DOI:
10.1039/D1NJ04798A
(Paper)
New J. Chem., 2022,
46, 169-177
An aggregation-induced emission-active bis-heteroleptic ruthenium(II) complex of thiophenyl substituted phenanthroline for the selective “turn-off” detection of picric acid†
Received
7th October 2021
, Accepted 16th November 2021
First published on 17th November 2021
Abstract
The development of metal complex-based aggregation-induced emission (AIE) active materials is of great importance and a challenging task. We have designed and successfully synthesized a new bis-heteroleptic ruthenium(II) polypyridine complex, Ru-1, of a thiophenol substituted 1,10-phenanthroline based ligand (L1). The solution structure of Ru-1 was fully characterized by 1D (1H, 13C) and 2D (1H-1H COSY, 1H-13C HSQC, and 1H-13C HMBC) NMR spectroscopy, and ESI-MS. Ru-1 shows aggregation-induced emission (AIE) enhancement in water and highly dense polyethylene glycol (PEG) media supported by DLS, SEM, and TEM studies. The complex, Ru-1, is weakly luminescent in acetonitrile, and a very bright luminescence of Ru-1 in the weakly soluble water and highly dense PEG media arises due to the formation of nanoaggregates and the restricted intramolecular motion (RIM). The probe in aqueous acetonitrile solution (7
:
3; v/v) is able to selectively and sensitively detect picric acid (PA) over other competitive nitroaromatic compounds through luminescence quenching. The limit of detection (LOD) signifies that Ru-1 is capable of detecting picric acid at the micromolar level. Theoretical calculations were carried out to assign electronic transitions in Ru-1, both the ground and excited states. Furthermore, a paper strip kit with Ru-1 has been constructed for the practical application and in-field detection of picric acid without any interference of other nitroaromatic analytes.
Introduction
Many traditional organic fluorophores generally have a planar structure and emit strongly in solution but experience emission quenching upon aggregation in highly concentrated solution or in the solid state. This phenomenon, notoriously known as aggregation-caused quenching (ACQ), dramatically reduces their luminous efficiency.1–4 There is no doubt that the detrimental ACQ effect has limited the applications of traditional fluorophores as bio-/chemosensors. This ACQ effect forces researchers to construct chemo-/biosensors in a very dilute solution, which limits the sensitivity and signal-to-background ratio. Consequently, the aggregation-induced emission enhancement (AIE) phenomenon opened a new door for designing luminescence light-up probes, which Tang and co-workers first reported in 2001.5 Generally, AIE-active molecules, which are non-luminescent or poorly luminescent in a benign solvent, show turn-on luminescence upon aggregation.6–9 These systems are characterized by highly intense emission in an aggregated state due to the restriction of intramolecular motions (RIM) resulting in the suppression of the non-radiative decay pathways that enables their use in high concentrations or thinfilms.10–12 The RIM process is generally caused by supramolecular interactions such as hydrogen bonding, C–H⋯π interactions, halogen bonding, etc. The AIE (or AIEE) phenomenon has also attracted considerable research interest due to its potential applications in several fields, such as sensing, bioimaging, organic light-emitting diode (OLED) devices, and organic lasers.13–17 The AIE research field has been expanded by researchers primarily via developing organic-based AIEgens, and metal complexes of iridium, platinum, palladium, copper, zinc, and ruthenium.18–20 Among several metal complexes, iridium(III)-based AIEgens are mostly reported; however, bis-heteroleptic ruthenium(II) polypyridine complex-based AIE active probes are very rare.21,22
Highly selective and fast detection of nitroaromatic compounds (NACs) that are widely used in the battlefield are always important to save humanity from mass destruction and prevent terrorist threats that have been frequently seen recently around the world.23–27 Common nitroaromatic compounds such as 2,4,6-trinitrophenol (TNP), 2,4-dinitrophenol (DNP), and 2,4,6-trinitrotoluene (TNT) are well known primary constituents of much unexploded military ordnance that includes explosive munitions such as grenades, bombs, mortar shells, and landmines worldwide.28–30 In the family of nitroaromatic compounds (NACs), 2,4,6-trinitrophenol, also known as picric acid (PA), is an organic acid (pKa = 0.38), and like many other poly nitroaromatic compounds, it is a powerful explosive.31,32 Picric acid is familiar for its wide use as an explosive until World War I.33,34 It is a good electron acceptor, and upon initiation, it can undergo a highly exothermic reaction producing toxic gas. In addition, PA is a strong irritant to skin/eyes and causes prospective damage to organs involved in the respiratory system and liver when it comes in contact with the human body.35,36 Despite its dangerous behavior, picric acid is enormously used in organic synthesis, chemical laboratories, pharmaceuticals, and dye industries, along with rocket fuels and the manufacture of explosives.37–39 The unreasonable use or release of PA may not only result in severe environmental pollution and damage to human health but could also threaten public safety.40,41 Thus, there is increased interest in developing highly sensitive and effective methods for detecting picric acid.42–45
To date, some methods have been employed to detect PA, such as photometry, fluorescent chemosensors, ion-selective electrodes, and gas or liquid chromatography.46,47 Amongst these, fluorescent chemosensors that exploit sensitive fluorescence quenching by nitroaromatic derivatives have been investigated extensively in both solid and solution phases at low concentrations with high sensitivity and selectivity.48–50 In the past few years, several fluorescent probes were reported for detecting PA, such as metal–organic frameworks, quantum dots, polymer-based materials, and aggregation-induced emission-active molecules.51–53 Even though several π electron-rich fluorescent organic chemosensors, and organic polymers, have been employed to detect the presence of electron-deficient nitro-aromatics, the development of a highly selective and efficient AIE-active metal complex-based PA probe is still very rare.54,55
Since the past decade, ruthenium(II) polypyridyl complexes have been extensively studied as luminescent sensors/probes due to their excellent photophysical properties such as visible excitation, long emissive lifetimes, and large Stokes shifts.56,57 Moreover, most Ru(II) polypyridine complexes are highly soluble and stable in aqueous media at room temperature. Recently, a sizeable amount of Ru(II) complex-based sensitive and specific luminescence probes have been developed to detect cations, anions, and bio-relevant species.58–61
In continuation of our research on ruthenium(II) and iridium(III) complex-based sensors,62–65 herein, we describe the synthesis and characterization of a new AIE-active bis-heteroleptic ruthenium(II) complex, Ru-1, of thiophenyl substituted 1,10-phenanthroline based ligand and its aptness for highly sensitive and selective detection of picric acid. The complex shows aggregation-induced emission enhancement in water and highly dense PEG media (Scheme 1). Probe Ru-1 in aqueous acetonitrile media (7
:
3; v/v) shows bright luminescence, which is quenched by picric acid due to the efficient electron transfer from a Ru(II)-complex based donor to the electron-deficient picric acid (Scheme 1). A portable test paper strip with Ru-1 has been prepared for the in-field detection of picric acid without any interference of other nitroaromatic analytes.
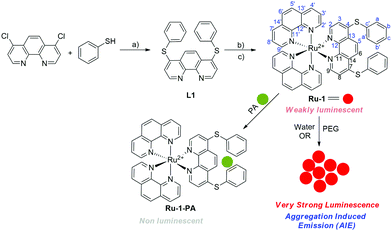 |
| Scheme 1 The synthetic procedure of ligand L1 and its corresponding complex Ru-1 and the schematic representation of picric acid (PA) detection and AIE by complex Ru-1. Reagents and conditions: (a) THF, stirring, 90 °C, 24 h; (b) cis-[Ru(phen)2Cl2], EtOH/H2O (2 : 1), stirring, 100 °C, N2; 7 h, and (c) excess KPF6. | |
Results and discussion
Synthesis and characterization
The ligand, 4,7-dichloro-1,10-phenanthroline and cis-[Ru(Phen)2Cl2], was synthesized according to the reported literature procedures.66,67 Ligand 4,7-bis(phenylthio)-1,10-phenanthroline (L1) was synthesized by refluxing the mixture of 4,7-dichloro-1,10-phenanthroline, sodium acetate, and thiophenol in a distilled THF solution and isolated in 52% yield (Scheme 2). L1 was fully characterized by 1H and 13C NMR spectroscopy, and electrospray ionization-mass spectrometry (ESI-MS) (Fig. S1–S3, ESI†). The bis-heteroleptic ruthenium(II) complex, Ru-1, was prepared by refluxing the mixture of L1 and cis-[Ru(Phen)2Cl2] in ethanol/water (2
:
1,v/v) under N2 for 12 h followed by the Cl− counterion exchange with PF6− (Scheme 1). Ru-1 was fully characterized by one-dimensional (1D) (1H, 13C) and two-dimensional (2D) (1H−1H COSY, 1H−13C HSQC, and 1H−13C HMBC) NMR spectroscopy, electrospray ionization mass spectrometry (ESI-MS) and elemental analysis (Fig. S4–S9, ESI†). The photophysical and electrochemical properties of Ru-1 were determined by UV-vis, photoluminescence (PL), and cyclic voltammetry (CV) methods. 1D and 2D NMR spectra of Ru-1 in acetone-d6 at room temperature show all expected resonances of 1,10-phenanthroline and 4,7-bis(phenylthio)-1,10-phenanthroline ligands. All characteristic proton signals in the 1H NMR spectrum have been assigned with the help of 1H−1H COSY NMR (Fig. 1a and Fig. S6, ESI†), and all the carbon signals of Ru-1 are assigned using HSQC and HMBC NMR spectra (Fig. 1b and Fig. S7, S8, ESI†). In ESI-MS, two characteristic peaks are found at m/z = 429.12 (calcd. 429.06) and m/z = 1003.00 (calcd. 1003.08), which can be assigned as doubly charged [C48H32N6S2Ru]2+ and singly charged [C48H32N6PF6S2Ru]+ species, respectively (Fig. S9, ESI†).
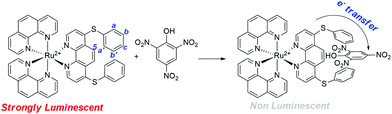 |
| Scheme 2 Plausible interaction between Ru-1 and picric acid. | |
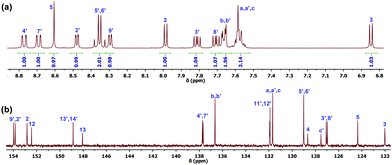 |
| Fig. 1
1H (a) and 13C NMR (b) spectra of Ru-1 in acetone-d6. | |
Electrochemical properties of Ru-1
The electrochemical properties of Ru-1 were examined by cyclic voltammetry (CV) using a Pt working electrode in dry acetonitrile under a N2 atmosphere with ferrocene (Fc) as a standard (Eox1/2 = + 0.400 V vs. Ag wire). Compound Ru-1 shows a single quasi-reversible (ΔEp = 80 mV) Ru2+/Ru3+ redox wave at Eox1/2 = +1.420 V (Epa = 1.460 V and Epc = 1.380 V) vs. Fc+/Fc (Fig. 2a). The redox wave is anodically shifted by 100 mV in comparison to [Ru(phen)3]2+ (Eox1/2 = +1.320 V vs. Fc+/Fc) (Fig. 2b) which supports the stronger π-acceptance of L1 than the phenanthroline ligand.
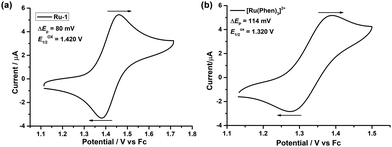 |
| Fig. 2 Cyclic voltammograms of (a) Ru-1 and (b) [Ru(phen)3](PF6)2, with 0.1 M Bu4NClO4 in dry and degassed CH3CN versus the Fc/Fc+ couple. | |
Photophysical and aggregation-induced emission (AIE) properties
The photophysical properties of Ru-1 were checked using UV–vis and photoluminescence (PL) spectroscopy. The UV-vis spectrum of Ru-1 shows bands at ∼225 nm (ε = 75
200 M−1 cm−1), ∼262 nm (ε = 84
400 M−1 cm−1), and ∼445 nm (ε = 30
200 M−1 cm−1) in acetonitrile at 25 °C (Fig. 3a). The bands at ∼225 nm and ∼262 nm can be assigned as an intraligand (IL) π–π* transition, whereas the band at ∼445 nm is due to the metal to ligand charge transfers (1MLCT): from the Ru(II) center to the phenanthroline unit. Excitation of Ru-1 at λex = 445 nm results in a broad weak luminescence centered at λem = ∼610 nm at 25 °C in CH3CN (Fig. 3a).
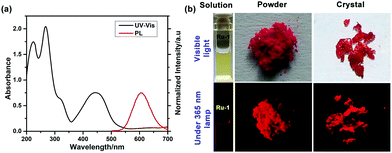 |
| Fig. 3 (a) Normalized absorbance and PL spectra of Ru-1 (25 μM) in dry acetonitrile. [λex = 445 nm; λem = ∼610 nm]. (b) Image of Ru-1 solution (CH3CN), crystal and powder in ambient light and under UV light (365 nm) irradiation. | |
Complex Ru-1 (25 μM) in dilute acetonitrile solution displays a weak red luminescence, whereas the solid power and crystalline Ru-1 show very bright luminescence under the illumination of a 365 nm UV light (Fig. 3b), which is the primary indication of aggregation-induced emission (AIE) enhancement behavior of complex Ru-1. To confirm, the luminescence behavior of Ru-1 was investigated using a binary solvent mixture. Complex Ru-1 is soluble in common organic solvents (good solvent), such as CH2Cl2, acetonitrile, and acetone, but sparingly soluble in water (poor solvent). Acetonitrile was chosen for the aggregation studies as it is miscible with water. The weak emission of Ru-1 (25 μM) at 610 nm is gradually enhanced and slightly red-shifted to 614 nm upon the incremental addition of H2O to the acetonitrile solution. The PL intensity of Ru-1 increases ∼3 fold in 90% water fraction (Fig. 4a). We assumed that the PL enhancement is due to the restriction of intramolecular motion (RIM) caused by the aggregation in a poor solvent. To verify the restriction of intramolecular motion (RIM) in Ru-1, the PL changes of Ru-1 were also monitored in CH3CN with increasing polyethylene glycol (PEG) (fPEG) fractions (0–90% CH3CN–PEG). The PL intensity of Ru-1 increases ∼10 times in the presence of 90% PEG in CH3CN, which further specifies the aggregation and AIE activity (Fig. 4b). The digital photograph also shows gradual enhancement of red luminescence at a higher fraction of PEG under 365 nm UV-light illumination (Fig. 4c). Moreover, to support the aggregate formation, UV–vis spectra of Ru-1 (25 μM) with an increasing amount of water (0–90%) were recorded. The absorption bands, as well as the baseline of UV–vis spectra of Ru-1 (25 μM), are uplifted, which confirms the light scattering by the nanoaggregates of compound Ru-1 (Fig. S10, ESI†). The origin of such an AIE phenomenon of Ru-1 can be explained similarly to those reported in previous literature.6–9 In the dilute solution, Ru-1 exists in a non-aggregated state and releases the excited energy from the 3MC excited state via a non-radiative relaxation pathway, resulting in a very weak luminescence in the isolated state (Vide infra). However, in the aggregated form, the weak intermolecular interactions between adjacent phenanthroline units restrict the intramolecular motions, which inhibit the non-radiative decay from the photoexcited triplet state and strengthen the PL of Ru-1.
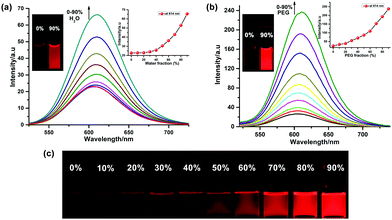 |
| Fig. 4 PL spectra of Ru-1 (25 μM) with different volume fractions (%) of (a) CH3CN–H2O and (b) CH3CN–PEG (λex = 445 nm and λem = 610 nm). (c) Photographs of the luminescence of Ru-1 in different PEG fractions (0–90%) under 365 nm UV illumination. | |
The aggregation of Ru-1 in the binary solvent mixture was supported by the DLS study. The hydrodynamic diameters of aggregates at fwater = 90% range from 100 to 400 nm with a Zaverage of 194.3 nm (Fig. 5a), whereas at fPEG = 90% they range from 150 to 800 nm, with a Zaverage of 385.9 nm (Fig. 5b). Furthermore, the morphological properties of aggregated Ru-1 (25 μM) were visualized in SEM and TEM in the water–CH3CN mixture (9
:
1). SEM and TEM images of Ru-1 (25 μM) clearly indicate the nanoaggregate formation (Fig. 5c and d). In SEM images, the diameter of spherical nanoaggregates ranges from ∼100–450 nm, while the TEM images show a nearly similar result with an average diameter of ∼75–300 nm.
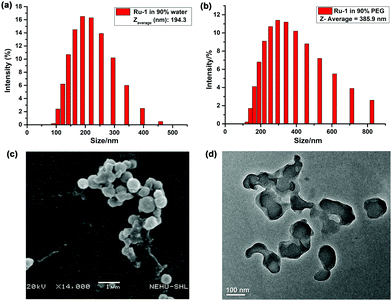 |
| Fig. 5 Particle size distribution of Ru-1 in (a) CH3CN–water (1 : 9), (b) CH3CN–PEG (1 : 9) mixture and (c) SEM and (d) TEM images in 90% water–CH3CN. | |
Picric acid sensing study
The aggregation-induced emission properties of Ru-1 were further utilized in sensing nitroaromatic explosives. Among various nitroaromatic explosives, 2,4,6-trinitrophenol (TNP), also known as picric acid (PA), has superior explosive power when compared to 2,4,6-trinitrotoluene (TNT). We carried out PL studies of Ru-1 in H2O/CH3CN (7
:
3, v/v) solution to investigate picric acid sensing. During the PL selectivity study, the emission band of Ru-1 centered at 612 nm remains almost unaffected upon the addition of 14 equiv. 4-nitrophenol (NP) and nitrobenzene (NB). The PL of Ru-1 is slightly quenched by other nitroaromatic compounds, such as 2,4-dinitrophenol (∼1.84 fold), 4-nitrobenzaldehyde (∼1.52 fold), and 4-nitroaniline (∼1.62 fold), to the Ru-1 solutions (Fig. 6a). However, only in the presence of PA, ∼11 fold PL intensity quenching of Ru-1 is registered, which implies Ru-1 as a highly selective sensor for PA. The PL titration of Ru-1 with picric acid reveals that the PL intensity is gradually decreased at 612 nm (λex = 445 nm) upon the addition of 0–14 equiv. of picric acid (Fig. 6b). As shown in the inset of Fig. 6a, the “turn-off” luminescence signaling behavior of Ru-1 is observed by the naked eye in the presence of PA under UV light illumination (365 nm). Herein, Ru-1 in H2O/CH3CN (7
:
3, v/v) solution acts as a “turn off” picric acid probe; therefore, to check the practical usefulness of Ru-1, a selectivity study in the presence of several metal ion-based quenchers such as Cu2+, Fe2+, Fe3+, Co2+, Cr3+, Mn2+, Ni2+, Ag+, Hg2+, Cd2+, and Pb2+ was performed in a PL spectroscopic channel under the same experimental conditions. As shown in Fig. 6c, except PA, other heavy metal and transition metal ion-based quenchers (14 equiv.) didn’t quench the PL of Ru-1.
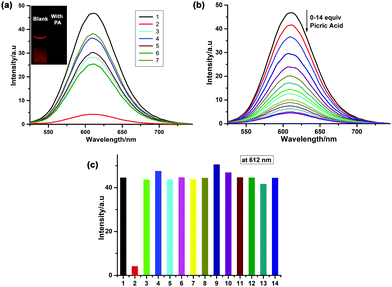 |
| Fig. 6 (a) PL spectra of Ru-1 (25 μM) in the presence of various nitroaromatic compounds (14.0 equiv.) in 70% aqueous acetonitrile solution. (1) Blank, (2) picric acid, (3) 4-nitroaniline, (4) 4-nitrophenol, (5) 4-nitrobenzaldehyde, (6) 2,4-dinitrophenol and (7) nitrobenzene. (b) PL titration of Ru-1 (25 μM) with picric acid in 70% aqueous acetonitrile solution at room temperature. [λex = 445 nm, λem = 610 nm]. (c) PL spectra of Ru-1 (25 μM) in the presence of PA and different metal ions. (1) Blank, (2) PA, (3) Cu2+, (4) Fe2+, (5) Fe3+, (6) Co2+, (7) Cr3+, (8) Mn2+, (9) Cd2+, (10) Ag+, (11) Pb2+, (12) Hg2+ and (13) Ni2+. | |
For practical applications, the limit of detection (LOD) is the key parameter to estimate the probe's sensitivity. Fig. 7a shows the calibration curve with the linear decrease of the PL intensity with increasing concentration of PA (0 to 175 μM).
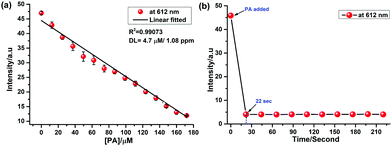 |
| Fig. 7 (a) A calibration curve for picric acid over a concentration range of 0–175 μM derived from fluorescence titration with Ru-1 (25 μM). (b) Time course of the fluorescence response of Ru-1 upon addition of picric acid at 610 nm. | |
From the slope (−1.9 × 105 M−1) of the linear plot, the LOD is calculated to be as low as 4.7 μM, which is comparable and lower than the reported ranges (Table S1, ESI†).26–28,68 The time course of PL quenching of Ru-1 in the presence of picric acid in H2O/CH3CN (7
:
3, v/v) solution was evaluated. As shown in Fig. 7b, the emission intensity was quenched immediately (< ∼22 s) after the addition of picric acid (14 equiv.) and remains unchanged over an extended period. The static quenching mechanism was ascribed to the formation of a non-luminescent Ru-1-PA conjugate (Scheme 2). The observed fluorescence quenching with picric acid is attributed to the electron transfer from photoexcited electron-rich Ru-1 to the highly electron-deficient picric acid. This electron transfer process dominates the non-radiative decay to the ground state, and substantially quenches the luminescence of Ru-1.
To find out the interactions between Ru-1 and PA in solution, a 1H NMR titration experiment was carried out in acetone-d6 (Fig. 8). The 1H NMR spectra revealed a slight change in the spectral pattern upon gradual addition of 0–14 equiv. of PA in the solution of Ru-1 (4.3 mM). The addition of PA promotes an upfield shift of the phenanthroline (H5) and phenyl proton (Ha,b,c,a′,b′) signals and downfield shift of phenanthroline (H2,3) protons of Ru-1. Also, a new signal appears at 9.17 ppm, which is the characteristic peak of PA aromatic protons. The titration result indicates that the picric acid group interacts with the L1 ligand in Ru-1via the formation of a stable Ru-1-PA conjugate, as shown in Scheme 2.
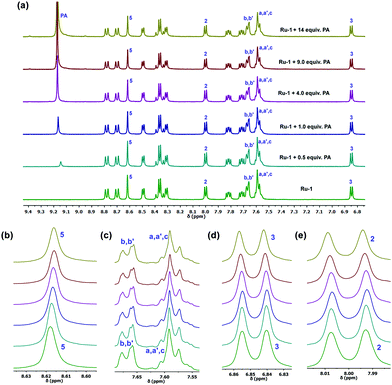 |
| Fig. 8 (a) 1H NMR titration of compound Ru-1 (4.3 mM) upon addition of PA (0–14 equiv.) in acetone-d6. A chemical shift (b) of 5, (c) phenyl (d) 2 and (e) 3 protons. | |
Furthermore, we investigated the aggregation behavior of Ru-1 upon the addition of PA in H2O/CH3CN (7
:
3, v/v). A DLS study reveals that the average size of Ru-1 is slightly increased after the addition of PA. The hydrodynamic diameters of the aggregated Ru-1 in H2O/CH3CN (7
:
3, v/v) range from 70 to 400 nm with a Zaverage of 169.2 nm, whereas after the addition of PA the Zaverage is increased to 258.5 nm (Fig. 9). This result indicates that the aggregation of Ru-1 is almost unaffected after the addition of PA in H2O/CH3CN (7
:
3, v/v).
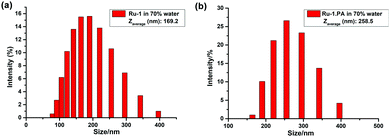 |
| Fig. 9 Particle size distribution of (a) Ru-1 and (b) Ru-1.PA in 70% water–CH3CN solution. | |
Visual detection of PA in solid state by a test paper strip
For practical application, the luminescence response towards PA is also determined in a solid-state. Ru-1 was used to prepare a portable solid-state sensor kit by dip-coating on a strip of filter paper. The Ru-1 coated paper strip shows a bright red luminescence under a laboratory UV lamp (365 nm). When 10 μL of PA solution (0.1 mM) was added to the paper strip, luminescence was fully quenched. In contrast, the luminescence of the paper strip was not observed upon the addition of other nitroaromatic analytes (10 μL; 0.1 mM) (Fig. 10). This result demonstrates the efficacy of the Ru-1 coated paper strips for the instantaneous detection of trace amounts of PA over other competitive nitroaromatic analytes.
 |
| Fig. 10 Selectivity study of various nitroaromatic analytes by Ru-1 coated paper strips upon UV irradiation at 365 nm: (1) blank, (2) picric acid, (3) 2,4-dinitrophenol, (4) 4-nitrophenol, (5) nitrobenzene, (6) 4-nitrobenzaldehyde and (7) 4-nitroaniline. | |
Theoretical calculations
The geometry optimization for complex Ru-1 was performed using Density Functional Theory (DFT). The energy-minimized structure is given in Fig. 11a, and the related bond lengths, bond angles, and Cartesian coordinates of the Ru-1 complex are given in Tables S2 and S3 (ESI†), respectively. To get insight into the electronic transitions responsible for the absorption spectrum of Ru-1, a TD-DFT calculation in the ground state was performed on the optimized geometry in acetonitrile. The theoretically obtained vertical excitation energies and composition of the related 1MLCT transition assigned to the experimental UV–vis spectrum in acetonitrile are displayed in Table 1. The result shows that the associated highest occupied molecular orbitals (HOMO, HOMO-1, and HOMO-2) are mainly located on the ruthenium(II) center (Rudz2/Rudxy), whereas the lowest unoccupied molecular orbitals (LUMO, LUMO+1, LUMO+2, and LUMO+3) are based on L1 and unsubstituted phenanthroline ligands (Fig. 11b). The experimental 1MLCT band at λmax = 445 nm (2.78 eV) is due to the strong transitions from HOMO → LUMO+3 (443.8 nm, 2.79 eV), HOMO → LUMO+2, HOMO → LUMO, HOMO-2 → LUMO+2 (442.73 nm, 2.80 eV) and HOMO-1 → LUMO, HOMO → LUMO+1 (435.64 nm, 2.84 eV).
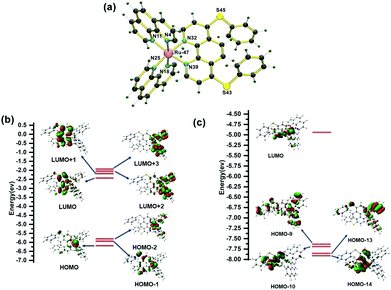 |
| Fig. 11 (a) The DFT optimized structures of Ru-1. Energy level diagram obtained from (b) ground state TD-DFT (B3LYP/6-31G+(d)//LANL2DZ) (1MLCT transitions) calculation and (c) triplet state TD-DFT (B3LYP/6-31G+(d)//LANL2DZ) calculation for Ru-1 in CH3CN (isovalue = 0.03). | |
Table 1 Selected transitions obtained from TD-DFT calculations at the B3LYP/6-31G(d,p)//LANL2DZ level responsible for experimental 1MLCT transitions for Ru-1 in CH3CN
Compound |
Wavelength (nm/eV) |
Transition |
Oscillator strength (f) |
Contribution (CI) (%) |
Experimentally observed |
Theoretically obtained |
Ru-1
|
445 nm (2.78 eV) |
443.81 nm (2.79 eV) |
H → L +3 |
0.0041 |
64.2 |
442.73 nm (2.80 eV) |
H → L +2 |
0.0015 |
64.5 |
H → L |
23.5 |
H-2 → L +2 |
11.2 |
435.64 nm (2.84 eV) |
H-1 → L |
0.0841 |
54.2 |
H → L +1 |
37.0 |
The TDDFT calculation at the triplet excited state reveals that the experimentally weak emission band of Ru-1 at ∼610 nm in acetonitrile is composed of 3MC, 3MLCT[Ru(dπ)→phen(π*)], and 3LLCT[L1(π)→phen(π*)] transition states, which are assigned as HOMO–10 → LUMO and HOMO–14 → LUMO transitions at 609.71 nm and HOMO–13 → LUMO and HOMO–9 → LUMO transitions at 593.79 nm. In CH3CN, complex Ru-1 is weakly luminescent owing to the population of the dark 3MC excited state at room temperature, and consequently, non-radiative decay occurs from the 3MC state (Table 2 and Fig. 11c).
Table 2 Selected TD-DFT transitions of the low-lying triplet excited state of Ru-1 calculated in CH3CN, which are responsible for experimental emissions at 610 nm
Compound |
Wavelength (nm/eV) |
Transition |
Contribution (%) |
Character |
Experimentally observed |
Theoretically obtained |
Ru-1
|
610 nm (2.03 eV) |
609.71 nm (2.03 eV) |
H-10 → L |
76.2 |
3MC/3MLCTRu→phen/3LLCT(L1→phen) |
H-14 → L |
12.3 |
593.79 nm (2.08 eV) |
H-13 → L |
86.0 |
H-9 → L |
21.3 |
Experimental section
Materials and physical measurements
All of the chemicals used for synthesis and sensing studies were purchased from commercial suppliers (Aldrich, Alfa Aesar, and Spectrochem India) and used as received without further purification. All 1D and 2D NMR spectra were recorded on a Bruker AVANCE II (400 MHz) spectrometer, and chemical shifts were expressed in ppm using residual protic solvent as the internal standard. Electrospray ionization high-resolution electrospray ionization mass spectrometry (ESI-HRMS) data were collected in an Agilent QTOF 6520 mass spectrometer. All the NMR and ESI-HRMS data were processed in MestReNova V12.0.0-20080. Infrared spectra were recorded using a PerkinElmer FT-IR spectrometer with KBr pellets in the range of 4000–400 cm−1. Elemental analysis measurements were done using a PerkinElmer 2500 series II elemental analyzer. UV–vis spectra were recorded on a Carry 60 UV–vis spectrophotometer (Agilent Technologies), whereas PL spectra were recorded on Hitachi F-4500 and PerkinElmer LS55 fluorescence spectrophotometers with a quartz cuvette (path length = 1 cm). Excitation and emission slits for photoluminescence data collection were set to 10 mm, and the PMT voltage was 700 V. Electrochemical experiments were performed in a CHI 600C electrochemical workstation (CH Instruments) at 25 °C. A Malvern Zen 3690 instrument has been used for dynamic light scattering (DLS) measurements. Transmission Electron Microscopy (TEM) analysis was carried out using JEOL JSM 100CX with an accelerating voltage of 200 kV. However, Scanning Electron Microscopy (SEM) analysis was performed using JSM-6360 (JEOL) with an accelerating voltage of 1.0 kV.
Synthesis of 4,7-bis(phenylthio)-1,10-phenanthroline (L1).
4,7-Dichloro-1,10-phenanthroline (0.250 g, 1.00 mmol) was taken in a round bottom flux and dissolved in 20 mL of THF. After that, sodium acetate (0.164 g, 2.00 mmol) was added to the reaction mixture. Afterward, thiophenol (225 μL, 2.20 mmol) was added to the reaction mixture. Then the reaction mixture was stirred at 90 °C for 24 h. After evaporating the THF, the crude solid was dissolved in a dichloromethane/water mixture (30 mL; 1
:
1, v/v) and stirred at room temperature for 1 h. The organic layer was collected and evaporated in a rotary evaporator under reduced pressure. The compound was washed with diethyl ether, and a pure yellow solid product was isolated in a 52% (0.205 g) yield. Anal. calcd for C24H16N2S2 (MW = 396.53): C, 72.70; H, 4.07; N, 7.06. Found: C, 72.78; H, 4.01; N, 7.10. ESI-MS [C24H16N2S2 + H]+: Calcd, m/z 397.08; found, m/z 397.08. 1H NMR (400 MHz, CDCl3): δ (ppm) = 8.84 (d, J = 4.8 Hz, 1H), 8.27 (s, 1H), 7.63–7.61 (m, 2H), 7.52–7.51 (m, 3H), 7.00 (d, J = 4.2 Hz, 1H).13C NMR (100 MHz, CDCl3): δ (ppm) = 149.4, 149.0, 145.5, 135.2, 130.2, 129.9, 129.4, 126.0, 122.0, 120.2.
Synthesis of [bis(1,10-phenanthroline) (4,7-bis(phenylthio)-1,10-phenanthroline)] ruthenium (II) hexahexafluorophosphate] (Ru-1).
The ligand, 4,7-bis(phenylthio)-1,10-phenanthroline (L1) (0.067 g, 0.170 mmol), and cis-[Ru(phen)2Cl2] (0.080 g, 0.150 mmol) were taken in a 100 ml three neck round bottom flask. The flask was degassed and purged with N2. After that, 40 ml of a degassed ethanol–water mixture (2
:
1, v/v) was added into the flask through a septum under a N2 atmosphere. The mixture was heated to reflux under N2 for 12 h. The reaction was continuously monitored by thin layer chromatography. After completion of the reaction, ethanol was evaporated and the reaction mixture was treated with excess of KPF6 (0.400 g). The mixture was stirred for 20 min for complete precipitation. The dark red precipitate was dissolved in dichloromethane (DCM). The DCM layer was collected and evaporated under reduced pressure which afforded a dark red solid compound. The crude compound was then purified by 60–120 mesh silica gel column chromatography (DCM
:
CH3CN; 75
:
25 as an eluent). During column chromatography the dark red band was collected. After evaporation of the collected portion, it was washed with diethyl ether (20 ml × 3) and dried under vacuum to get desired pure complex, Ru-1, as a dark red crystalline solid. Yield: 85% (0.147 g). Anal calcd. for C48H32N6P2F12S2Ru (Mw = 1147.94) C, 50.22; H, 2.81; N, 7.32; found: C, 50.29; H, 2.78; N, 7.37; FTIR in KBr disc (ν/cm−1): 3448, 2924, 1707, 1623, 1581, 1545, 1476, 1022, 839, 692. ESI-MS [C48H32N6S2Ru]2+: calcd, m/z = 429.06; found, m/z = 429.12. ESI-MS [C48H32N6PF6S2Ru]+: calcd, m/z = 1003.08; found. m/z = 1003.00. 1H NMR (400 MHz, acetone-d6): δ (ppm) = 8.77 (d, J = 9.2 Hz, 1H, H4′), 8.69 (d, J = 8 Hz, 1H, H7′), 8.61 (s, 1H, H5), 8.48 (d, J = 5.2 Hz, 1H, H2′), 8.35 (dd, J = 8.8 Hz, 14.4 Hz, 2H, H5′,6′), 8.29 (d, J = 6.4 Hz, 1H, H9′), 7.98 (d, J = 6 Hz, 1H, H2), 7.80 (dd, J = 8.4, 5.2 Hz, 1H, H3′), 7.70 (dd, J = 8.4, 5.2 Hz, 1H, H8′), 7.67–7.65 (m, 2H, Hb,b′), 7.60–7.56 (m, 3H, Ha,a′,c), 6.85 (d, J = 6 Hz, 1H, H3), 13C NMR (100 MHz, acetone–d6): δ (ppm) = 154.0 (C9′), 153.9 (C2′), 152.8 (C2), 152.4 (C12), 148.9 (2C, C13′,14′), 148.1 (C13), 137.7 (2C, C4′,7′), 136.6 (2C, Cb,b′), 131.9 (2C, C11′,12′), 131.7 (3C, Ca,a′,c), 129.0 (2C, C5′,6′), 128.7 (C4), 127.5 (Cc′), 127.1–127.0 (2C, C3′,8′), 124.4 (C5), 122.0 (C3).
Electrochemical studies
A three electrode cell system has been taken for electrochemical analysis, which contains a Pt working electrode, a Pt wire auxiliary electrode, and an Ag wire as a pseudo-reference electrode. Experiments were performed in 1.0 mM of Ru-1 solutions in dry and degassed acetonitrile in the presence of supporting electrolyte tetra-n-butylammonium perchlorate (0.10 M). To compare the change of the oxidation potential of Ru-1, the cyclic voltammetry data of [Ru(Phen)3](PF6)2 was also collected under the same experimental conditions. The electrochemical potential window was calibrated using ferrocene (as an internal standard) after each experiment. The standard redox potential of the ferrocene/ferrocenium (Fc/Fc+) couple was taken as +0.400 V vs. Ag wire electrode. A scan rate of 100 mV s−1 was kept for all the measurements.
Calculation of limit of detection (LOD)
The LOD was calculated based on the PL titration data of Ru-1 in the presence of picric acid (PA) (0–175 μM). To determine the S/N ratio, the standard deviation of the blank solution was calculated with ten replicate data of Ru-1 without the addition of PA. Finally, the LOD of Ru-1 for PA was calculated from the following equation.
where σ is the standard deviation of the blank solution, and K is the slope obtained from the plot of the calibration curve.
Computational studies
The geometry optimization of Ru-1 (singlet) was performed with the Gaussian 09 and Gaussian 16 program packages, using density functional theory (DFT). The B3LYP/6-31G+(d)69 basis set was used for C, H, N, and O together with LANL2DZ70 for ruthenium. Time-dependent density functional theory (TD-DFT) calculations at the ground-state geometry in acetonitrile were performed in conjunction with the conductor like polarizable continuum model (CPCM)71 for acetonitrile with a spin-restricted formalism to examine low-energy excitation at the same level of calculation, which is employed for geometry optimizations with 50 number states. The triplet state TDDFT calculations were performed using the optimized ground state geometry at the same level [B3LYP/6-31G+(d)] associated with the PCM (acetonitrile) were employed for singlet–triplet transitions to the study of the nature of the non-emissive and emissive states of Ru-1.
Preparation of test paper strips with Ru-1 and solid-state PA detection
To use complex Ru-1 for the solid-state detection of PA in a manageable way, a simple paper strip (1 cm × 1 cm) loaded with Ru-1 was prepared by dipping the filter paper into an acetonitrile solution of Ru-1 (1 mM) for 10 s and drying under vacuum. To demonstrate its application in a paper-based luminescence sensing of PA, different nitroaromatics in acetonitrile (1 × 10−5 M) were added dropwise on the test paper strips using a micropipette. After drying the strips under vacuum, the strips were observed under a 365 nm UV light, and the photos were taken by a Nikon D90 DSLR camera with a Nikon AF-S DX Nikkor 35 mm f/1.8 G Prime Lens.
Conclusions
In conclusion, a bis-heteroleptic Ru(II) complex, Ru-1, of 4,7-bis(phenylthio)-1,10-phenanthroline and 1,10-phenanthroline has been synthesized. The photophysical properties of Ru-1 were examined by UV–Vis and fluorescence spectroscopy. Theoretical calculations were performed to get the ground state energy minimized structure and to assign electronic transitions responsible for absorption and emission spectra in complex Ru-1. The weak luminescence of Ru-1 is due to the nonradiative decay via a mostly populated 3MC state. Compound Ru-1 shows the AIE properties in water and PEG, and the aggregated state exhibits bright red luminescence. The formation of the aggregated particles was supported by DLS, SEM, and TEM. The PL study revealed that the luminescence quenching of Ru-1 in the presence of picric acid (PA) was highly selective over other nitroaromatic compounds and also natural metal ion quenchers. The limit of detection for PA was calculated to be as low as 4.7 μM. The detection mechanism was discussed in detail, and the results demonstrated that the rapid PL quenching of complex Ru-1 in the presence of PA is due to the formation of a non-luminescent complex conjugate and a donor to acceptor charge transfer between Ru-1 and PA. To the best of our knowledge, this is the first report on a Ru(II) complex-based AIE-active probe for “turn-off” picric acid detection. For practical applications, a solid-state paper strip coated with complex Ru-1 has been employed to detect PA without any interference by other competitive nitroaromatic analytes selectively.
Author contributions
S. K. Patra: conceptualization, methodology, data collection, investigation and validation, and writing – original draft; B. Sen: theoretical study; M. Rabha: UV–vis and PL data collection; S. Khatua: conceptualization, supervision, project administration, formal analysis and review and editing.
Conflicts of interest
There are no conflicts to declare.
Acknowledgements
This work has been financially supported by DST, India (No. SB/FT/CS-115/2012), and DST-SPLICE, India (DST/CCP/HICAB/SN-Meghalaya/178/2018(G)). We thank the Sophisticated Analytical and Instrumentation Facility (SAIF), North Eastern Hill University (NEHU), for NMR data. S. K. P. and B. S. thank CSIR (SRF) for their fellowship.
Notes and references
- R. H. Friend, R. W. Gymer, A. B. Holms, J. H. Burroughes, R. N. Marks, C. Taliani, D. D. C. Bradley, D. A. Dos Santos, J. L. Brédas, M. Lögdlund and W. R. Salaneck, Nature, 1999, 397, 121–128 CrossRef CAS.
- J. Mei, Y. Huang and H. Tian, ACS Appl. Mater. Interfaces, 2018, 10, 12217–12261 CrossRef CAS PubMed.
- J. Shi, Y. Li, Q. Li and Z. Li, ACS Appl. Mater. Interfaces, 2018, 10, 12278–12294 CrossRef CAS.
- Y. Huang, Z. Wang, Z. Chen and Q. Zhang, Angew. Chem., Int. Ed., 2019, 58, 9696–9711 CrossRef CAS PubMed.
- J. Luo, Z. Xie, J. W. Y. Lam, L. Cheng, H. Chen, C. Qiu, H. S. Kwok, X. Zhan, Y. Liu, D. Zhu and B. Z. Tang, Chem. Commun., 2001, 1740–1741 RSC.
- Y. Hong, J. W. Y. Lam and B. Z. Tang, Chem. Soc. Rev., 2011, 40, 5361–5388 RSC.
- J. Mei, N. L. C. Leung, R. T. K. Kwok, J. W. Y. Lam and B. Z. Tang, Chem. Rev., 2015, 115, 11718–11940 CrossRef CAS PubMed.
- X. Cai and B. Liu, Angew. Chem., Int. Ed., 2020, 59, 9868–9886 CrossRef CAS.
- Z. Guo, C. Yan and W.-H. Zhu, Angew. Chem., Int. Ed., 2020, 59, 9812–9825 CrossRef CAS PubMed.
- S. K. Sheet, B. Sen, K. Aguan and S. Khatua, Dalton Trans., 2018, 47, 11477–11490 RSC.
- H. Zhang, H. Li, J. Wang, J. Sun, A. Qin and B. Z. Tang, J. Mater. Chem. C, 2015, 3, 5162–5166 RSC.
- D. Ding, K. Li, B. Liu and B. Z. Tang, Acc. Chem. Res., 2013, 46, 2441–2453 CrossRef CAS.
- P. Gopikrishna and P. K. Iyer, J. Phys. Chem. C, 2016, 120, 26556–26568 CrossRef CAS.
- L. Ravotto and P. Ceroni, Coord. Chem. Rev., 2017, 346, 62–76 CrossRef CAS.
- S. Samanta, S. Goswami, M. N. Hoque, A. Ramesh and G. Das, Chem. Commun., 2014, 50, 11833–11836 RSC.
- P. Gopikrishna, N. Meher and P. K. Iyer, ACS Appl. Mater. Interfaces, 2018, 10, 12081–12111 CrossRef CAS.
- X. Deng, X. Yu, J. Xiao and Q. Zhang, Aggregate, 2021, 2, e35 CrossRef.
- P. Alam, C. Climent, P. Alemany and I. R. Laskar, J. Photochem. Photobiol., C, 2019, 41, 100317 CrossRef CAS.
- V. Sathish, A. Ramdass, P. Thanasekaran, K.-L. Lu and S. Rajagopal, J. Photochem. Photobiol., C, 2015, 23, 25–44 CrossRef CAS.
- Y. Tian, Z.-Y. Wang, S.-Q. Zang, D. Li and T. C. W. Mak, Dalton Trans., 2019, 48, 2275–2279 RSC.
- E. Babu, P. M. Mareeswaran, M. M. Krishnan, V. Sathish, P. Thanasekaran and S. Rajagopal, Inorg. Chem. Commun., 2018, 98, 7–10 CrossRef CAS.
- S. K. Sheet, B. Sen, S. K. Patra, M. Rabha, K. Aguan and S. Khatua, ACS Appl. Mater. Interfaces, 2018, 10, 14356–14366 CrossRef CAS.
- P. Robidoux, J. Hawari and S. Thiboutot, Environ. Toxicol. Chem., 1999, 23, 1026 CrossRef.
- S. Zhang, F. Lü, L. Gao, L. Ding and Y. Fang, Langmuir, 2007, 23, 1584–1590 CrossRef CAS PubMed.
- M. L. Hu, M. Joharian, S. A. Razavi, A. Morsali, D. Z. Wu, A. A. Tehrani, J. Wang, P. C. Junk and Z. F. Guo, J. Hazard. Mater., 2021, 406, 124501 CrossRef CAS.
- V. Bhalla, A. Gupta, M. Kumar, D. S. S. Rao and S. K. Prasad, ACS Appl. Mater. Interfaces, 2013, 5, 672–679 CrossRef CAS.
- L. Ding, Y. Bai, Y. Cao, G. Ren, G. J. Blanchard and Y. Fang, Langmuir, 2014, 30, 7645–7653 CrossRef CAS.
- W. Wei, R. Lu, S. Tang and X. Liu, J. Mater. Chem. A, 2015, 3, 4604–4611 RSC.
- S. Kumari, S. Joshi, T. C. Cordova-Sintjago, D. D. Pant and R. Sakhuja, Sens. Actuators, B, 2016, 229, 599–608 CrossRef CAS.
- S. Kaur, V. Bhalla, V. Vij and M. Kumar, J. Mater. Chem. C, 2014, 2, 3936–3941 RSC.
- P. C. Ashbrook and T. A. Houts, ACS Div. Chem. Health Safety, 2003, 10, 27 Search PubMed.
- G. V. Perez and A. L. J. Perez, Chem. Educ., 2000, 77, 910 CrossRef CAS.
- P. Vishnoi, S. Sen, G. N. Patwari and R. Murugavel, New J. Chem., 2015, 39, 886–892 RSC.
- G. Sivaraman, B. Vidyaa and D. Chellappa, RSC Adv., 2014, 4, 30828–30831 RSC.
- H. Sohn, R. M. Calhoun, M. J. Sailor and W. C. Trogler, Angew. Chem., Int. Ed., 2001, 40, 2104–2105 CrossRef CAS.
- R. Rajak, M. Saraf, S. K. Verma, R. Kumar and S. M. Mobin, Inorg. Chem., 2019, 58, 16065–16074 CrossRef CAS PubMed.
- M. Bagheri, M. Y. Masoomi, A. Morsali and A. Schoedel, ACS Appl. Mater. Interfaces, 2016, 8, 21472–21479 CrossRef CAS PubMed.
- G. He, H. Peng, T. Liu, M. Yang, Y. Zhang and Y. Fang, J. Mater. Chem., 2009, 19, 7347–7353 RSC.
- S. S. Nagarkar, A. V. Desai and S. K. Ghosh, Chem. Commun., 2014, 50, 8915–8918 RSC.
- S. S. Nagarkar, B. Joarder, A. K. Chaudhari, S. Mukherjee and S. K. Ghosh, Angew. Chem., Int. Ed., 2013, 52, 2881–2885 CrossRef CAS.
- Y. Xu, B. Li, W. Li, J. Zhao, S. Select and Y. Pang, Chem. Commun., 2013, 49, 4764–4766 RSC.
- J.-F. Xiong, J.-X. Li, G.-Z. Mo, J.-P. Huo, J.-Y. Liu, X.-Y. Chen and Z.-Y. Wang, J. Org. Chem., 2014, 79, 11619–11630 CrossRef CAS.
- Y. X. Ma, H. Li, S. Peng and L. Y. Wang, Anal. Chem., 2012, 84, 8415–8421 CrossRef CAS.
- S. Maity, M. Shyamal, D. Das, P. Mazumdar, G. P. Sahoo and A. Misra, Sens. Actuators, B, 2017, 248, 223–233 CrossRef CAS.
- P.-Y. Gu, C. Wang, L. Nie, G. Long and Q. Zhang, RSC Adv., 2016, 6, 37929–37932 RSC.
- M. E. Germain and M. J. Knapp, Chem. Soc. Rev., 2009, 38, 2543–2555 RSC.
- Y. Zhang, X. Ma, S. Zhang, C. Yang, Z. Ouyang and X. Zhang, Analyst, 2009, 134, 176–181 RSC.
- S. Sanda, S. Parshamoni, S. Biswas and S. Konar, Chem. Commun., 2015, 51, 6576–6579 RSC.
- V. Bhalla, S. Kaur, V. Vij and M. Kumar, Inorg. Chem., 2013, 52, 4860–4865 CrossRef CAS PubMed.
- S. Dey, A. Maity, M. Shyamal, D. Das, S. Maity, P. K. Giri, N. Mudi, S. S. Samanta, P. Hazra and A. Misra, Photochem. Photobiol. Sci., 2019, 18, 2717–2729 CrossRef CAS.
- Z. C. Hu, B. J. Deibert and J. Li, Chem. Soc. Rev., 2014, 43, 5815–5840 RSC.
- X. M. Liu, Y. H. Xu and D. L. Jiang, J. Am. Chem. Soc., 2012, 134, 8738–8741 CrossRef CAS.
- A. Azhdari Tehrani, L. Esrafili, S. Abedi, A. Morsali, L. Carlucci, D. M. Proserpio, J. Wang, P. C. Junk and T. Liu, Inorg. Chem., 2017, 56, 1446–1454 CrossRef CAS.
- M. Raizada, F. Sama, M. Ashafaq, M. Shahid, M. Ahmad and Z. Siddiqi, J. Mater. Chem. C, 2017, 5, 9315–9330 RSC.
- J. Sahoo, S. B. Waghmode, P. S. Subramanian and M. Albrecht, ChemistrySelect, 2016, 1, 1943–1948 CrossRef CAS.
- S. H. Lee, E. Y. Lee, D. W. Yoo, S. J. Hong, J. H. Lee, H. Kwak, Y. M. Lee, J. Kim, C. Kim and J. K. Lee, New J. Chem., 2007, 31, 1579–1582 RSC.
- S. Khatua and M. Schmittel, Org. Lett., 2013, 15, 4422–4425 CrossRef CAS PubMed.
- R. B. P. Elmes, G. J. Ryan, M. L. Erby, D. O. Frimannsson, J. A. Kitchen, M. Lawler, D. C. Williams, S. J. Quinn and T. Gunnlaugsson, Inorg. Chem., 2020, 59, 10874–10893 CrossRef CAS.
- B. Sen, S. K. Patra, M. Rabha, S. K. Sheet, K. Aguan, D. Samanta and S. Khatua, Eur. J. Inorg. Chem., 2021, 3549–3560 CrossRef CAS.
- M. J. Li, K. M. C. Wong, C. Q. Yi and V. W. W. Yam, Chem. – Eur. J., 2012, 18, 8724–8730 CrossRef CAS PubMed.
- S. K. Sheet, M. Rabha, B. Sen, S. K. Patra, K. Aguan and S. Khatua, ChemBioChem, 2021, 22, 2880–2887 CrossRef CAS.
- B. Sen, S. K. Sheet, S. K. Patra, D. Koner, N. Saha and S. Khatua, Inorg. Chem., 2019, 58, 9982–9991 CrossRef CAS.
- B. Sen, M. Rabha, S. K. Sheet, D. Koner, N. Saha and S. Khatua, Inorg. Chem. Front., 2021, 8, 669–683 RSC.
- S. K. Sheet, B. Sen and S. Khatua, Inorg. Chem., 2019, 58, 3635–3645 CrossRef CAS PubMed.
- S. K. Sheet, B. Sen, R. Thounaojam, K. Aguan and S. Khatua, Inorg. Chem., 2017, 56, 1249–1263 CrossRef CAS PubMed.
- R. A. Altman and S. L. Buchwald, Org. Lett., 2006, 8, 2779–2782 CrossRef CAS PubMed.
- J. E. Collins, J. J. S. Lamba, J. C. Love, J. E. McAlvin, C. Ng, B. P. Peters, X. Wu and C. L. Fraser, Inorg. Chem., 1999, 38, 2020–2024 CrossRef CAS.
- C. Zhou, X. Han, G. Liao, C. Zhou, P. Jin, Y. Guo, H. Gao, Y. Zhang, S. Yang and J. Sun, ChemistrySelect, 2019, 4, 2868–2873 CrossRef CAS.
- A. D. Becke, J. Chem. Phys., 1993, 98, 5648–5652 CrossRef CAS.
- P. J. Hay and W. R. Wadt, J. Chem. Phys., 1985, 82, 299–310 CrossRef CAS.
- M. Cossi and V. T. Barone, J. Chem. Phys., 2001, 115, 4708–4717 CrossRef CAS.
Footnote |
† Electronic supplementary information (ESI) available: All NMR, ESI-MS data, DFT calculation related files, tables and associated figures. See DOI: 10.1039/d1nj04798a |
|
This journal is © The Royal Society of Chemistry and the Centre National de la Recherche Scientifique 2022 |
Click here to see how this site uses Cookies. View our privacy policy here.