DOI:
10.1039/D1NR03320D
(Paper)
Nanoscale, 2022,
14, 86-98
Transparent polycarbonate coated with CeO2 nanozymes repel Pseudomonas aeruginosa PA14 biofilms†
Received
24th May 2021
, Accepted 16th November 2021
First published on 17th November 2021
Abstract
Highly transparent CeO2/polycarbonate surfaces were fabricated that prevent adhesion, proliferation, and the spread of bacteria. CeO2 nanoparticles with diameters of 10–15 nm and lengths of 100–200 nm for this application were prepared by oxidizing aqueous dispersions of Ce(OH)3 with H2O2 in the presence of nitrilotriacetic acid (NTA) as the capping agent. The surface-functionalized water-dispersible CeO2 nanorods showed high catalytic activity in the halogenation reactions, which makes them highly efficient functional mimics of haloperoxidases. These enzymes are used in nature to prevent the formation of biofilms through the halogenation of signaling compounds that interfere with bacterial cell–cell communication (“quorum sensing”). Bacteria-repellent CeO2/polycarbonate plates were prepared by dip-coating plasma-treated polycarbonate plates in aqueous CeO2 particle dispersions. The quasi-enzymatic activity of the CeO2 coating was demonstrated using phenol red enzyme assays. The monolayer coating of CeO2 nanorods (1.6 μg cm−2) and the bacteria repellent properties were demonstrated by atomic force microscopy, biofilm assays, and fluorescence measurements. The engineered polymer surfaces have the ability to repel biofilms as green antimicrobials on plastics, where H2O2 is present in humid environments such as automotive parts, greenhouses, or plastic containers for rainwater.
Introduction
Polycarbonate (PC) is an engineering plastic with a wide range of applications that is used as a substitute for glass1 for large-area coverings and safety glazing, e.g., in the automotive industry, in greenhouses, or for outdoor covers, as it is much softer and easily moldable to meet modern design and production requirements. However, low hardness, scratch resistance, and low resistance to photo- or biocorrosion limit the use of PC for many industrial applications. It is therefore of interest to modify or improve the surface of the polymer without changing its bulk properties. PC is very transparent to visible light and has better light transmission properties compared to different types of silica glass. However, PC unfortunately has a low glass transition temperature of ∼150 °C and gradually softens above this temperature, and begins to flow above ∼300 °C. To allow PC to be used in applications exposed to weathering or UV radiation, a special surface treatment is required. The surface properties of plastic surfaces can be specifically modified by coating. There are a variety of strategies to functionalize polymer surfaces for biomedical applications by plasma treatment, superhydrophobic surfaces, quaternary ammonium compounds, chitosan-containing surfaces, or embedded nanoparticles (NPs).2–7
Coating polymer surfaces with metal oxide nanoparticles allows potentially interesting commercial applications such as self-cleaning or antibacterial properties.8 Polymer surfaces coated with TiO2 nanoparticles are more scratch-resistant and have antibacterial properties due to the photocatalytic properties of TiO2 but a recent classification of nano-TiO2 as a “suspected carcinogen” by the EU may lead to restrictions or even a ban on their chemical use in consumer products.9 Coating with ZnO/TiO2 NPs may lead to self-cleaning, while coating with nano-Ag or carbon nanotubes may confer antibacterial properties.10 A common problem with silver nanoparticles or antibiotics is the decrease in the antibacterial activity due to the leaching of the active compound with time, leading to materials with a limited life span and metal (Cu, Ag) nanoparticles that are harmful to the environment.11 Tailored surfaces to which bacteria and cells cannot adhere represent an alternative approach to combat bioadhesion.12 Surfaces coated with poly(ethylene oxide) or polymers with zwitterionic groups are known to suppress nonspecific protein adsorption and bacterial adhesion.13,14 Superhydrophobic antibacterial coatings with polycarbonate have been proposed for catheters to prevent infections.15
Antibiotics are the most important tools in the fight against bacteria. However, bacteria are adaptable and can build up resistance to antibiotics.16 For this reason, research is constantly being conducted to find other ways of combating them. One of these antibiotic-resistant bacteria is the Gram-negative bacterium Pseudomonas aeruginosa (P. Aeruginasa). It is among the most common causes of respiratory pneumonia, wound infections, and urinary tract infections worldwide.17
Our approach to fabricating biofilm-inhibiting surfaces of such bactaria is non-toxic and inspired by nature: using oxidizing halogenating enzymes (haloperoxidases, HPOs), seaweeds catalyze the oxidation of halides (Cl− and Br−) with hydrogen peroxide (H2O2) to form hypohalous acids (HOCl and HOBr).18–20 In the presence of ubiquitous Br− ions and H2O2, generated in sunlight, CeO2 is—by virtue of its catalytic activity—a functional substitute for haloperoxidases, which can also brominate AHL signal molecules so that communication between the bacteria is disrupted (quorum quenching) and biofilm formation is inhibited in a non-biocidal way.21 Metal complexes,22–24 V2O5,25 and CuO26 or ceria21,27 nanoparticles have been reported to act as “nanozymes” (inorganic nanoparticle-based artificial enzymes) by mimicking the HPO activity.28,29 Ceria nanoparticles are readily and inexpensively available, non-toxic,30 highly insoluble (pKL = 60),31 stable over a wide pH range,32 and therefore, environmentally safe. Thus, they are ideally suited for real-world applications.22,33–35
The important problems in the coating of polymer surfaces are the low temperature stability and the low surface energy of the polymer. These lead to poor surface wetting, which results in weak adhesion.36 Polymer surfaces can be coated with metal oxides in various ways to achieve potentially interesting commercial applications such as self-cleaning or antibacterial properties.37 Different low-temperature techniques such as pulsed laser deposition (PLD),38 magnetron sputtering,39 spray pyrolysis,40 and metal organic chemical vapor deposition (MOCVD)41 are available to prepare surface-active CeO2 films at low temperature. However, most physical vapor deposition processes have problems in depositing large area films, require complex optimization of deposition conditions, and the substrates are silicon or transition metals. Wet chemical deposition is suitable for producing thin layers on large surfaces but drying metal oxide films on heat-sensitive polymer substrates is problematic42 as it leads to thin films with low surface area and low catalytic activity. A quick and simple method to increase the surface energy and adhesion is plasma treatment with oxygen (O2) or argon (Ar).33,41 As a result, oxygen-containing groups such as hydroxyl, carbonyl, and carboxyl groups are incorporated on the surface, thus increasing the polarity.42
Last but not least, CeO2 nanoparticles for surface coatings must be available in sufficiently large quantities and be well dispersible to achieve homogeneous coatings as agglomerates otherwise impair the optical appearance and deteriorate the surface roughness of the material. Since nanoparticles are only reactive when exposed at the surface, coating approaches are much more material efficient and effective than embedding particles in a bulk polymer.21 We present (i) a large scale synthesis of CeO2 nanorods along with (ii) a polycarbonate coating approach that allows easily scalable process parameters and thus potential mass production. (iii) We demonstrate by scanning electron microscopy and scanning probe techniques the bacterial repellancy of the film and (iv) show the quorum quenching mechanism through the production of the virulence factor pyocyanin in response to the haloperoxidase-like activity of the CeO2 coating. The proven non-toxicity of the coating makes polycarbonate surfaces promising for resisting biofilm formation in daily use where H2O2 is present in humid environments such as greenhouses or plastic containers for rainwater (H2O2 production in ground water,43 the atmosphere,44 or in natural water exposed to sunlight).45
Results and discussion
Particle preparation and analysis
A freshly prepared cerium(III) hydroxide dispersion was used for the synthesis of ceria nanoparticles in aqueous media with hydrogen peroxide as the oxidant and nitrilotriacetic acid (NTA) as the capping agent. The capping agent ensured the formation of a stable suspension without inhibiting the haloperoxidase activity of the CeO2 nanoparticles by forming surface complexes. In addition, the capping agent can be used to produce a stable suspension that is used for polycarbonate coating. The reaction can be easily scaled up to the 100 g scale in batch operation for coating large surface areas.
Fig. 1A displays the TEM micrograph of a sample obtained after centrifugation from an aqueous nanoparticle dispersion. The reaction yielded nanorods with a length of 100–200 nm and a width of 10–15 nm, suitable for the transparent coating of surfaces. The X-ray powder diffraction pattern confirmed the formation of single phase CeO2 (Fig. 1C) in the bulk sample. The nanoparticles were carefully washed three times by centrifugation at 9000 rpm for 30 min. We avoided irreversible agglomeration by preventing complete solvent evaporation. NTA is well-suited as a ligand for CeO2 because the carboxylate groups are hard donors for ionic bonding to Ce4+ ions. NTA is thought to form a complex with cerium surface atoms, in which the three carboxylate groups are bound by ionic interactions and the N atom of the NTA ligand by dative interactions.46 Light scattering experiments revealed an average hydrodynamic radius of 115 ± 79 nm for CeO2 nanoparticles with NTA surface ligands.
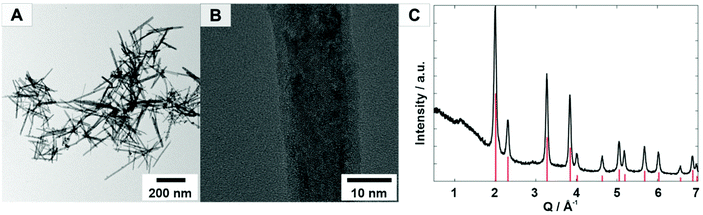 |
| Fig. 1 (A, B) TEM image of the CeO2 nanorods and (C) X-ray powder diffraction pattern of a CeO2 sample. Red ticks indicate the calculated reflection positions for CeO2 based on the structural data from the JCPDS data bank (JCPDS# 00-034-0394). | |
Haloperoxidase activity
The haloperoxidase-like activity of CeO2 nanorods was demonstrated with a phenol red bromination assay. In this assay, a phenolsulfonphthalein indicator (phenol red, PR) is brominated in aqueous solution in the presence of Br− and H2O2 to bromophenol blue (3′,3′′,5′,5′′-tetrabromophenolsulfonphthalein, Br4PR). The reaction can be monitored spectrophotometrically because the educt and product have different distinct absorption maxima at λmax,PR = 430 nm, and λmax,Br4PR = 590 nm. Michaelis–Menten enzyme kinetics were assumed because the haloperoxidase-like activity was dependent on the H2O2 concentration. The kinetics were measured for different concentrations of H2O2 and fitted with the Hill equation (eqn (1)) (Fig. 2).47 |  | (1) |
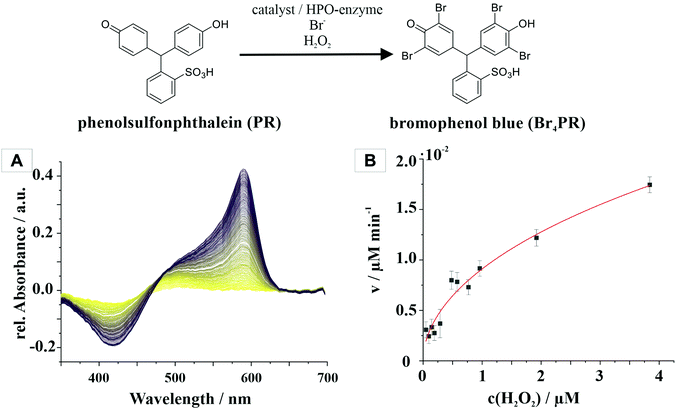 |
| Fig. 2 Haloperoxidase-like activity of CeO2 nanoparticles determined with a phenol red bromination assay. (A) Time-dependent UV-vis spectra indicate the change in the oxidative bromination from phenol red (PR) to bromophenol blue (Br4PR). (B) Shows the Michaelis–Menten kinetics for the substrate H2O2 using the CeO2 enzyme mimic. | |
The Michaelis constant Km is a measure of the affinity of the enzyme, in this case CeO2, for the H2O2 substrate with the concentration [S]0. The value Km has a reciprocal relationship with the affinity, i.e., the higher the constant, the weaker the binding strength between the enzyme and the substrate. For CeO2, the constant is 0.85 mM, which indicated a high affinity for H2O2. The maximum rate of the reaction is described by the vmax value, which is 0.021 μM min−1 for the CeO2 nanorods.
The bromination rate depends on (i) the surface charge, (ii) BET surface area, and (iii) the presence or nature of surface ligands. CeO2 particles have a ζ potential of −22.5 ± 0.9 mV and a BET surface of 81.606 m2 g−1. They were functionalized with nitrilotriacetic acid (NTA) to achieve better dispersibility in aqueous solution. This is an important prerequisite for achieving homogeneous surface coating.
|  | (2) |
The rate of reaction (ROR) was determined to relate to the conversion rate to the available surface area (eqn (2)).48 The maximum rate vmax = 0.021 μM min−1 and the specific surface gave a reaction of ROR(CeO2) = 0.0087 μmol m−2 min−1.
CeO2 nanoparticles catalyze the oxidative bromination of N-(3-oxo-acyl)homoserine lactones (AHLs) at the activated C2 atom, forming mono- and dibromo-3-oxo-dodecanoylhomoserine lactones. The bromination is complete after approx. 60 min. The brominated AHLs are hydrolyzed in the subsequent step, the hydrolysis being complete after approx. 5 h, as demonstrated by inductively coupled plasma mass spectrometry (ICP-MS). Pseudomonas Aeroginosa bacteria use 3-oxo-dodecanoylhomoserine lactone to communicate with each other and to determine their cell count (quorum sensing, QS),20 where biofilm formation is under control of QS through the production of the virulence factor pyocyanin in response to the haloperoxidase-like activity (Fig. 6, vide infra and ESI†).
Coating polycarbonate with ceria nanoparticles
Polycarbonate has a hydrophobic surface, which leads to a small surface energy. The surface was treated in an oxygen plasma. Fig. 3 shows the contact angle of untreated and oxygen plasma-treated polycarbonate. The contact angle of the pristine polycarbonate surface was 86.75° and decreased to 26.88° after a 20 min oxygen plasma treatment, i.e., the surface became significantly more hydrophilic. Hofrichter et al.49 showed by XPS spectroscopy that plasma treatment increases the oxygen content and decreases the carbon content by generating oxygen-containing groups such as –(C–O)–, –(C
O)–, and –(O–C
O)– on the polycarbonate surface. The binding of ceria nanoparticles to polycarbonate occurs by the ester condensation of their surface hydroxyl groups with carboxylate surface groups or by hydrogen bonding between the polar groups on the polymer surface and the ceria particles.50,51
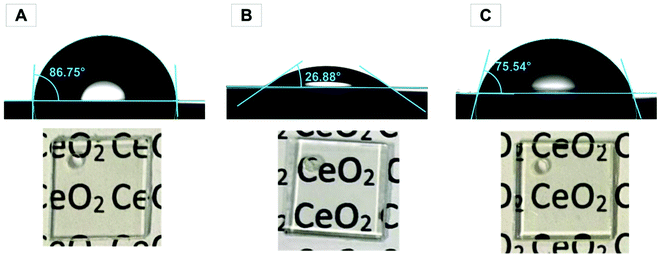 |
| Fig. 3 Contact angle of a water drop on (A) untreated (B) O2 plasma-treated, and (C) CeO2-coated polycarbonate with an optical microscopy image of the polycarbonate plates (1 × 1 cm). | |
The smaller contact angle of 26.88° shows a significantly higher hydrophilic surface, which facilitates the contact of the suspension with the surface of the polycarbonate and thus enables the coating with ceria nanoparticles. For the dip coating process, oxygen plasma-treated polycarbonate slides were treated in aqueous ceria suspensions and slowly pulled out of the dispersion to obtain a homogeneous coating. After dip coating, the plates were annealed at 110 °C for 16 h. Excess CeO2 was carefully washed off before further testing. Due to the hydrophilicity of ceria on the surface, the contact angle after the coating process showed a 12.74° lower value than that of the untreated polycarbonate sample, i.e., the coating with CeO2 nanorods increased the hydrophilicity of the polycarbonate surface. The ceria content at the surface was determined by ICPMS (1.6 μg cm−2). Leaching tests showed no trace of ceria in the water supernatant after 7 days. Fig. 3 demonstrates an image of untreated, plasma-treated, and CeO2-coated PC, where there is no visual difference. In addition, this assumption is proven by spectroscopic investigation using UV/Vis spectroscopy (Fig. S9†). The polycarbonate absorbs light in the UV range for all the three samples.
The surface morphology of the untreated and CeO2-coated polycarbonate was characterized after 10 days of storage at 7 °C by atomic force microscopy (Park System NX10, Fig. 4). When comparing the height images, the untreated polycarbonate showed a smooth topography with a low surface roughness of Rq = 1.5 nm; the polycarbonate surface coated with cerium oxide nanorods exhibited a slightly higher roughness of Rq = 5.8 nm.
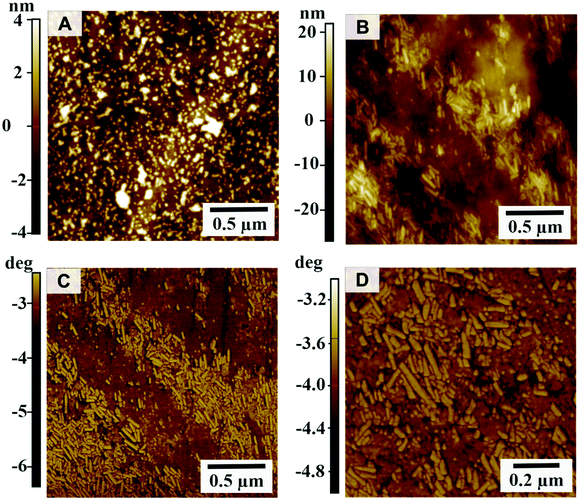 |
| Fig. 4 Surface morphology analyzed by AFM. Height images of (A) untreated polycarbonate slides, (B) polycarbonate slides coated with CeO2 nanorods, and (C+D) phase images of CeO2-coated polycarbonate slides. | |
The size of the particles can be inferred from the individual profiles.52 With a typical length of 80–200 nm (Fig. S4, ESI†) and an apparent typical thickness of 27–47 nm (see Fig. S3† red arrows), the CeO2 nanorods appear to have a lower aspect ratio compared to the TEM images (Fig. 1). However, this difference originating from the tip convolution effects is bypassed when comparing the measured z-heights. Typical thicknesses of 6.2–12.7 nm (see green arrows, Fig. S3†) well fit with the observed aspect ratio. The CeO2 nanoparticles show a distinct contrast to the polycarbonate substrate in the phase (Fig. 4C and D). The phase image of the CeO2-coated polycarbonate plates shows the homogeneous distribution of the nanorods (Fig. 4B–D) compared to the uncoated polycarbonate slides.
The surface was also examined by scanning electron microscopy (SEM) (Fig. S1†). EDX mapping was conducted to analyze the elemental distribution and verify the presence of CeO2. The surface was sputtered with a 1 nm gold layer to achieve a better contrast. As reported in the literature, Ce has a characteristic peak in the X-ray emission spectrum of Lα: 4.839 keV, which is reflected by the signal at 4.9 eV in the recorded EDX spectrum.53 The low signal can be explained by the low concentration of CeO2 on the surface. Also visible is the characteristic Mα signal of gold at 2.12 keV, which is attributed to the sputtered gold layer on the surface. Moreover, the signals of oxygen (O) at 0.53 keV (Kα) and carbon (C) at 0.28 keV (Kα) can be recognized.
CeO2-Coated polycarbonate inhibits biofilm formation
The coated plates were tested regarding their potential to inhibit the biofilm formation of P. aeruginosa, a prominent Gram-negative bacterium, which is responsible for up to 10% of all healthcare-associated infections (HAI) in Germany as well as spoiling drinking water through its occurrence in drinking water pipes.54 Due to its strong biofilm-forming behavior, simple surface cleaning with disinfectants is often not sufficient and gives rise to strains resistant to antibiotics.
To understand the effect of CeO2 nanoparticles on biofilm formation of P. aeruginosa, crystal violet staining assay was performed to determine the amount of the adherent cells (Fig. 5A). After culturing the bacteria on polycarbonate for 72 h, the attached cells were stained with crystal violet solution. Biofilm formation was reduced by ∼75% on the coated PC surfaces. The thickness of the biofilms on the PC surfaces was quantified by confocal laser scanning microscopy (Fig. 5B). For this purpose, the bacterial biofilms were cultivated for 72 h at 30 °C on different PC samples placed in 24-well plates. The attached cells were then stained with SYTO9 and propidium iodide, two specific dyes staining the total cell count in the biofilm. The CeO2-coated plates showed a significant reduction in the biofilm thickness to ∼20 μm compared to the uncoated PC surfaces, where the biofilm thickness was more than 50 μm. In addition, the biofilm on the coated plates grows in microcolonies and is not evenly distributed over the entire surface as it is on the uncoated plates.
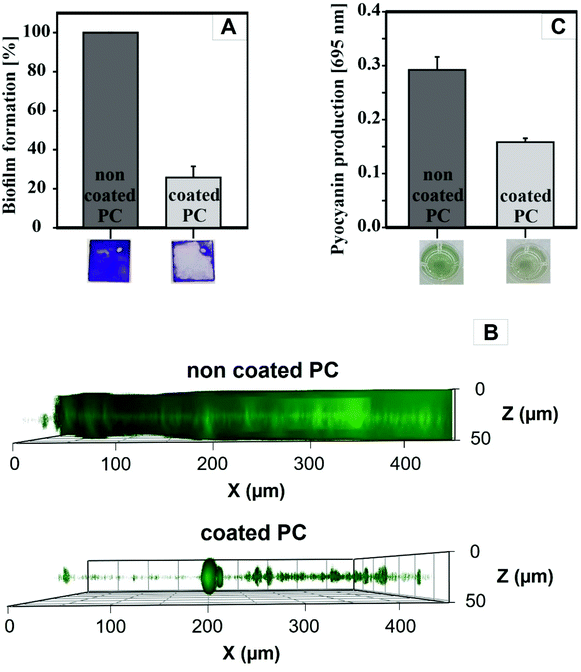 |
| Fig. 5 (A) Bacterial biofilm formation on non-coated and CeO2-coated polycarbonate plates. Crystal violet staining assay of P. aeruginosa grown in lysogeny broth. The stained plates are shown in the lower part of the plots, (B) confocal laser scanning microscopy of P. aeruginosa biofilm on coated and non-coated surfaces. The non-coated plate served as the positive control. The planktonic cells were removed, and the remaining bacteria attached to the surfaces were stained with SYTO 9 and propidium iodide for 30 min at 30 °C. The effect of the CeO2-coated plates on the cell attachment was then visualized. (C) Effect of CeO2-coated polycarbonate surfaces versus non-coated plates on pyocyanin production in P. aeruginosa. Error bars represent the standard deviation of three independent experiments. | |
We analyzed whether the biofilm inhibiting effect of CeO2 is mediated by blocking bacterial quorum sensing, a process designated as quorum quenching. In P. aeruginosa, the biosynthesis of pyocyanin, another virulence factor besides biofilm formation, is under control of quorum sensing. Therefore, we analyzed the pyocyanin production on CeO2-coated and non-coated PC surfaces (Fig. 5C). For that purpose, the bacteria were grown for 72 h at 30 °C on uncoated and coated polycarbonate plates. The plates were then removed, and the remaining culture fluid was analyzed for pyocyanin. The CeO2-coated polycarbonate plates reduced the pyocyanin formation of the biofilm grown on the surface by 54% compared to the uncoated plate. This indicates that quorum sensing-dependent pyocyanin formation is reduced by CeO2 nanoparticles and lends support to the idea that CeO2 also inhibits bacterial biofilm formation by quorum quenching. The remaining pyocyanin synthesis might be due to pelagic bacteria in the well, which are not directly exposed to the CeO2 nanoparticles.
Assay for bacterial viability
To understand the process of biofilm colonization, it is important to analyze whether biofilm formation is reduced by killing the bacteria or by suppressing the group behavior.
Live/dead fluorescence assays were performed using SYTO 9 and propidium iodide (PI) dye. SYTO 9 stains only live cells, while PI penetrates only dead or damaged cells.55 In this way, the number of living and dead cells present in the biofilm can be analyzed. Fig. 6 shows that the live/dead ratio does not change significantly for the CeO2/polycarbonate plates and the uncoated reference, while the total amount of bacteria decreases.55 In addition, the biofim-inhibiting activity of the CeO2 nanorods was investigated using atomic force microscopy (AFM) and scanning electron microscopy (SEM) (Fig. S10†) for the P. aeruginosa cell culture.
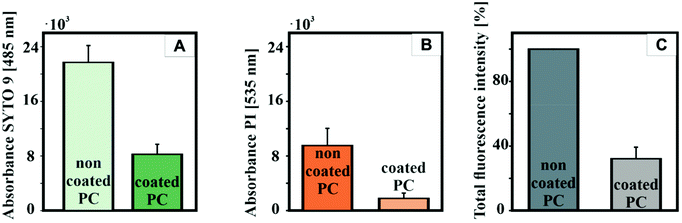 |
| Fig. 6 Quantification of P. aeruginosa biofilm formation on non-coated and CeO2-coated polycarbonate plates. Blank plates served as yjr positive control. The planktonic cells were removed, and the remaining bacteria attached to the plates were stained with SYTO 9 (A) and propidium iodide (PI) (B). (C) Finally, the effect of the incorporated CeO2 nanorods on bacterial biofilm formation was quantified by measuring the absorption at 485 nm for SYTO9 and 535 nm for propidium iodide, respectively. Error bars represent the standard deviation of three independent experiments. | |
Bacteria were cultured on CeO2-coated and uncoated polycarbonate substrates in the presence of halide and H2O2. Fig. 7A+B show a homogeneous coating of bacteria with a size of 1–1.5 μm (Fig. S6†) on an uncoated polycarbonate substrate. Due to non-interrupted communication (QS) between the bacteria, they were able to generate an extracellular polymeric substance (EPS) matrix, subsequently forming a confluent biofilm and proliferate. In contrast, Fig. 7C shows a single isolated bacterium on the surface of the CeO2-coated substrate. Here, the bacteria were brought to the surface with the LB medium but the interrupted QS prevented proliferation and inhibited biofilm formation because the AHLs used for interbacterial communication were modified and therefore inactivated by the CeO2 nanozyme in the presence of the halide Br− and H2O2 by oxidative bromination.22
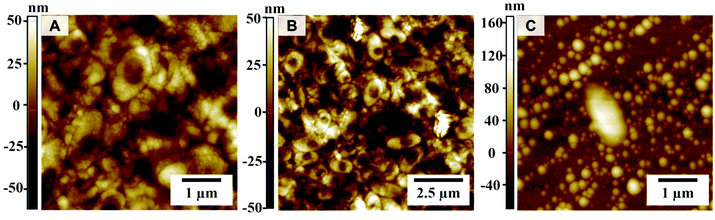 |
| Fig. 7 Atomic force microscope (AFM) images of non-coated (A+B) and CeO2-coated polycarbonate (C) with the P. aeruginosa culture. | |
Cytotoxicity of CeO2 nanorods
A cell viability assay was made to determine the ability of the cells to maintain a state of survival in the presence of the CeO2 nanorods. The cytotoxicity of the CeO2 nanorods was examined in HeLa and RAW 264.7 cells using a luminescence cell viability assay. The CeO2 particles showed no cytotoxicity after 24 h of incubation, even for concentrations up to 300 μg mL−1.
The viability of yjr HeLa cells is constant at 100% after 2 and 24 h, even at high concentrations (Fig. 8). Similarly, the viability of the RAW 264.7 cells remains constant at 100% with increasing particle concentration. Thus, we have shown that CeO2 coating is a non-cytotoxic approach to prevent the adhesion of bacteria to a polymer surface.
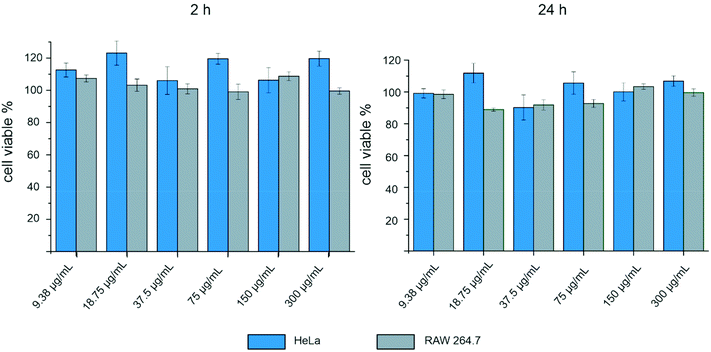 |
| Fig. 8 Effect of CeO2–NTA on the cell viability of HeLa and RAW 264.7 cells after 2 h and 24 h at different nanoparticle concentrations. | |
Conclusion
We have fabricated highly transparent CeO2-coated polycarbonate plates with high wettability and high catalytic haloperoxidase activity to prevent bacterial adhesion. Multigram quantities of highly dispersible CeO2 nanorods with diameters of 10–15 nm and lengths of 100–200 nm were synthesized from aqueous dispersions of cerium(III) hydroxide by oxidation with hydrogen peroxide in the presence of nitrilotriacetic acid (NTA) as the capping agent.
Transparent CeO2-coated polycarbonate panels were prepared by oxygen plasma treatment of the polycarbonate plates, followed by dip coating with CeO2/NTA dispersions. Low concentrations (as low as 1.6 μg cm−2, corresponding to a monolayer coating of CeO2 nanorods, analyzed by atomic force microscopy) were able to reduce P. aeruginosa biofilm formation on polycarbonate by at least 75%. Using cytotoxicity assays and live/dead assays, we demonstrated that the cerium oxide coating prevents bacterial adhesion, following a non-toxic mode of action. Only very low (milli/micromolar) concentrations of the substrates Br− and H2O2 are required for haloperoxidase-like reactions to occur. These concentrations are comparable to bromide concentrations found in animal and plant body fluids and to H2O2 concentrations produced in daylight.45 This makes CeO2 nanorods a potent and “green” catalyst for antibacterial applications33 since no chemicals are released into the environment other than those that occur anyway in natural metabolic processes of plants. Evidence for quorum quenching and therefore a non-biocidal mode of action was provided by analysis of the QS-dependent virulence factor pyocyanin. Microbial biofilms are ubiquitous on domestic, industrial, and other surfaces. Conventional biocides (strong oxidants, antibiotics, etc.) are increasingly problematic due to their lack of efficacy, non-targeted environmental effects, or development of resistance by microorganisms.
Experimental section
Materials and synthesis
Cerium(III) nitrate hexahydrate, nitrilotriacetic acid (99%), and sodium nitrilotriacetate (99+%) were purchased from Acros Organics. Hydrogen peroxide (35%) was purchased from Carl Roth. Ammonium hydroxide solution (25%) was obtained from VWR. All chemicals were used without further purification. Ultrapure water (18.2 MΩ cm−1) was used for all experiments. Polycarbonate (1 mm thickness, 15 × 15 cm plates) was obtained from GoodFellow.
Synthesis of cerium dioxide nanorods.
The synthesis was adapted from ref. 56 and modified for upscaling to multigram amounts of monodisperse CeO2 nanorods. In two centrifuge tubes, a total 10 g of Ce(NO3)3·6H2O were dissolved in 80 mL of H2O. Subsequently, 8 mL of NH4OH solution was added. After gentle shaking, the Ce(OH)3 precipitate was centrifuged (3000 rmp, 10 min) to remove excess water. The colorless powder was redispersed in 500 mL of water, and preheated to 60 °C under magnetic and Ultra-Turrax® stirring (15
000 rpm with S 25 EC-T-C-18G ST homogenizer). After the suspension had turned homogenous, 1.4 g NTA and 0.7 g Na3NTA were added. After further 15 min of continuous stirring, the grey-white suspension turned red after slowly adding 3 mL of H2O2 (35%). The temperature was slowly increased to 72 °C under ultra-Turrax®-stirring for 45 min. The reaction was allowed to cool to room temperature under magnetic stirring. To remove NH4NO3, the suspension was washed three times with H2O (9000 rmp, 20 min).
Phenol red assay.
The intrinsic haloperoxidase activity was determined using the phenol red assay. In this assay, tetrabromophenol blue (Br4PR) was formed via the reaction of phenol red (PR) in the presence of a bromide source (KBr) and H2O2 in the presence of an active catalyst (CeO2 nanorods). The reaction was monitored using an Agilent Cary 3500 spectrophotometer in the wavelength range from 350 to 700 nm at 25 °C. The baseline was measured at a constant temperature in a stirred (800 rpm) mixture of CeO2 nanorods (36.3 μmol), KBr (50 μmol), and PR (0.1 μmol) in 2 mL ultrapure water (18.2 MΩ cm−1) before the addition of H2O2 (6 μmol).
Lactone bromination.
The degradation of the quorum sensing molecule 3-oxo-C12-HSL by oxidative bromination was followed by time-dependent reverse high performance liquid chromatography coupled with electrospray ionisation-mass spectrometry. Several reaction mixtures (1 mL total volume) containing 900 μM 3-Oxo-HL, 20 mM KBr, 20 mM H2O2, and CeO2 nanoparticles (200 μg mL−1) were analyzed over a period of 5 h. The reactions were terminated by removing the catalyst by centrifugation and the supernatant was analyzed by mass spectrometry.
Michaelis–Menten kinetics.
For the Michaelis–Menten kinetics, the amount of H2O2 was varied. The kinetics was performed at λ = 592 nm, the absorbance maximum of Br4PR. For each measurement, the same stock solution of PR (50 μM) mixed with KBr (25 mM) was used. After the addition of 62.5 μL of nanoparticle suspension (1 mg mL−1), the reaction was stirred (800 rpm) for 2 min for temperature equilibration (25 °C). Prior to H2O2 addition, the absorbance was set to zero. The kinetics were measured for 10 min. All data points were linearly fitted in the period of 2–8 min. The concentration was calculated using the Lambert–Beer law. The extinction coefficient was determined by measuring the absorbance of five different Br4PR solutions (2 μM, 6 μM, 10 μM, 14 μM, 18 μM) in a quartz cuvette (d = 1 cm). Using the Lambert–Beer law, an extinction coefficient of 32
740 L mol−1 cm−1 was determined for BR4PR (free acid) in ultra-pure water with an Agilent Cary 3500 UV-VIS spectrophotometer.
CeO2 coating.
Polycarbonate plates (1 mm thickness) with a size of 1 × 1 cm were washed with MQ water and ethanol to remove the surface contamination. To activate the surface, the plates were treated in oxygen plasma for 20 min (290 W, 0.1 mbar). The plasma-treated polycarbonate plates were dip-coated in a CeO2 dispersion (9.5 ± 0.1 mg mL−1) in MQ water with a dipping speed of 60 mm min−1, held for 60 s in the dispersion, and pulled out with the same speed. The CeO2-coated polycarbonate plate was dried in air at 110 °C for 16 h. Finally, the plates were washed with MQ water to remove excess CeO2 from the surface.
Bacterial strains and growth conditions.
P. aeruginosa PA14 was obtained from the lab of Dr Max Schobert (Technische Universität Braunschweig, Germany). P. aeruginosa was aerobically grown in LB medium [1% (w/v) NaCl; 1% (w/v) tryptone; 0.5% (w/v) yeast extract] at 30 °C. For the preparation of agar plates, 1.5% (w/v) agar was added to the medium.
Biofilm assays.
For the quantification of bacterial biofilm production, a modified method of previously published protocols was used.57 Briefly, P. aeruginosa was aerobically grown in LB medium overnight at 30 °C. The cultures were then diluted in LB in a volume of 1 mL per well of a 24-well polystyrene microtiter plate (Sarstedt, Nürnbrecht) at a final Optical Density at 600 nm (OD600) of 0.5. In addition, KBr (Roth, Karlsruhe, Germany) and H2O2 (Roth, Karlsruhe, Germany) at a final concentration of 32 mM and 0.8 mM, respectively, were added to the wells. Then, the blank plates and CeO2/polycarbonate composites were subjected. Subsequently, the microtiter plate was incubated for 72 h under gentle shaking (150 rpm) at 30 °C. H2O2 was added stepwise at a final concentration of 0.8 mM every 24 h. Then, the plates were rinsed with water to remove the planktonic cells. After drying for 5 min, 1 mL of 1% (w/v) crystal violet (Merck, Darmstadt) was added to the wells containing the plates. After 30 min incubation at room temperature, unbound crystal violet was removed by gently submerging the plates two times in water. The plate was then air-dried overnight at room temperature. For quantification, 1 mL of 30% (v/v) acetic acid (Roth, Karlsruhe) was added to the plates to solubilize the crystal violet from the biofilm. After 15 min of incubation at room temperature, the absorbance was quantified in a plate reader (Tecan, Salzburg) at 575 nm.
Fluorescence quantification of bacterial biofilm.
P. aeruginosa was cultivated in the LB medium overnight at 30 °C. The cultures were then diluted in LB, in a volume of 1 mL per well of a 24-well polystyrene microtiter plate (Sarstedt, Nürnbrecht) at a final OD600 of 0.5. In addition, KBr (Roth, Karlsruhe, Germany) and H2O2 (Roth, Karlsruhe, Germany) at a final concentration of 32 mM and 0.8 mM, respectively, were added to the wells. Next, the non-coated plates and CeO2/polycarbonate slides were added to the wells. The microtiter plate was incubated for 72 h under gentle shaking (150 rpm) at 30 °C. H2O2 was added stepwise at a final concentration of 0.8 mM every 24 h. Then, the plates were rinsed with water to remove the planktonic cells. Subsequently, the plates were placed in 1 mL of a combined SYTO 9 and propidium iodide solution (Thermo Fisher, Pittsburgh, USA) and incubated for 30 min at 30 °C. Then, the plates were rinsed again with water and placed inside the well of a 24-well polystyrene microtiter plate. Finally, biofilm fluorescence was then quantified in a plate reader (Tecan, Salzburg) using a wavelength of 485 nm and 535 nm for the fluorophores SYTO 9 and PI, respectively.
Confocal laser scanning microscopy.
P. aeruginosa was cultivated in the LB medium overnight at 30 °C. The cultures were then diluted in LB in a volume of 1 mL per well of a 24-well polystyrene microtiter plate (Sarstedt, Nürnbrecht) at a final OD600 of 0.5. In addition, KBr (Roth, Karlsruhe, Germany) and H2O2 (Roth, Karlsruhe, Germany) at a final concentration of 32 mM and 0.8 mM, respectively, were added to the wells. The non-coated and CeO2-coated plates were then added to the wells. The microtiter plate was then incubated for 72 h under gentle shaking (150 rpm) at 30 °C. Every 24 h, H2O2 was added at a final concentration of 0.8 mM. The plates were then rinsed with water to remove the planktonic cells. Afterward, the plates were placed in 1 mL of a combined SYTO 9 and propidium iodide 1
:
1000 dilution (Thermo Fisher, Pittsburgh, USA) and incubated for 30 min at 30 °C. The plates were then again rinsed with water and put on microscopy cover slides. The samples were examined on an Axio Imager 2 (Carl Zeiss, Jena, Germany). The fluorophore SYTO 9 was visualized with an excitation of 470 nm, while propidium iodide was visualized with an excitation of 558 nm. The images and the biofilm thickness were then processed and quantified using the ZEN 3.3 blue software.
Pyocyanin analyses.
For pyocyanin production, an alternate method of already published protocols was used.58 For this purpose, P. aeruginosa was cultivated in the LB medium overnight at 30 °C. The cultures were then diluted in LB in a volume of 1 mL per well of a 24-well polystyrene microtiter plate (Sarstedt, Nürnbrecht) at a final OD600 of 0.5. In addition, KBr (Roth, Karlsruhe, Germany) and H2O2 (Roth, Karlsruhe, Germany) at a final concentration of 32 mM and 0.8 mM, respectively, were added to the wells. The non-coated plates and CeO2/polycarbonate slides were then added to the wells. The microtiter plate was then incubated for 72 h under shaking conditions (350 rpm) at 37 °C. Every 24 h, H2O2 was added stepwise at a final concentration of 0.8 mM. Afterward, the composites were removed from the wells and the remaining supernatant was collected in a microreaction tube (Eppendorf, Germany). The clls were separated from the culture fluids via centrifugation at 16 × 1000g for 15 min. Then, the supernatant was passed through 0.22 μm filters (Merck, Darmstadt). Finally, the cell-free culture fluids were analyzed for absorption at 695 nm and therefore quantified in a plate reader (Tecan, Salzburg) for pyocyanin.
Cell culture.
RAW 264.7 cells and human cervix carcinoma cells (HeLa) were cultured in Dulbecco's modified Eagle's medium (DMEM) supplemented with 10% FBS, 100 U mL−1 penicillin, 100 mg mL−1 streptomycin, and 2 mM glutamine. Cells were grown in a humidified incubator at 37 °C and 5% CO2. Cell passaging and harvesting were performed using 0.25% Trypsin-EDTA for 5 min at 37 °C, 5% CO2 (all reagents were from Thermo Fisher, Germany). Cell viability and cell count were determined using trypan blue and measuring by an automated cell counter (TC10, Bio-Rad, Germany).
Cell viability.
Cell viability was determined using CellTiter-Glo® Luminescent Assay according to the manufacturer's manual (Promega, USA). After cell harvesting, HeLa or RAW264.7 cells were seeded in 96-well plates (item no.: 655083, Greiner BioOne, Austria) with a cell number of 5000 cells per well. Cells were incubated overnight at 37 °C and 5% CO2 to enable cell detachment and afterward the medium was removed. Nanoparticle solutions were prepared in FBS-supplemented DMEM and added to the cells. After incubation for either 2 h or 24 h, the same volume of the CellTiter-Glo® Reagent was added and the luminescent signal was measured with an Inifinite M1000 plate reader (Tecan, Switzerland). Cell viability was displayed as the percentage of cell viability compared to the untreated cells.
Characterization
Electron microscopy.
The samples for transmission electron microscopy (TEM) were prepared by placing a drop of dilute NP dispersion in ethanol on a carbon-coated copper grid. The TEM images were obtained with a FEI Tecnai 12 TWIN LaB6 at 120 kV together with a Gatan US1000 CCD-camera (16-bit, 2048 × 2048 pixels) using the Gatan Digital Micrograph software.
Samples for scanning electron microscopy (SEM) were prepared with carbon film pads on aluminum holders. A silicon wafer was used for the sample preparation and fixed on the carbon pad. A polycarbonate plate was fixed on the carbon pad and sputtered with gold (thickness 1 nm). The SEM images were obtained using an FEI Nova NanoSEM 630 equipped with an EDAX-Pegasus X4 M unit for EDX measurements. The acceleration voltage was set to 5 kV. The elemental composition was calculated using the EDAX Genesis Software Suite.
Powder X-ray diffraction.
X-ray diffraction patterns were recorded on a STOE Stadi P diffractometer equipped with a Dectris My-then 1k detector in transmission mode using Mo Kα1 radiation. Crystalline phases were identified according to the PDF-2 database using the Bruker AXS EVA 10.0 software.
Zeta potentials.
Zetapotentials and hydrodynamic radii were measured on a Malvern Zetasizer Nano using disposable capillary cells (DTS1070) and single-use polystyrene cuvettes. Data analysis was performed with a Malvern Zetasizer Software 8.01.4906.
BET surface area.
BET measurements were conducted with a 3P Micro 300 gas adsorption instrument using nitrogen as the analysis gas at 77.4 K. The software 3P Surface Area & Pore Size Analyzer System 10.03.02 was used to analyze the recorded data.
Inductively coupled plasma mass spectrometry (ICPMS).
To determine the ceria content of the coated polycarbonate plates, the plates were dissolved in CHCl3, then dried at 45 °C, and incinerated at 600 °C. The ash was dissolved with sub-boiled HNO3 (65%), diluted with ultra-pure water, and measured with iCAP™ RQ ICP-MS (Thermo Scientific™).
Atomic force microscopy.
AFM measurements were conducted with an Park Systems NX10, using True Non-Contact™ mode and tapping mode. OMCL-AC160TS cantilever (k = 26 N m−1, f ≈ 300 kHz) were used to image polycarbonate substrates and CeO2 coating. For bacterial imaging, SD-R30-FM cantilevers (k = 2.8 N m−1, f ≈ 75 kHz) were applied. The Park Systems XEI Software was used for analysis. All measurements were conducted at ambient conditions.
UV/Vis.
UV/Vis measurements were conducted with an Agilent Cary 3500.
Plasma treatment.
The plates were treated with oxygen plasma in a Plasmaprozessor 200G from TePla Technics Plasma GmbH at 0.1 mbar and 290 W for 20 min.
Contact angle measurements.
The contact angle was measured with the contact angle measurement system OCA35 and the water drop was deposited by a Hamilton syringe (100 μL) with a hydrophobic needle.
Author contributions
O. J. carried out surface coatings and writing of original draft, F. P. carried out synthesis and writing of original draft, A. G. performed biofilm assays and contributed to original draft, J. O. and V. M. performed cytotoxicity tests, M. L. carried out electron microscopy, M. v. d. A and B. M. carried out ICP/MS analysis, A. K. performed AFM analysis, A. K., R. H. and WT conceived and designed research and revised the manuscript. All authors have given approval to the final version of the manuscript.
Conflicts of interest
The authors declare no competing financial interests.
Acknowledgements
This research was funded by a grant from the Deutsche Forschungsgemeinschaft (DFG) to W. T. (grant number TR 210/38-1) and the Bundesministerium für Bildung und Forschung (BMBF) Neue antimikrobielle Wirkstoffe gegen Biofilme (project number 031B0583A) to R. H. and Entwicklung innovativer biozider Nanopartikel zur Anwendung in der Kunststofftechnik (project number 19585N) to W. T. We thank S. Berinskat and F. Ludwig for X-ray diffraction measurements and Prof. A. Möller for access to the diffractometer. We are indebted to Prof. Rudolf Zentel (Department Chemie (JGU Mainz)) for the access to the Zetasizer. Thanks also apply to Prof. Hans-Jürgen Butt (MPI for Polymer Research Mainz) for providing the contact angle measurement system and the plasma cleaner. The microscopy equipment is operated by the Electron Microscopy Center (EMZM). Confocal laser scanning microscopy was performed at the Institute for Biotechnology and Drug Research (IBWF) Mainz.
References
- U. Schulz, Kunststoffoptiken mit Antireflex- und Antibeschlageigenschaften durch Plasmaätzen und Beschichtung, Vak. Forsch. Prax., 2014, 26, 26–31, DOI:10.1002/vipr.200800354.
- W. Sun, W. Liu, Z. Wu and H. Chen, Chemical Surface Modification of Polymeric Biomaterials for Biomedical Applications, Macromol. Rapid Commun., 2020, 41, e1900430, DOI:10.1002/marc.201900430.
- F. Theinkom, L. Singer, F. Cieplik, S. Cantzler, H. Weilemann, M. Cantzler, K. A. Hiller, T. Maisch and J. L. Zimmermann, Antibacterial efficacy of cold atmospheric plasma against Enterococcus faecalis planktonic cultures and biofilms in vitro, PLoS One, 2019, 14, e0223925, DOI:10.1371/journal.pone.0223925.
- T. Suryaprabha and M. G. Sethuraman, Fabrication of copper-based superhydrophobic self-cleaning antibacterial coating over cotton fabric, Cellulose, 2017, 24, 395–407, DOI:10.1007/s10570-016-1110-z.
- D. Druvari, N. D. Koromilas, V. Bekiari, G. Bokias and J. K. Kallitsis, Polymeric Antimicrobial Coatings Based on Quaternary Ammonium Compounds, Coatings, 2018, 8, 8, DOI:10.3390/coatings8010008.
- A. Nastulyavichus, S. Kudryashov, N. Smirnov, I. Saraeva, A. Rudenko, E. Tolordava, A. Ionin, Y. Romanova and D. Zayarny, Antibacterial coatings of Se and Si nanoparticles, Appl. Surf. Sci., 2019, 469, 220–225, DOI:10.1016/j.apsusc.2018.11.011.
- B. S. Necula, I. Apachitei, F. D. Tichelaar, L. E. Fratila-Apachitei and J. Duszczyk, An electron microscopical study on the growth of TiO2-Ag antibacterial coatings on Ti6Al7Nb biomedical alloy, Acta Biomater., 2011, 7, 2751–2757, DOI:10.1016/j.actbio.2011.02.037.
- R. André, F. Natalio, M. N. Tahir and W. Tremel, Self-cleaning antimicrobial surfaces by bio-enabled growth of SnO2 coatings on glass, Nanoscale, 2013, 5, 3447–3456 RSC.
- “Toxic lobbying: the titanium dioxide label debate continues”, can be found under https://corporateeurope.org/en/2019/06/toxic-lobbying-titanium-dioxide-label-debate-continues, 2019.
-
Y. C. Ching, T. M. S. U. Gunathilake, K. Y. Ching, C. H. Chuah, V. Sandu, R. Singh and N.-S. Fiou, in Durab. Life Predict. Biocomposites, Fibre-Reinforced Compos. Hybrid Compos, ed. M. Jawaid, M. Thariq and N. Saba, Woodhead Publishing, 2019, pp. 407–426 Search PubMed.
- J. Zhang, W. Guo, Q. Li, Z. Wang and S. Liu, The effects and the potential mechanism of environmental transformation of metal nanoparticles on their toxicity in organisms, Environ. Sci. Nano, 2018, 5, 2482–2499, 10.1039/C8EN00688A.
- S. Krishnan, C. J. Weinman and C. K. Ober, Advances in polymers for anti-biofouling surfaces, J. Mater. Chem., 2008, 18, 3405–3413, 10.1039/B801491D.
- W. Yang, H. S. Sundaram, J. R. Ella, N. He and S. Jiang, Low fouling electrospun PLLA films modified with zwitterionic poly(sulfobetaine methacrylate)-catechol conjugates, Acta Biomater., 2016, 40, 92–99, DOI:10.1016/j.actbio.2016.05.035.
- S. Ozcan, P. Kaner, D. Thomas, P. Cebe and A. Asatekin, Hydrophobic Antifouling Electrospun Mats from Zwitterionic Amphiphilic Copolymers, ACS Appl. Mater. Interfaces, 2018, 10, 18300–18309, DOI:10.1021/acsami.8b03268.
- A. Borghesi and M. Stronati, Strategies for the prevention of hospital-acquired infections in the neonatal intensive care unit, J. Hosp. Infect., 2008, 68, 293–300, DOI:10.1016/j.jhin.2008.01.011.
- S. Mathur and R. Singh, Antibiotic resistance in food lactic acid bacteria–a review, Int. J. Food Microbiol., 2005, 105, 281–295, DOI:10.1016/j.ijfoodmicro.2005.03.008.
- K. Page, M. Wilson and I. P. Parkin, Antimicrobial surfaces and their potential in reducing the role of the inanimate environment in the incidence
of hospital-acquired infections, J. Mater. Chem., 2009, 19, 3818–3831, 10.1039/B818698G.
- J. B. Kristensen, R. L. Meyer, B. S. Laursen, S. Shipovskov, F. Besenbacher and C. H. Poulsen, Antifouling enzymes and the biochemistry of marine settlement, Biotechnol. Adv., 2008, 26, 471–481, DOI:10.1016/j.biotechadv.2008.05.005.
- M. Sandy, J. N. Carter-Franklin, J. D. Martin and A. Butler, Vanadiumbromoperoxidase from Delisea pulchra: enzyme-catalyzed formation of bromofuranone and attendant disruption of quorum sensing, Chem. Commun., 2011, 47, 12086–12088, 10.1039/C1CC15605E.
- M. B. Miller and B. L. Bassler, Quorum sensing in bacteria, Annu. Rev. Microbiol., 2001, 55, 165–199 CrossRef CAS PubMed
https://pubmed.ncbi.nlm.nih.gov/11544353/..
- K. Herget, P. Hubach, S. Pusch, P. Deglmann, H. Götz, T. E. Gorelik, I. A. Gural'skiy, F. Pfitzner, T. Link, S. Schenk, M. Panthöfer, V. Ksenofontov, U. Kolb, T. Opatz, R. André and W. Tremel, Haloperoxidase Mimicry by CeO2−x Nanorods Combats Biofouling, Adv. Mater., 2017, 29, 1603823, DOI:10.1002/adma.201603823.
- B. Sels, D. De Vos, M. Buntinx, F. Plerard, A. Kirsch-De Mesmaeker and P. Jacobs, Layered double hydroxides exchanged with tungstate as biomimetic catalysts for mild oxidative bromination, Nature, 1999, 400, 855–857, DOI:10.1038/23674.
- V. Conte and B. Floris, Vanadium and molybdenum peroxides: synthesis and catalytic activity in oxidation reactions, Dalton Trans., 2011, 40, 1419–1436, 10.1039/C0DT00706D.
- R. Wever and M. A. van der Horst, The role of vanadium haloperoxidases in the formation of volatile brominated compounds and their impact on the environment, Dalton Trans., 2013, 42, 11778–11786, 10.1039/C3DT50525A.
- F. Natalio, R. André, A. F. Hartog, B. Stoll, K. P. Jochum, R. Wever and W. Tremel, Vanadium pentoxide nanoparticles mimic vanadium haloperoxidases and thwart biofilm formation, Nat. Nanotechnol., 2012, 7, 530–535, DOI:10.1038/nnano.2012.91.
- L. Wang, J. Hou, S. Liu, A. J. Carrier, T. Guo, Q. Liang, D. Oakley and X. Zhang, CuO nanoparticles as haloperoxidase mimics: Chloride-accelerated heterogeneous Cu-Fenton chemistry for H2O2 and glucose sensing, Sens. Actuators, B, 2019, 287, 180–184, DOI:10.1016/j.snb.2019.02.030.
- A. Filippi, F. Liu, J. Wilson, S. Lelieveld, K. Korschelt, T. Wang, T. Reich, Y. Wang, U. Pöschl, W. Tremel and H. Tong, Antioxidant activity of cerium dioxide nanoparticles and nanorods in scavenging hydroxyl radicals, RSC Adv., 2019, 9, 11077–11081, 10.1039/C9RA00642G.
- H. Wei and E. Wang, Nanomaterials with enzyme-like characteristics (nanozymes): next-generation artificial enzymes, Chem. Soc. Rev., 2013, 42, 6060–6093, 10.1039/C3CS35486E.
- K. Korschelt, M. N. Tahir and W. Tremel, A Step into the Future: Applications of Nanoparticle Enzyme Mimics, Chem. – Eur. J., 2018, 24, 9703–9713, DOI:10.1002/chem.201800384.
- C. K. Kim, T. Kim, I.-Y. Choi, M. Soh, D. Kim, Y.-J. Kim, H. Jang, H.-S. Yang, J. Y. Kim, K.-K. Park, S. P. Park, S. Park, T. Yu, B. W. Yoon, S.-H. Lee and T. Hyeon, Ceria Nanoparticles that can Protect against Ischemic Stroke, Angew. Chem., Int. Ed., 2012, 51, 11039–11043, DOI:10.1002/anie.201203780.
- T. V. Plakhova, A. Y. Romanchuk, S. N. Yakunin, T. Dumas, S. Demir, S. Wang, S. G. Minasian, D. K. Shuh, T. Tyliszczak, A. A. Shiryaev, A. V. Egorov, V. K. Ivanov and S. N. Kalmykov, Solubility of Nanocrystalline Cerium Dioxide: Experimental Data and Thermodynamic Modeling, J. Phys. Chem. C, 2016, 120, 22615–22626, DOI:10.1021/acs.jpcc.6b05650.
-
N. N. Greenwood and A. Earnshaw, Chemistry of the Elements, Elsevier Science, 2nd edn, 2012, p. 1238 Search PubMed.
- K. Herget, H. Frerichs, F. Pfitzner, M. N. Tahir and W. Tremel, Functional Enzyme Mimics for Oxidative Halogenation Reactions that Combat Biofilm Formation, Adv. Mater., 2018, 30, 1707073, DOI:10.1002/adma.201707073.
- A. Qureshi, S. Shah, S. Pelagade, N. L. Singh, S. Mukherjee, A. Tripathi, U. P. Despande and T. Shripathi, Surface modification of polycarbonate by plasma treatment, J. Phys.: Conf. Ser., 2010, 208, 012108, DOI:10.1088/1742-6596/208/1/012108.
- H. Yaghoubi, N. Taghavinia and E. K. Alamdari, Self cleaning TiO2 coating on polycarbonate: Surface treatment, photocatalytic and nanomechanical properties, Surf. Coat. Technol., 2010, 204, 1562–1568, DOI:10.1016/j.surfcoat.2009.09.085.
- R. M. Thurston, J. D. Clay and M. D. Schulte, Effect of Atmospheric Plasma Treatment On Polymer Surface Energy and Adhesion, J. Plast. Film Sheeting, 2007, 23, 63–78, DOI:10.1177/8756087907078698.
- M. Lorenzetti, I. Dogša, T. Stošicki, D. Stopar, M. Kalin, S. Kobe and S. Novak, The Influence of Surface Modification on Bacterial Adhesion toTitanium-Based Substrates, ACS Appl. Mater. Interfaces, 2015, 7, 1644–1651, DOI:10.1021/am507148n.
- G. Balakrishnan, S. Tripura Sundari, P. Kuppusamia, P. Chandra Mohan, M. P. Srinivasan, E. Mohandas, V. Ganesan and D. Sastikumar, A study of microstructural and optical properties of nanocrystalline ceria thin films prepared by pulsed laser deposition, Thin Solid Films, 2011, 519, 2520–2526, DOI:10.1016/j.tsf.2010.12.013.
- H. A. Miran, Z.-T. Jiang, M. Altarawneh, J.-P. Veder, Z.-F. Zhou, M. M. Rahman, Z. N. Jaf and B. Z. Dlugogorski, Influence of DC magnetron sputtering reaction gas on structural and optical characteristics of Ce-oxide thin films, Ceram. Int., 2018, 44, 16450–16458, DOI:10.1016/j.ceramint.2018.06.059.
- I. Z. Dubé, J. M. García Rangel, J. Roque, I. C. Romero-Ibarra and M. F. García Sánchez, Strong texture tuning along different crystalline directions in glass-supported CeO2 thin films by ultrasonic spray pyrolysis, Sci. Rep., 2021, 11, 2006, DOI:10.1038/s41598-021-81353-x.
- R. Lo Nigro, G. Malandrino and I. L. Fragalà, Metal–Organic Chemical Vapor Deposition of CeO2〈100〉 Oriented Films on No-Rolled Hastelloy C276, Chem. Mater., 2001, 13(12), 4402–4404, DOI:10.1021/cm011232f.
- M. Dronova, V. Lair, P. Vermaut, A. Ringuedé and V. An, Study of ceria thin films prepared via electrochemical deposition: Role of selected electrochemical parameters on growth kinetics, Thin Solid Films, 2020, 693, 137674, DOI:10.1016/j.tsf.2019.137674.
- X. Yuan, P. S. Nico, X. Huang, T. Liu, C. Ulrich, K. H. Williams and J. A. Davis, Production of Hydrogen Peroxide in Groundwater at Rifle, Colorado, Environ. Sci. Technol., 2017, 51, 7881–7891, DOI:10.1021/acs.est.6b04803.
- H. Sakugawa, I. R. Kaplan, W. Tsai and Y. Cohen, Atmospheric hydrogen peroxide, Environ. Sci. Technol., 1990, 24, 1452–1462, DOI:10.1021/es00080a002.
- W. J. Cooper, R. G. Zlka, R. G. Petasne and J. M. C. Plane, Photochemical Formation of H2O2 in Natural Waters Exposed to Sunlight, Environ. Sci. Technol., 1988, 22, 1156–1160, DOI:10.1021/es00175a004.
- E. J. Salazar-Sandoval, M. K. G. Johansson and A. Ahniyaz, Aminopolycarboxylic acids as a versatile tool to stabilize ceria nanoparticles – a fundamental model experimentally demonstrated, RSC Adv., 2014, 4, 9048–9055, 10.1039/C3RA45875J.
- S. Goutelle, M. Maurin, F. Rougier, X. Barbaut, L. Bourguignon, M. Ducher and P. Maire, The Hill equation: a review of its capabilities in pharmacological modelling, Fundam. Clin. Pharmacol., 2008, 22, 633–648, DOI:10.1111/j.1472-8206.2008.00633.x.
- S. Kozuch and J. M. L. Martin, “Turning Over” Definitions in Catalytic Cycles, ACS Catal., 2012, 2, 2787–2794, DOI:10.1021/cs3005264.
- A. Hofrichter, P. Bulkin and B. Drévillon, Plasma treatment of polycarbonate for improved adhesion, J. Vac. Sci. Technol., A, 2002, 20, 245, DOI:10.1116/1.1430425.
- U. Schubert, Polymers Reinforced by Covalently Bonded Inorganic Clusters, Chem. Mater., 2001, 13, 3487–3494, DOI:10.1021/cm001258r.
- U. Schubert and N. Materialien, Wo sich Materialwissenschaften und Chemie treffen, Chem. Unserer Zeit, 2021, 55, 14–24, DOI:10.1002/ciuz.202000001.
- J. Dagata, P. Kavuri, A. Vladar, K. Ehara, N. Farkas, C.-L. Wu and H. Itoh, Method for measuring the diameter of polystyrene latex reference spheres by atomic force microscopy, Natl. Inst. Stand. Technol. Spec. Publ. SP, 260–185, 2016, 1–26, DOI:10.6028/NIST.SP.260-185.
- C. J. Boehlert, J. D. Farr, R. K. Schulze, R. A. Pereyra and J. A. Archuleta, Initial electron back-scattered diffraction observations of cerium, Philos. Mag., 2003, 83, 1735–1744, DOI:10.1080/1478643031000080708.
- M. Exner, W. Nissing, K. Behringer, S. Engelhart, S. Pleischl, C. Koch, M. Trautmann, A. Kramer, P. Walger, H. Martiny and L. Jatzwauk, Bedeutung von Wasser und gramnegativen Keimen in der Hygiene, Hyg. Med., 2016, 41(Suppl. 2), 3–32 Search PubMed.
- Y. Deng, L. Wang, Y. Chen and Y. Long, Optimization of staining with SYTO 9/propidium iodide: interplay, kinetics and impact on Brevibacillus brevis, BioTechniques, 2020, 69, 89–98, DOI:10.2144/btn-2020-0036.
- W. J. Cooper, R. G. Zika, R. G. Petasne and J. M. C. Plane, Photochemical formation of hydrogen peroxide in natural waters exposed to sunlight, Environ. Sci. Technol., 1988, 22, 1156–1160, DOI:10.1021/es00175a004; M. Aguirre, E. Johansson Salazar-Sandoval, M. Johansson, A. Ahniyaz, M. Paulis and J. R. Leiza, Hybrid acrylic/CeO2 nanocomposites using hydrophilic, spherical and high aspect ratio CeO2 nanoparticles, J. Mater. Chem. A, 2014, 2, 20280–20287, 10.1039/C4TA03620D.
- G. A. O'Toole, Microtiter dish biofilm formation assay, J. Visualized Exp., 2010, 10, 2437, DOI:10.3791/2437.
- C. T. O'Loughlin, L. C. Miller, A. Siryaporn, K. Drescher, M. F. Semmelhack and B. L. Bassler, A quorum-sensing inhibitor blocks Pseudomonas aeruginosa virulence and biofilm formation, Proc. Natl. Acad. Sci. U. S. A., 2013, 110, 17981–17986, DOI:10.1073/pnas.1316981110.
Footnotes |
† Electronic supplementary information (ESI) available: Fig. S1 demonstrates the scanning electron microscopy (SEM) image (A) and energy-despersive X-ray (EDX) spectrum (B) of the CeO2-coated surface of polycarbonate. This is followed by information about the uncoated and CeO2-coated polycarbonate surface investigated by AFM (Fig. S2–4). The uncoated and CeO2-coated PC surface with P. aeruginosa were also examined by AFM (Fig. S5+6). Fig. S7 demonstrates the microscope images of HeLa and RAW cells on the surface of CeO2-coated polycarbonate. In conclusion, an image of aqueous CeO2-suspersion is shown in Fig. S8. Fig. S9 shows the UV/Vis spectra of uncoated, plasma-treated, and CeO2-coated PC, showing no visual difference after coating. See DOI: 10.1039/d1nr03320d |
‡ These authors contributed equally to this work. |
|
This journal is © The Royal Society of Chemistry 2022 |