DOI:
10.1039/D2NR00558A
(Communication)
Nanoscale, 2022,
14, 3013-3019
Light-harvesting antennae based on copper indium sulfide (CIS) quantum dots†
Received
29th January 2022
, Accepted 7th February 2022
First published on 14th February 2022
Abstract
Copper indium sulfide quantum dots (CIS QDs) and their core–shell analogues (CIS@ZnS QDs) were functionalized with pyrene chromophores via a dihydrolipoamide bifunctional binding moiety: UV excitation of the pyrene chromophores resulted in sensitized emission of the CIS core because of an efficient energy transfer process; the core–shell hybrid system exhibits a 50% increased brightness when excited at 345 nm.
Introduction
Copper indium sulfide quantum dots (CIS QDs) are a valid alternative to conventional quantum dots based on cadmium or lead due to their non-toxicity and good optical properties. Indeed, CIS QDs exhibit high molar absorption coefficients over the entire visible spectral region (104–105 M−1 cm−1), elevated photoluminescence quantum yields (up to 70%) and long emission lifetimes (hundreds of nanoseconds).1–3 The quantum dot properties can be varied by functionalizing its surface with molecules that can provide new features, e.g. colloidal stability in a different solvent. For CIS QDs this functionalization can be performed via ligand exchange, therefore substituting the original capping molecules used to stabilize the nanoparticle during its synthesis with other ones possessing an appropriate anchoring group, namely thiols.4 By this approach, fluorophores can be conveniently used for the functionalization of CIS QDs to ameliorate the optical properties of the nanoparticle. In this context, nanoparticles can be decorated with a large number of chromophores that efficiently absorb the incoming light and then channel it to the quantum dot, which can emit at higher wavelengths, as assessed by our previous results on silicon quantum dots.5–9 Such systems are called light-harvesting antennae.10 More in general, light-harvesting antennae are organized multi-component systems in which many suitably organized chromophores absorb the incident light and then channel the excitation energy to a common acceptor component.10 Many examples of such systems are also reported in nature, e.g. the green plant antenna system, where the light is absorbed by chlorophylls and the energy funnelled in the reaction center. In the case of emissive structures, light-harvesting antennae are characterized by a superior brightness‡ if compared to the isolated chromophores. This approach is rather unexplored in the case of CIS QDs. The only example we found in the literature is that of a mixture of core–shell CIS@ZnS QDs with an organic fluorophore (POPOP) acting as white-light-emitting (WLE) hybrid composite: the authors evidenced a possible energy transfer from the fluorophore to the nanoparticles with limited efficiency (<30%).11
In the present work, we describe the functionalization of CIS QDs with a ligand containing a UV-absorbing chromophore, namely pyrene. The ligand possesses a dihydrolipoamide moiety, therefore two thiol anchoring moieties that allow an efficient replacement of the original single thiol-containing ligand.4 It is worth noting that dihydrolipoic acid derivatives have been widely used for the functionalization of cadmium-containing quantum dots,12–17 but some examples are also reported for CIS-based nanoparticles.18–20 The pyrene-functionalized nanoparticles are characterized by a superior absorption below 350 nm and a sensitized emission of the CIS QD upon pyrene excitation due to energy transfer from the organic chromophore towards the quantum dot. This represents the first example of a light-harvesting antenna based on copper indium sulfide quantum dots with enhanced brightness compared to the pristine sample without pyrene chromophores at the surface.
Results and discussion
Synthesis
Copper indium sulfide (CIS) QDs with or without a ZnS shell (hereafter named CIS@ZnS QDs and CIS QDs, respectively) were synthesized according to a heating-up procedure adapted from literature.21 The produced nanoparticles were stabilized and coated with an organic shell composed of 1-octanethiol, which allows a good dispersibility in non-polar solvents like chloroform. Afterwards, a partial ligand exchange with 6,8-dimercapto-N-(pyren-1-ylmethyl)octanamide (compound 2) was performed. This molecule was chosen since it contains a dihydrolipoic acid-derivative moiety and therefore possesses two thiol groups that reinforce the interaction with the surface of the nanoparticle and therefore allows a more efficient replacement with the native mono-thiol ligand.4 This was obtained firstly by amide coupling between lipoic acid and 1-pyrenemethylamine, followed by reduction of the sulfur–sulfur bond. The overall synthetical procedure is represented in Fig. 1. Ligand exchange was accomplished on both shelled and pristine quantum dots, yielding the samples named CIS@ZnS-py QDs and CIS-py QDs, respectively.
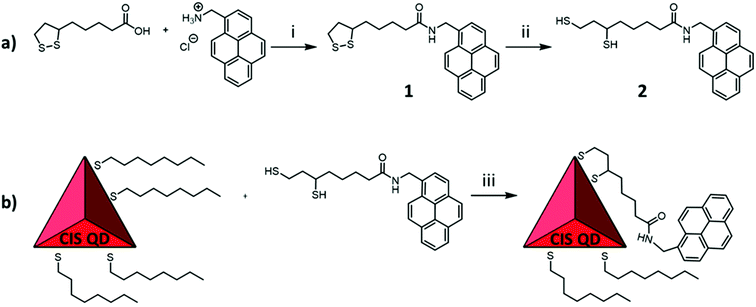 |
| Fig. 1 Schematic representation of the adopted strategy to functionalize copper indium sulfide QDs with pyrene. (a) The synthesis of the ligand involves firstly an amide coupling between (±)-α-lipoic acid and 1-pyrenemethylamine hydrochloride, yielding compound 1, followed by the reduction of the S–S bond, producing compound 2 (reaction conditions: i – HBTU, EDC, DIPEA, in DMF, 1 day; ii – NaBH4 in DMF/water, 4 hours). (b) Ligand exchange to 1-octanethiol passivated CIS QDs or CIS@ZnS QDs (iii – chloroform, overnight) For simplicity purposes, few ligands per nanocrystal have been drawn. | |
Structural and morphological characterization
The STEM-HAADF image of the sample CIS@ZnS QDs are reported in Fig. 2a. The distribution of the particles dimension is quite heterogenous with values from 2.5 nm up to 4 nm. The morphological characterization shows a triangular shape, particularly evident for some of the nanoparticles (see e.g., the one indicated by the red arrow in Fig. 2a), consistent with the tetrahedral shape previously reported in literature.21 The chalcopyrite crystalline phase was determined by Selected Area Electron Diffraction (SAED) indexing (Fig. 2b). The table in Fig. 2b displays all the measured d-spacings and the typical d-spacings of a chalcopyrite phase (CuInS2). High resolution micrograph (Fig. 2c) confirms the crystalline character of the nanoparticles (indicated by the red circles). The lattice spacing of 0.32 nm reported in the FFT inset (relative to the particle in the white square in Fig. 2c) is compatible with a tetragonal chalcopyrite phase. The same analyses were performed on the shell-free sample and are reported in Fig. S1a and S1b of the ESI.†
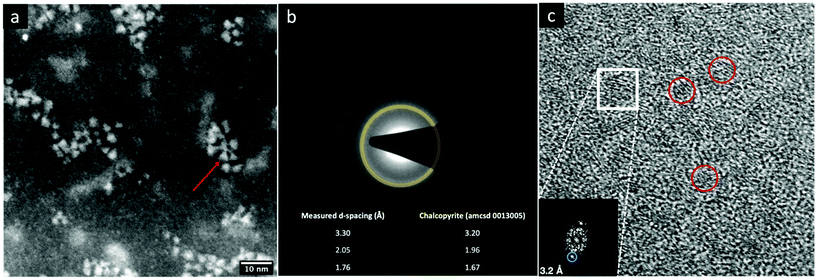 |
| Fig. 2 (a) High magnification STEM micrographs, selected area electron diffraction (SAED) and (c) high resolution (HR) TEM image of CIS@ZnS QDs. The FFT (fast Fourier transform) in the inset refers to the zone highlighted with the white square. | |
1H-NMR spectroscopy was used to verify the presence of pyrene attached onto CIS QDs surface. Fig. 3 shows the overlap between the spectrum of compound 1 (green line) and CIS@ZnS-py QDs (red line) in CDCl3. The main peaks are highlighted and associated to the protons of the molecule represented in the inset. The chemical shifts of the aromatic protons occur at around 7.7–8.3 ppm; signals at 3.0–3.5 ppm are due to the hydrogens in alpha position with respect to the sulfur atoms, at 2.0–2.2 ppm the band related to the protons linked to the carbon in proximity of the carboxylic group, while at about 1.2–1.4 ppm, the signals of the alkyl thiol chain are predominant, indication of a non-complete replacement of the native ligand. It is worth noting that the signals related to the hydrogens of the ligand attached to the quantum dot appear broadened due to the restricted motions that cause an enhancement of the relaxation times, as we also observed in previous works.5,6 For comparison purposes, the NMR spectrum of the physical mixture compound 2 – CIS@ZnS QDs is reported in the ESI (Fig. S2†).
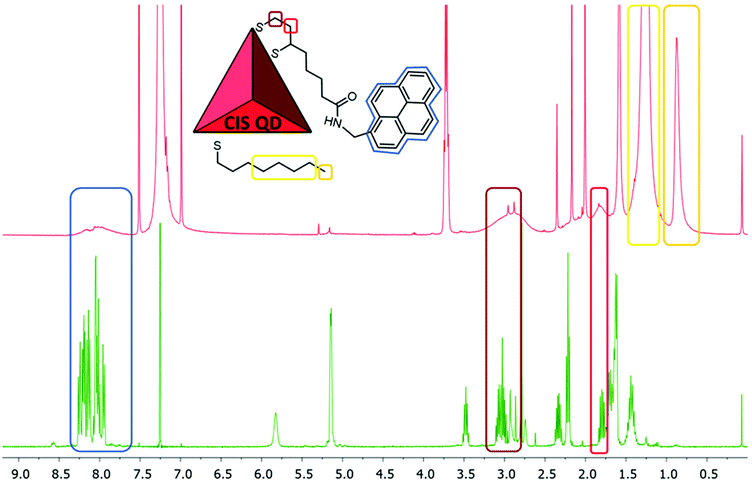 |
| Fig. 3
1H-NMR (400 MHz, CDCl3) spectra of compound 1 (green line) and CIS@ZnS-py (red line), which is schematized in the inset. The principal signals are highlighted to show the broadening of the peaks related to the attached molecules. | |
Photophysical properties
The absorption spectra of all the investigated sample of CIS QDs (Fig. 4) show the characteristic shoulder at ca.530 nm, which corresponds to the band gap transition. To better determine the corresponding wavelength, the local minimum of the second derivative of the spectra in the range 400–600 nm was evaluated, according to a procedure reported in literature (see Fig. S3 and S4 of the ESI† for further details).22 In every case, the electronic transition occurs at 530 nm, corresponding to an energy gap of about 2.3 eV.
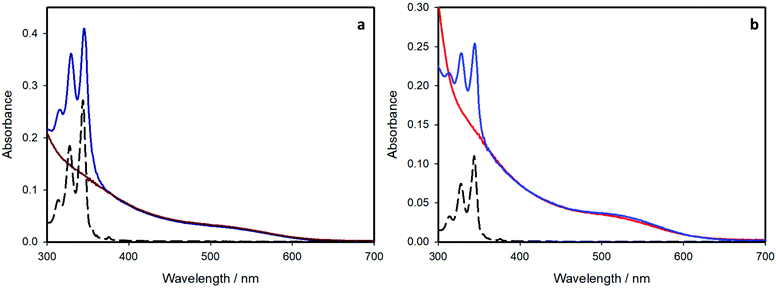 |
| Fig. 4 Absorption spectra of (a) CIS QDs (dark red line) and CIS-py QDs (dark blue line) and (b) CIS@ZnS QDs (red line) and CIS@ZnS-py QDs (blue line) in chloroform, compared to the absorption spectrum of pyrene (black dashed line). | |
In the case of the pyrene-functionalized samples (blue lines), the typical absorption features of CIS QDs are overlapped to those of pyrene, clearly visible at 300–350 nm (black dashed lines). Since each component maintains its own absorption properties, no significant interaction occurs between the organic chromophore and the inorganic nanoparticle in the ground-state.
The average number of chromophores per nanocrystal can be estimated from the absorption spectra and the molar absorption coefficients (ε) of the present species. The molar absorption coefficient of the pyrene-based ligand is 4 × 104 M−1 cm−1 at 345 nm. For CIS QDs, we relied on the work from Booth et al.22 who correlated the photophysical properties of copper indium sulfide quantum dots to their size. From their formula, it was possible to estimate the diameter of the CIS core for the samples, which is 3.6 nm and 2.7 nm for the shell-free and the ZnS-coated samples, respectively,§ which is in good accordance with the data obtained by TEM analysis. Moreover, the molar absorption coefficient computed at 530 nm (i.e. the first electronic transition), using the formula reported, is:
where
d is the diameter in nanometers and the result is expressed in M
−1 cm
−1. Consequently, at 530 nm, where pyrene does not absorb, the molar absorption coefficients for
CIS QDs and
CIS@ZnS QDs are 9.5 × 10
4 M
−1 cm
−1 and 3.3 × 10
4 M
−1 cm
−1, respectively. From these data the average number of pyrene moieties per nanocrystals is estimated to be about 20 for
CIS-py QDs and 3 for
CIS@ZnS-py QDs.
¶ In our opinion, this discrepancy can be due to a different surface reactivity towards the dihydrolipoamide either because of the different composition exposed (CuInS
2 or ZnS), or because of the different dimensions of the nanoparticles.
23
Upon selective excitation of the nanocrystal core at 450 nm, the emission spectrum features a bell-shaped band centered at 710 nm for the shell-free quantum dots and 650 nm for the ZnS-coated ones (Fig. 5 and Table 1). This blue-shift is well-known for such core–shell QDs and is associated to an interdiffusion of zinc ions into the CIS core24 and an etching of the core material.21 As expected,1,2,21 the emission quantum yield remarkably increases upon coating the nanocrystals with a ZnS shell.
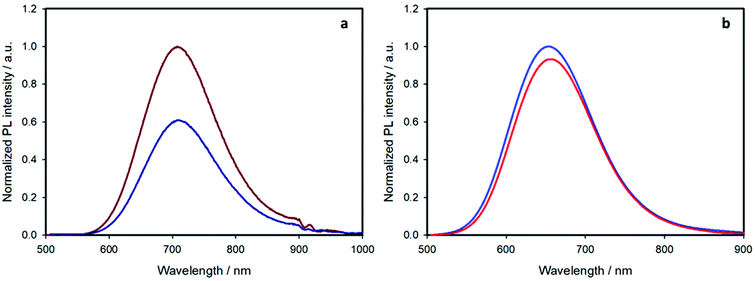 |
| Fig. 5 Emission spectra with intensity proportional to the corresponding emission quantum yields of (a) CIS QDs (dark red line) and CIS-py QDs (dark blue line) and (b) CIS@ZnS QDs (red line) and CIS@ZnS-py QDs (blue line) in chloroform; λex = 450 nm. The relative intensities in each panel reflect the corresponding emission quantum yields. | |
Table 1 Main photophysical properties of the studied quantum dots in air-equilibrated chloroform suspension
|
Pyrene emission |
Quantum dot emission |
λ
em (nm) |
τ
(ns) |
λ
em (nm) |
τ
(ns) |
PLQYc (%) |
Excitation at 340 nm, emission at 390 nm (pre-exponential factor between brackets).
Weighted average lifetime, excitation at 405 nm, emission at the corresponding maximum.
Excitation at 450 nm.
|
CIS QDs
|
— |
— |
710 |
70 |
1.9 |
CIS-py QDs
|
395 |
1.4 (0.08) |
710 |
50 |
1.2 |
20 (0.02) |
CIS@ZnS QDs
|
— |
— |
650 |
350 |
28 |
CIS@ZnS-py QDs
|
395 |
1.0 (0.04) |
650 |
260 |
30 |
20 (0.01) |
If we now consider the effect of ligand exchange on the emission quantum yield upon selective excitation of the nanocrystal at 450 nm, two opposite behaviors are observed. For the pristine nanoparticles, the emission quantum yield decreases (from 1.9% to 1.2%, Table 1), a phenomenon associated to the introduction of surface defects.1,25,26 On the other hand, the quantum yield of the shelled sample slightly increased (from 28% to 30%, Table 1).
Exciting at 345 nm, where the 44% and 68% of light is also absorbed by pyrene, for the core–shell and the shell-free samples, respectively, the emission spectra feature two additional bands (Fig. 6): a structured band with maximum at 395 nm, due to the emission of pyrene monomer, and a broad band peaked at 480 nm, correlated to the emission of pyrene excimer (characterized by a lifetime of 30 ns). For a solution of free pyrene chromophores, excimer emission is observed only at concentration higher than 10−4 M.27 In the present case, the sample concentration is lower than 5 × 10−5 M and the presence of excimer is related to pyrene close proximity at the nanocrystal surface, as also reported for other pyrene-functionalized quantum dots.6,28
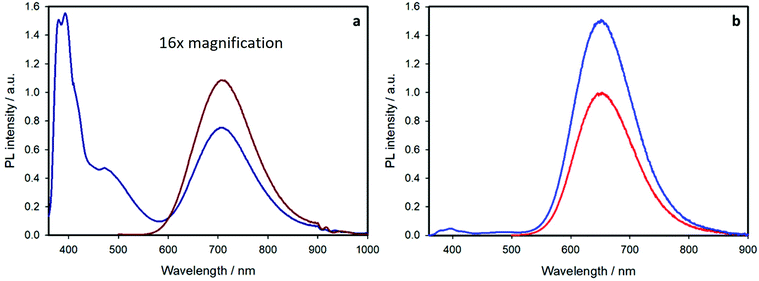 |
| Fig. 6 Emission spectra of equimolar suspensions of (a) CIS QDs (dark red line) and CIS-py QDs (dark blue line) and (b) CIS@ZnS QDs (red line) and CIS@ZnS-py QDs (blue line) in chloroform, exciting at 345 nm. The emission spectra in (a) are magnified by a 16 factor for comparison purposes. | |
The pyrene monomer emission at 395 nm is strongly quenched for both samples. This is confirmed by the lifetime measurements reported in Table 1. For both pyrene-containing samples, the emission decay at 395 nm (upon excitation at 340 nm) can be fitted by a bi-exponential function: the shorter component (ca. 1 ns) corresponds to the quenched pyrene chromophores attached at the nanocrystal surface and the long component (20 ns) is equal to that of the free pyrene ligand (compound 2 in air-equilibrated chloroform). The quenching efficiency is ca. 95% for both the samples. The kinetic rate constants for the quenching process are 6.6 × 108 s−1 and 9.5 × 108 s−1 for the shell-free and the core–shell system, respectively, as estimated by the comparison of the emission lifetimes (see ESI† for more details).
The presence of free pyrene ligand is expected: the binding of the ligand at the nanocrystal surface is dynamic, as previously reported for typical quantum dots,29–33 and an equilibrium between free and attached chromophores is contemplated. From the pre-exponential factors of the two lifetimes, we can estimate that the free pyrene ligand is around 20% for both samples (see ESI† for more details).
The above-discussed quenching process of the pyrene emission is related to energy transfer from the pyrene chromophore to the CIS core, as demonstrated by the excitation spectra performed at an emission wavelength of 700 nm. Fig. 7 displays the excitation spectra of the pyrene-functionalized samples (blue lines) compared to the chromophore-free samples (red lines) and their absorption spectra (green lines).
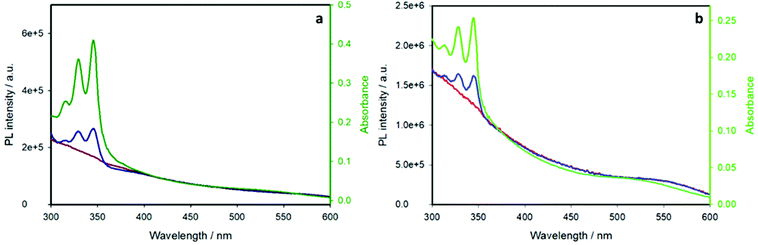 |
| Fig. 7 Excitation spectra of (a) CIS QDs (dark red line) and CIS-py QDs (dark blue line) compared to the absorption spectrum of CIS-py QDs (green line) and (b) CIS@ZnS QDs (red line) and CIS@ZnS-py QDs (blue line) with the absorption profile of CIS@ZnS-py QDs in chloroform. λem = 700 nm. | |
The pyrene-functionalized samples show the characteristic pyrene absorption peaks in the range 300–350 nm, demonstrating that the emission of the inner CIS QD is sensitized by pyrene: excitation of the organic chromophore at wavelengths lower than 350 nm results in an energy transfer to the nanoparticle, which emits at ca. 700 nm (a schematic representation of the energy level diagram and the energy transfer process is reported in Fig. S5†). This is, to the best of our knowledge, the first case of an efficient light-harvesting antenna based on copper indium sulfide quantum dots.
The brightness of the shell-free sample did not receive benefits by the enhanced absorption, because of the concomitant strong decrease of the intrinsic emission quantum yield of the nanoparticle upon ligand exchange, as previously discussed. On the contrary, for the core–shell sample, the brightness (relative to the emission at 700 nm upon excitation at 345 nm) increases of 50% for CIS@ZnS-py QDs compared to CIS@ZnS QDs. This result highlights the importance of coating the quantum dot with a ZnS layer to optimize their luminescence performance.
It is interesting to notice that CIS@ZnS QDs enhance their emissive properties after ligand exchange: most of the literature examples report an opposite trend because ligand exchange causes the introduction of defective states.34 In our opinion, this was possible due to (i) a partial exchange of the native alkyl thiol ligands, (ii) the linkage with an efficient chromophore and (iii) the presence of a protective ZnS shell.
The introduction of a higher amount of pyrene chromophore was also attempted: the reaction with a 4-times higher concentration of compound 2 yielded CIS@ZnS QDs functionalized with 13 pyrene units, demonstrating that the amount of the linked chromophore can be tuned on purpose. However, the signal of the pyrene excimer emission was predominant, due to the high amount of chromophore. Therefore, also the energy transfer efficiency was compromised (see ESI and Fig. S6† for more details).
Conclusions
We have successfully functionalized copper indium sulfide quantum dots and their core–shell analogues, namely CIS@ZnS QDs, with a dihydrolipoamide derivative bearing a pyrene moiety. The resulting systems act as light-harvesting antennae: pyrene increases the absorption properties and sensitizes the emission of the quantum dot at 700 nm. In particular, for the ZnS coated sample, we observed a substantial improvement of the photoluminescence properties of the quantum dot, with an increase of the brightness up to 50% when exciting at 345 nm. We believe that this is particularly advantageous for water-suspendable systems, since PLQY of quantum dots usually decreases when transferred in polar solvents.1,12,26 The enhanced absorption, along with the energy transfer process to the quantum dot can compensate this effect.
Functionalization with chromophores absorbing in the visible range and with a more stable binding are ongoing in our laboratories.
Conflicts of interest
There are no conflicts to declare.
Acknowledgements
The University of Bologna is gratefully acknowledged for financial support. The European Union's Horizon 2020 Research and Innovation Program (Grant Agreement No. 881603 Graphene Flagship Core Project 3, and Grant Agreement No. 861857 “CHALLENGES” project) is acknowledged.
Notes and references
- A. D. P. Leach and J. E. Macdonald, J. Phys. Chem. Lett., 2016, 7, 572–583 CrossRef CAS PubMed.
- H. Zhong, Z. Bai and B. Zou, J. Phys. Chem. Lett., 2012, 3, 3167–3175 CrossRef CAS PubMed.
- G. Morselli, M. Villa, A. Fermi, K. Critchley and P. Ceroni, Nanoscale Horiz., 2021, 6, 676–695 RSC.
- J. Kolny-Olesiak and H. Weller, ACS Appl. Mater. Interfaces, 2013, 5, 12221–12237 CrossRef CAS PubMed.
- R. Mazzaro, A. Gradone, S. Angeloni, G. Morselli, P. G. Cozzi, F. Romano, A. Vomiero and P. Ceroni, ACS Photonics, 2019, 6, 2303–2311 CrossRef CAS.
- G. Morselli, F. Romano and P. Ceroni, Faraday Discuss., 2020, 222, 108–121 RSC.
- M. Locritani, Y. Yu, G. Bergamini, M. Baroncini, J. K. Molloy, B. A. Korgel and P. Ceroni, J. Phys. Chem. Lett., 2014, 5, 3325–3329 CrossRef CAS PubMed.
- A. Fermi, M. Locritani, D. Carlo, M. Pizzotti, S. Caramori, Y. Yu, B. A. Korgel and P. Ceroni, Faraday Discuss., 2015, 185, 481–495 RSC.
- L. Ravotto, Q. Chen, Y. Ma, S. A. Vinogradov, M. Locritani, G. Bergamini, F. Negri, Y. Yu, B. A. Korgel and P. Ceroni, Chem, 2017, 2, 550–560 CAS.
- F. Romano, Y. Yu, B. A. Korgel, G. Bergamini and P. Ceroni, Top. Curr. Chem., 2016, 374, 89–106 CrossRef PubMed.
- P. Ilaiyaraja, P. S. V. Mocherla, T. K. Srinivasan and C. Sudakar, ACS Appl. Mater. Interfaces, 2016, 8, 12456–12465 CrossRef CAS PubMed.
- H. T. Uyeda, I. L. Medintz, J. K. Jaiswal, S. M. Simon and H. Mattoussi, J. Am. Chem. Soc., 2005, 127, 3870–3878 CrossRef CAS PubMed.
- N. Zhan, G. Palui, M. Safi, X. Ji and H. Mattoussi, J. Am. Chem. Soc., 2013, 135, 13786–13795 CrossRef CAS PubMed.
- G. Palui, T. Avellini, N. Zhan, F. Pan, D. Gray, I. Alabugin and H. Mattoussi, J. Am. Chem. Soc., 2012, 134, 16370–16378 CrossRef CAS PubMed.
- K. Susumu, T. Pons, I. L. Medintz and H. Mattoussi, Mater. Res. Soc. Symp. Proc., 2007, 1019, 76–81 CrossRef.
- J. Park, J. Nam, N. Won, H. Jin, S. Jung, S. Jung, S. H. Cho and S. Kim, Adv. Funct. Mater., 2011, 21, 1558–1566 CrossRef CAS.
- J. Zhou, Y. Liu, J. Tang and W. Tang, Mater. Today, 2017, 20, 360–376 CrossRef CAS.
- J. Feng, M. Sun, F. Yang and X. Yang, Chem. Commun., 2011, 47, 6422–6424 RSC.
- L. Li, T. J. T. Daou, I. Texier, T. K. Chi, T. T. Kim Chi, N. Q. Liem and P. Reiss, Chem. Mater., 2009, 21, 2422–2429 CrossRef CAS.
- T. Pons, E. Pic, N. Lequeux, E. Cassette, L. Bezdetnaya, F. Guillemin, F. Marchal and B. Dubertret, ACS Nano, 2010, 4, 2531–2538 CrossRef CAS PubMed.
- L. Li, A. Pandey, D. J. Werder, B. P. Khanal, J. M. Pietryga and V. I. Klimov, J. Am. Chem. Soc., 2011, 133, 1176–1179 CrossRef CAS PubMed.
- M. Booth, A. P. Brown, S. D. Evans and K. Critchley, Chem. Mater., 2012, 24, 2064–2070 CrossRef CAS.
- L. Liu, X. Zhang, L. Ji, H. Li, H. Yu, F. Xu, J. Hu, D. Yang and A. Dong, RSC Adv., 2015, 5, 90570–90577 RSC.
- J. Park and S. W. Kim, J. Mater. Chem., 2011, 21, 3745–3750 RSC.
- M. Booth, R. Peel, R. Partanen, N. Hondow, V. Vasilca, L. J. C. Jeuken and K. Critchley, RSC Adv., 2013, 3, 20559–20566 RSC.
- N. Tsolekile, S. Parani and M. C. Matoetoe, Nano-Struct. Nano-Objects, 2017, 12, 46–56 CrossRef CAS.
-
V. Balzani, P. Ceroni and A. Juris, Photochemistry and Photophysics - Concepts, Research, Applications, Wiley-VCH, 1 edn, 2015 Search PubMed.
- R. Mazzaro, M. Locritani, J. K. Molloy, M. Montalti, Y. Yu, B. A. Korgel, G. Bergamini, V. Morandi and P. Ceroni, Chem. Mater., 2015, 27, 4390–4397 CrossRef CAS.
- S. Silvi and A. Credi, Chem. Soc. Rev., 2015, 44, 4275–4289 RSC.
- M. Green, J. Mater. Chem., 2010, 20, 5797–5809 RSC.
- A. Loiudice, O. Segura Lecina, A. Bornet, J. M. Luther and R. Buonsanti, J. Am. Chem. Soc., 2021, 143, 13418–13427 CrossRef PubMed.
- R. R. Knauf, J. C. Lennox and J. L. Dempsey, Chem. Mater., 2016, 28, 4762–4770 CrossRef CAS.
- W. Liu, A. B. Greytak, J. Lee, C. R. Wong, J. Park, L. F. Marshall, W. Jiang, P. N. Curtin, A. Y. Ting, D. G. Nocera, D. Fukumura, R. K. Jain and M. G. Bawendi, J. Am. Chem. Soc., 2010, 132, 472–483 CrossRef CAS PubMed.
- Z. Long, W. Zhang, J. Tian, G. Chen, Y. Liu and R. Liu, Inorg. Chem. Front., 2021, 8, 880–897 RSC.
Footnotes |
† Electronic supplementary information (ESI) available. See DOI: 10.1039/d2nr00558a |
‡ Brightness is defined as the product between the molar absorption coefficient at the excitation wavelength and the sensitized emission quantum yield of the system. |
§ The formula used to compute the diameter core d from the emission maximum wavelength λ in nm is: d = 68.952 − 0.2136 λ + 1.717 × 10−4λ2. |
¶ The reported values have been corrected for the pyrene chromophores not attached at the nanocrystal surface, as evaluated by the analysis of emission lifetimes. |
|
This journal is © The Royal Society of Chemistry 2022 |
Click here to see how this site uses Cookies. View our privacy policy here.