notes and references
- Z. W. Seh, J. Kibsgaard, C. F. Dickens, I. Chorkendorff, J. K. Nørskov and T. F. Jaramillo, Combining theory and experiment in electrocatalysis: Insights into materials design, Science, 2017, 355, 146 CrossRef PubMed.
- Y. Pan, Theoretical discovery of high capacity hydrogen storage metal tetrahydrides, Int. J. Hydrogen Energy, 2019, 44, 18153–18158 CrossRef CAS.
- L. Sun, Q. Luo, Z. Dai and F. Ma, Material libraries for electrocatalytic overall water splitting, Coord. Chem. Rev., 2021, 444, 214049 CrossRef CAS.
- P. Xiong, X. Zhang, H. Wan, S. Wang, Y. Zhao, J. Zhang, D. Zhou, W. Gao, R. Ma, T. Sasaki and G. Wang, Interface modulation of two-dimensional superlattices for efficient overall water splitting, Nano Lett., 2019, 19, 4518–4526 CrossRef CAS.
- S. Y. Park, H. Abroshan, X. Shi, H. S. Jung, S. Siahrostami and X. Zheng, CaSnO3: an electrocatalyst for two-electron water oxidation reaction to form H2O2, ACS Energy Lett., 2019, 4, 352–357 CrossRef CAS.
- S. C. Perry, D. Pangotra, L. Vieira, L. I. Csepei, V. Sieber, L. Wang, C. P. de León and F. C. Walsh, Electrochemical synthesis of hydrogen peroxide from water and oxygen, Nat. Rev. Chem., 2019, 3, 442–458 CrossRef CAS.
- A. Zagalskaya and V. Alexandrov, Role of defects in the interplay between adsorbate evolving and lattice oxygen mechanisms of the oxygen evolution reaction in RuO2 and IrO2, ACS Catal., 2020, 10, 3650–3657 CrossRef CAS.
- J. H. Kim, A. Mirzaei, H. W. Kim and S. S. Kim, Low power-consumption CO gas sensors based on Au-functionalized SnO2-ZnO core-shell nanowires, Sens. Actuators, B, 2018, 267, 597–607 CrossRef CAS.
- S. Gao, N. Wang, S. Li, D. Li, Z. Cui, G. Yue, J. Liu, X. Zhao, L. Jiang and Y. Zhao, A multi-wall Sn/SnO2@carbon hollow nanofiber anode material for high-rate and long-life lithium-ion batteries, Angew. Chem., Int. Ed., 2020, 59, 2465–2472 CrossRef CAS PubMed.
- L. Yang, J. Huang, L. Shi, L. Cao, W. Zhou, K. Chang, X. Meng, G. Liu, Y. Jie and J. Ye, Efficient hydrogen evolution over Sb doped SnO2 photocatalyst sensitized by Eosin Y under visible light irradiation, Nano Energy, 2017, 36, 331–340 CrossRef CAS.
- J. Xie, K. Huang, X. Yu, Z. Yang, K. Xiao, Y. Qiang, X. Zhu, L. Xu, P. Wang, C. Cui and D. Yang, Enhanced electronic properties of SnO2 via electron transfer from graphene quantum dots for efficient perovskite solar cells, ACS Nano, 2017, 11, 9176–9182 CrossRef CAS.
- K. Wan, D. Wang, F. Wang, H. Li, J. Xu, X. Wang and J. Yang, Hierarchical In2O3@SnO2 core-shell nanofiber for high efficiency formaldehyde detection, ACS Appl. Mater. Interfaces, 2019, 11, 45214–45225 CrossRef CAS.
- F. Li, L. Chen, G. P. Knowles, D. R. MacFarlane and J. Zhang, Hierarchical mesoporous SnO2 nanosheets on carbon cloth: a robust and flexible electrocatalyst for CO2 reduction with high efficiency and selectivity, Angew. Chem., Int. Ed., 2017, 56, 505–509 CrossRef CAS PubMed.
- S. Liu, J. Xiao, X. F. Lu, J. Wang, X. Wang and X. Lou, Efficient Electrochemical reduction of CO2 to HCOOH over sub-2 nm SnO2 quantum wires with exposed grain boundaries, Angew. Chem., Int. Ed., 2019, 58, 8499–8503 CrossRef CAS PubMed.
- X. Shi, S. Siahrostami, G. L. Li, Y. Zhang, P. Chakthranont, F. Studt, T. F. Jaramillo, X. Zheng and J. K. Nørskov, Understanding activity trends in electrochemical water oxidation to form hydrogen peroxide, Nat. Commun., 2017, 8, 701 CrossRef PubMed.
- V. Viswanathan, H. A. Hansen and J. K. Nørskov, Selective electrochemical generation of hydrogen peroxide from water oxidation, J. Phys. Chem. Lett., 2015, 6, 4224–4228 CrossRef CAS.
- H. Wan, X. Liu, H. Wang, R. Ma and T. Sasaki, Recent advances in developing high-performance nanostructured electrocatalysts based on 3d transition metal elements, Nanoscale Horiz., 2019, 4, 789–808 RSC.
- J. Linnemann, K. Kanokkanchana and K. Tschulik, Design strategies for electrocatalysts from an electrochemist's perspective, ACS Catal., 2021, 11, 5318–5346 CrossRef CAS.
- S. Abbou, R. Chattot, V. Martin, F. Claudel, L. Solà-Hernandez, C. Beauger and L. Dubau, Manipulating the corrosion resistance of SnO2 aerogels through doping for efficient and durable oxygen evolution reaction electrocatalysis in acidic media, ACS Catal., 2020, 10, 7283–7294 CrossRef CAS.
- I. Ullah, A. Munir, S. Muhammad, S. Ali, N. Khalid, M. Zubair, M. Sirajuddin, S. Z. Hussain, S. Ahmed, Y. Khan, I. Hussain and A. Haider, Influence of W-doping on the optical and electrical properties of SnO2 towards photocatalytic detoxification and electrocatalytic water splitting, J. Alloys Compd., 2020, 827, 154247 CrossRef CAS.
- J. E. N. Swallow, B. A. D. Williamson, T. J. Whittles, M. Birkett, T. J. Featherstone, N. Peng, A. Abbott, M. Farnworth, K. J. Cheetham, P. Warren, D. O. Scanlon, V. R. Dhanak and T. D. Veal, Self–compensation in transparent conducting F–doped SnO2, Adv. Funct. Mater., 2018, 28, 1701900 CrossRef.
- W. Li, L. He, X. Bai, L. Liu, M. Ikram, H. Lv, M. Ullah, M. Khan, K. Kan and K. Shi, Enhanced NO2 sensing performance of S-doped biomorphic SnO2 with increased active sites and charge transfer at room temperature, Inorg. Chem. Front., 2020, 7, 2031–2042 RSC.
- M. V. Dozzi, A. Candeo, G. Marra, C. D'Andrea, G. Valentini and E. Selli, Effects of photodeposited gold vs platinum nanoparticles on N, F-doped TiO2 photoactivity: a time-resolved photoluminescence investigation, J. Phys. Chem. C, 2018, 122, 14326–14335 CrossRef CAS.
- M. Behtash, P. H. Joo, S. Nazir and K. Yang, Electronic structures and formation energies of pentavalent-ion-doped SnO2: first-principles hybrid functional calculations, J. Appl. Phys., 2015, 117, 175101 CrossRef.
- X. Lin, Y. Gao, M. Jiang, Y. Zhang, Y. Hou, W. Dai, S. Wang and Z. Ding, Photocatalytic CO2 reduction promoted by uniform perovskite hydroxide CoSn(OH)6 nanocubes, Appl. Catal., B, 2018, 224, 1009–1016 CrossRef CAS.
- H. Wan, R. Ma, X. Liu, J. Pan, H. Wang, S. Liang, G. Qiu and T. Sasaki, Rare cobalt-based phosphate nanoribbons with unique 5-coordination for electrocatalytic water oxidation, ACS Energy Lett., 2018, 3, 1254–1260 CrossRef CAS.
- X. Zhao, B. Pattengale, D. Fan, Z. Zou, Y. Zhao, J. Du, J. Huang and C. Xu, Mixed-node metal-organic frameworks as efficient electrocatalysts for oxygen evolution reaction, ACS Energy Lett., 2018, 3, 2520–2526 CrossRef CAS.
- O. Diaz-Morales, S. Raaijman, R. Kortlever, P. J. Kooyman, T. Wezendonk, J. Gascon, W. T. Fu and M. T. M. Koper, Iridium-based double perovskites for efficient water oxidation in acid media, Nat. Commun., 2016, 7, 12363 CrossRef CAS PubMed.
- G. Kresse and D. Joubert, From ultrasoft pseudopotentials to the projector augmented-wave method, Phys. Rev. B: Condens. Matter Mater. Phys., 1999, 59, 1758–1775 CrossRef CAS.
- G. Kresse and J. Furthmüller, Efficient iterative schemes for ab initio total-energy calculations using a plane-wave basis set, Phys. Rev. B: Condens. Matter Mater. Phys., 1996, 54, 11169–11186 CrossRef CAS PubMed.
- D. E. Appleman and H. T. Evans Jr., Indexing and refinement of powder diffraction data, U.S. Geol. Surv. Comput. Contrib., 1973, 20, 60 Search PubMed.
- H. Wan, P. Rong, X. Liu, L. Yang, Y. Jiang, N. Zhang, R. Ma, S. Liang, H. Wang and G. Qiu, Morphological evolution and magnetic property of rare-earth-doped hematite nanoparticles: promising contrast agents for T1-weighted magnetic resonance imaging, Adv. Funct. Mater., 2017, 27, 1606821 CrossRef.
- X. Wang, W. Gao, Z. Zhao, L. Zhao, J. P. Claverie, X. Zhang, J. Wang, H. Liu and Y. Sang, Efficient photo-electrochemical water splitting based on hematite nanorods doped with phosphorus, Appl. Catal., B, 2019, 248, 388–393 CrossRef CAS.
- S. Liu, H. Zhang, L. Xu and L. Ma, Synthesis of hollow spherical tin phosphides (Sn4P3) and their high adsorptive and electrochemical performance, J. Cryst. Growth, 2016, 438, 31–37 CrossRef CAS.
- V. Tallapally, R. J. A. Esteves, L. Nahar and I. U. Arachchige, Multivariate synthesis of tin phosphide nanoparticles: temperature, time, and ligand control of size, shape, and crystal structure, Chem. Mater., 2016, 28, 5406–5414 CrossRef CAS.
- S. Wu, H. Pang, W. Zhou, B. Yang, X. Meng, X. Qiu, G. Chen, L. Zhang, S. Wang, X. Liu, R. Ma, J. Ye and N. Zhang, Stabilizing CuGaS2 by crystalline CdS through an interfacial Z-scheme charge transfer for enhanced photocatalytic CO2 reduction under visible light, Nanoscale, 2020, 12, 8693–8700 RSC.
- N. T. Suen, S. F. Hung, Q. Quan, N. Zhang, Y. J. Xu and H. M. Chen, Electrocatalysis for the oxygen evolution reaction: recent development and future perspectives, Chem. Soc. Rev., 2017, 46, 337–365 RSC.
- S. Li, Y. Gao, N. Li, L. Ge, X. Bu and P. Feng, Transition metal-based bimetallic MOFs and MOF-derived catalysts for electrochemical oxygen evolution reaction, Energy Environ. Sci., 2021, 14, 1897 RSC.
- T. Ling, D. Y. Yan, Y. Jiao, H. Wang, Y. Zheng, X. Zheng, J. Mao, X. W. Du, Z. Hu, M. Jaroniec and S. Z. Qiao, Engineering surface atomic structure of single-crystal cobalt(II) oxide nanorods for superior electrocatalysis, Nat. Commun., 2016, 7, 12876 CrossRef CAS PubMed.
- S. R. Kelly, X. Shi, S. Back, L. Vallez, S. Y. Park, S. Siahrostami, X. Zheng and J. K. Nørskov, ZnO as an active and selective catalyst for electrochemical water oxidation to hydrogen peroxide, ACS Catal., 2019, 9, 4593–4599 CrossRef CAS.
- S. Siahrostami, G. L. Li, V. Viswanathan and J. K. Norskov, One- or two-electron water oxidation, hydroxyl radical, or H2O2 evolution, J. Phys. Chem. Lett., 2017, 8, 1157–1160 CrossRef CAS PubMed.
Footnote |
† Electronic supplementary information (ESI) available. See DOI: 10.1039/d1qi01169c |
|
This journal is © the Partner Organisations 2022 |