DOI:
10.1039/D2NA00020B
(Minireview)
Nanoscale Adv., 2022,
4, 1949-1961
Application of nanotechnology in the diagnosis and treatment of acute pancreatitis
Received
10th January 2022
, Accepted 16th March 2022
First published on 19th March 2022
Abstract
Acute pancreatitis (AP) is a common digestive system disease. The severity of AP ranges from mild edema in the pancreas to severe systemic inflammatory responses leading to peripancreatic/pancreatic necrosis, multi-organ failure and death. Improving the sensitivity of AP diagnosis and developing alternatives to traditional methods to treat AP have gained the attention of researchers. With the continuous rise of nanotechnology, it is being widely used in daily life, biomedicine, chemical energy and many other fields. Studies have demonstrated the effectiveness of nanotechnology in the diagnosis and treatment of AP. Nanotechnology has the advantages of simplicity, rapidity and sensitivity in detecting biomarkers of AP, as well as enhancing imaging, which helps in the early diagnosis of AP. On the other hand, nanoparticles (NPs) have oxidative stress inhibiting and anti-inflammatory effects, and can also be loaded with drugs as well as being used in anti-infection therapy, providing a new approach for the treatment of AP. In this article, we elaborate and summarize on the potential of nanoparticles for diagnostic and therapeutic applications in AP from the current reported literature and experimental results to provide useful guidelines for further research on the application of nanotechnology.
1. Introduction
Acute pancreatitis (AP) is a relatively common acute abdominal condition requiring hospitalization. The incidence has been increasing every year for the last two decades, with a regional variation of 4.9–73.4 cases per 100
000 people.1 AP is an exocrine inflammatory disease due to the abnormal activation of pancreatic enzymes from various causes. Although most cases are mild, about 20% of them will develop into moderate to severe, with a mortality rate of about 30%.2,3
The etiology of acute pancreatitis is diverse, and the age, gender distribution and severity of patients with AP due to different causes vary. In most high-income countries, cholecystolithiasis is still the leading cause of AP, followed by excessive alcohol consumption and hypertriglyceridemia. Alcoholic acute pancreatitis and hypertriglyceridemia are more common in young male patients, while biliary sources predominate in older patients.1,4 Other less common etiologies include medications, endoscopic retrograde cholangiopancreatography (ERCP), genetics, trauma, etc. Early diagnosis and etiology control help improve the prognosis of AP and prevent recurrence.5
The commonly used clinical classification for the severity of AP is the revised Atlanta classification (RAC). RAC: (1) mild acute pancreatitis (MAP), which accounts for 80–85% of acute pancreatitis, is not associated with local or systemic complications, and usually the patients recover within 2 weeks and death does not usually occur. (2) Moderately severe acute pancreatitis (MSAP), with temporary (≤48 h) organ damage and/or local complications, has a low early mortality rate. However, when necrotic tissue is combined with infection, the mortality rate increases. (3) Severe acute pancreatitis (SAP), accounts for about 7.5% of acute pancreatitis and is accompanied by persistent (>48 h) systemic organ dysfunction and a high mortality rate.
The pathological basis of AP is the secretion of the enzymogen prolyl protease, trypsinogen and amylase from the pancreatic acinar cells (PACs) to the pancreatic ducts. These enzymes are prematurely activated in PACs and cause self-digestion in the pancreas. This pathological self-digestion leads to edema, hemorrhage, inflammation and necrosis. The damaged PACs release numerous pro-inflammatory factors, which include tumor necrosis factor-α (TNF-α), interleukin (IL)-6, IL-1β etc., which further exacerbate the inflammatory response, thereby exacerbating AP. Among them, the levels of inflammatory factors TNF-α, IL-8, and monocyte chemotactic protein (MCP-1) are elevated from the beginning of the AP disease course.6 Late in the course of AP (usually after 2 weeks), inflammatory cells increase and necrotizing pancreatitis, systemic inflammatory response syndrome, damage to distant organs including the lungs, heart, kidneys and liver, and then further development of sepsis occur, later aggravating to multiple organ dysfunction syndrome and eventually death.7,8
A series of pathophysiological changes in pancreatic alveolar cells are caused directly or indirectly by common causes of pancreatitis (e.g., pancreatic duct obstruction, alcohol, bile acids, etc.).9 The major cellular changes in the pathogenesis of AP include premature activation of trypsinogen,10 abnormal calcium signalling,11 mitochondrial injury,12 endoplasmic reticulum (ER) stress,13 impaired unfolded protein response (UPR)14 and impaired autophagy.15 The association and action between pancreatic follicular cells and the immune system allows for a continuous inflammatory reaction to occur and develop (Fig. 1).16 These pathogenic mechanisms provide potential therapeutic targets for future drug development for treating AP, particularly for the study of nanomaterials.
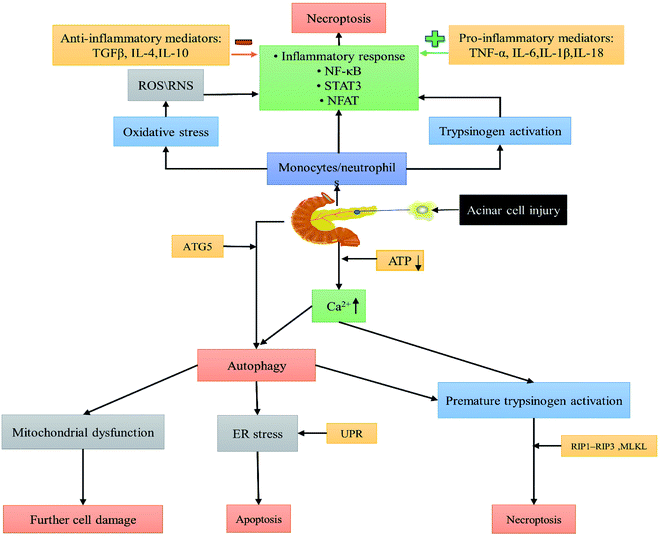 |
| Fig. 1 Major pathogenic mechanisms of acute pancreatitis include premature activation of trypsinogen, abnormal calcium signalling, mitochondrial injury, endoplasmic reticulum (ER) stress, impaired unfolded protein response (UPR) and impaired autophagy. | |
The typical symptoms of acute pancreatitis are acute onset of epigastric continuous severe pain, accompanied by abdominal distension, nausea, and vomiting; pain cannot be relieved after vomiting; and severe cases may develop hypovolemic shock. Severely dehydrated and elderly patients may have altered mental status. AP can be complicated by one or more organ dysfunction, with lung injury and renal impairment being common. Laboratory tests may reveal elevated pancreatic enzymes (e.g., serum amylase and lipase), and elevated lipase is more specific than amylase for the diagnosis of AP. Elevated levels of these two pancreatic enzymes were not associated with the severity of AP. Abdominal CT is an essential imaging method for AP diagnosis. Typical radiographic findings of early AP are pancreatic edema, peripancreatic exudate, and pancreatic and/or peripancreatic tissue necrosis.
According to international guidelines and consensus, the diagnosis of AP is based on two of the following three conditions: (1) severe epigastric pain; (2) serum amylase or (and) serum lipase at least more than three times the normal value; or (3) abdominal ultrasound, contrast-enhanced computed tomography (CT), magnetic resonance imaging (MRI) and other imaging studies are consistent with the presentation of acute pancreatitis.1,17,18
In recent decades, the methods of diagnosis and treatment of acute pancreatitis have also changed significantly, reducing mortality rates. In addition to CT and MRI, endoscopic diagnosis, minimally invasive treatment and multidisciplinary diagnosis and treatment have also been developed. However, diagnosis still relies mainly on laboratory tests and imaging. Treatment depends mainly on drug therapy, which has certain drawbacks.19–21 For example, the commonly used laboratory test, namely the spectrophotometric method for amylase is time-consuming and less sensitive (<10−6 mol L−1); imaging tests such as CT and MRI are not sensitive to early AP.22,23 Drugs targeting inflammatory cytokines in AP have not been approved for clinical use. This has all led researchers to develop new methods to improve the diagnostic sensitivity and therapeutic efficacy for AP.
At present, nanotechnology is an emerging and popular discipline that is widely used in medical biology, for example nano-drug delivery systems,24 antibacterial and anti-inflammatory agents,25–27 diagnostic imaging,28–30 photodynamic and photothermal therapeutic agents,31,32 and multimodal combination therapy.33–35 Nanoparticle-based therapeutic modalities have emerged as promising treatments for AP. NPs are usually in the particle size range of 1–100 nm, with a maximum of 1000 nm. These particles are formed by natural or artificial manipulation of polymers or metals.36–38 Nanotechnology mainly plays its diagnostic and therapeutic roles by changing the size and architecture of different NPs or connecting different nanomaterials to form complexes.39–41 What's more, the modified nanomaterials have different functionalization and targeting to be more useful.42–45 As the research has progressed, different “nanodiscs” with multifunctionalization have been developed, such as nanosheets and “nano-boats”.46,47 Meanwhile, the advantage of nanomaterial targeting is that it can target specific target cells, such as tumor cells and macrophages, with effective affinity for therapeutic purposes.48,49 NPs have been widely reported in the diagnosis and treatment of AP. The purpose of this review is to discuss the clinical implications of the different nanoplatforms that have been published in recent years for the diagnosis and therapy of AP.
2. Diagnosis
2.1 Laboratory tests
2.1.1 Amylase.
Amylase is a calcium metalloenzyme present in normal human serum, urine and saliva. Amylase activity in both serum and urine is used as an essential biomarker for the diagnosis of AP.50 Attia et al. synthesized a nano-optical dinuclear sensor, the Pd-(2-aminothiazole) (urea) complex, to detect amylase activity in serum and urine. Amylase activity was evaluated by the quenching of the Pd(atz, ur) complex at 457 nm laser with 2-chloro-4-nitrophenol producing a different luminescence intensity of the reacting enzyme. The sensitivity and specificity of serum amylase detection by this method to improve the diagnosis of AP were reported to be 96.88% and 94.41%.51 In addition, Shi et al. reported a rapid ultra-sensitive tetraphenylethylene probe based on polymerization-induced emission for the direct detection of amylase in serum or urine. This probe is hydrolyzed by amylase to release insoluble AIE residues with significantly enhanced fluorescence, allowing quantitative analysis with the advantages of being a simple, rapid and highly sensitive procedure.52 Moreover, Mandal et al. developed a biosensor consisting of a conductive polyaniline–polyaniline amine salt film covered by starch-coated gold nanoparticles (SAuNPs) for point-of-care and sensitive detection of amylase content. The resistance of the sensor is modified by adding different doses of amylase to the biosensor, which selectively depletes the starch stabilized on SAuNPs. The electrical resistance is linearly related to the amylase concentration, whereby the activity is measured (Fig. 2).53
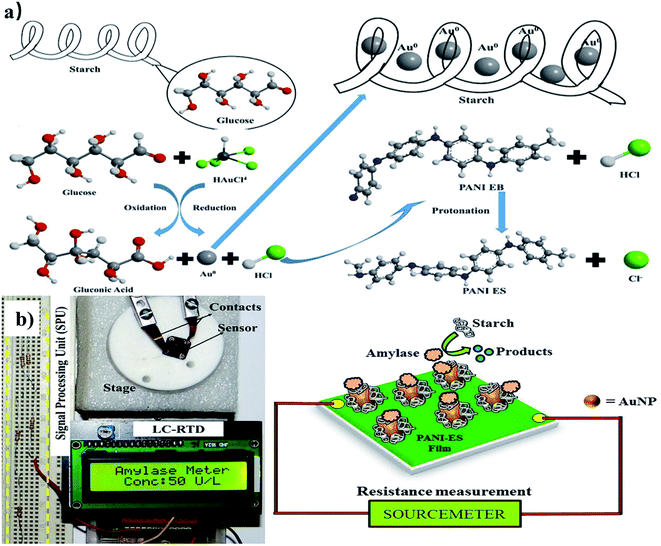 |
| Fig. 2 (a) Preparation process of the amylase sensor. (b) Point-of-care testing of amylase in blood serum. Reproduced with permission from ref. 53. Copyright © 2018 Elsevier B.V. | |
2.1.2 Trypsin.
Trypsin is one of the digestive enzymes secreted by the pancreas and it is stored as an inactivate precursor in enzymogenic pellets. Under normal conditions, pancreatic acinar cells can properly inhibit trypsin activity. In pancreatitis there is an excessive activation of various proteases (especially trypsin) which eventually damages cells.54 Trypsin is also one of the biomarkers of pancreatitis.55 Since urination removes biological waste and metabolic products, including proteins and peptides, from the blood, overexpression of trypsin (up to 84.4 μg mL−1) is frequently detected in the urine of patients with pancreatitis.56 A multifunctional nanoprobe was designed for trypsin quantitative analysis with fluorescence resonance energy transfer (FRET) by Poon et al. With the GQD–BSA–CMR2 complex as a nanoprobe, the FRET system is disrupted by BSA in the presence of trypsin, and the amount of trypsin can be quickly determined by measuring the ratio of these two emission peaks (Fig. 3).57 Furthermore, Lucas et al. demonstrated a metal-enhanced fluorescence (MEF) based principle for shutting down enzyme assays for the rapid detection of low concentrations of trypsin.58 Besides, Hou et al. reported a novel sensor for the detection of trypsin by fluorescently labeling peptides with negatively charged carbon nanoparticles (CNPs) modified with acid oxidation. Quantification of trypsin activity is through dye release and the intensity of endocytosis with a detection limit of 0.7 mg mL−1.59 Recently, Guan has developed a highly sensitive trypsin serum sensing system using a mixed ion permeable membrane based on anodic aluminum oxide (AAO) and modified with a trypsin substrate. Based on the change in the electrical properties of the peptide-modified hybrid ion membrane surface after a trypsin-initiated peptide hydrolysis reaction and the electric double layer effect reducing the effective ion current diameter within the AAO nanocells and improving the ion current rectification ratio, the minimum quantitative detection of trypsin concentration can reach 0.1 pM.60
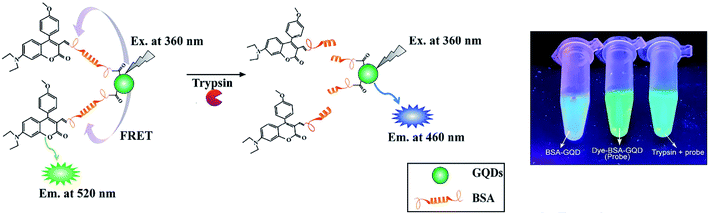 |
| Fig. 3 Nanoprobe (GQD-BSA, left), GQD-BSA-CMR2 (middle) and GQD-BSA-CMR2 with trypsin (right) under a UV lamp. Reproduced with permission from ref. 57. Copyright ©2016 Elsevier B.V. | |
Nanozymes are a new class of enzymes with biocatalytic activity that also retain the unique properties of nanomaterials, and have become a research hotspot in the biomedical field in recent years.61 An oxidase-like nanozyme, citrate-capped platinum nanoparticles (Cit–Pt NPs), was designed by Lin et al. Using protamine as an aggregation promoter for Cit–Pt NPs and a specific hydrolytic substrate for trypsin to enhance the oxidase activity of Cit–Pt NPs at pH 8, a one-step detection of trypsin can be achieved. This method has been successful in detecting trypsin in clinical urine samples.62
2.1.3 Serum lipase.
When the serum lipase level is more than 3 times higher than normal, it supports the diagnosis of AP, with a rapid increase in the activity of this enzyme 12 hours after the onset of the disease and a decrease to normal within 3–5 days.63 In particular, serum lipase levels are more sensitive and specific than serum amylase levels in the diagnosis of AP in all pediatric age groups.64 Chakraborty et al. developed a selectively sensing supramolecular self-assembled nanostructure for lipase using the aggregation-induced emission (AIE) property of naphthalene diimine (NDI). In different water volumes of DMSO, NDI has different morphologies and exhibits different intensities of AIE (blue-green luminescence). This nanostructure detects lipase content though hydrolysis of a benzyl ester linkage with a limit of detection 10.0 ± 0.8 μg L−1 (Fig. 4).65 Also based on the AIE mechanism, glutamate-functionalized tetraphenylethylene (TPE) has been successfully applied to real human serum samples as a fluorescent probe for the detection of lipase levels, with the advantages of fast and convenient reaction time.66
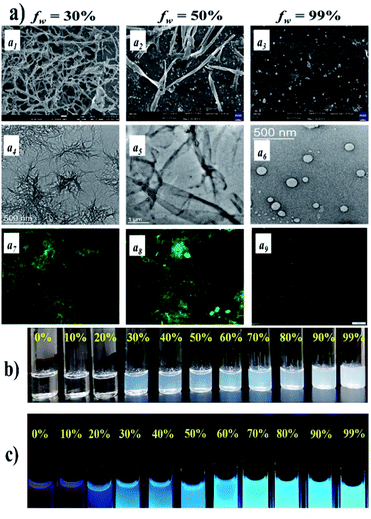 |
| Fig. 4 FESEM (a1–a3), TEM (a4–a6), and fluorescence (a7–a9) microscopy images of NDI-2 in 70 : 30, 50 : 50, and 1 : 99 (v/v) DMSO–water binary solvent mixtures, respectively ([NDI-2] = 20 mM). (b) Photograph of the formation of translucent solutions with increasing water content in DMSO for NDI-2 (50 mM) and (c) photograph of emission color of NDI-2 (50 mM) upon UV-light irradiation (lex = 365 nm). Reproduced with permission from ref. 65. Copyright ©2021 The Royal Society of Chemistry. | |
2.1.4 Triglycerides (TGs).
Hyperlipidemia is one of the causes of pancreatitis. Lipid levels affect the severity of AP, and early control can help prevent recurrence and improve prognosis.1 Biosensor detection has specificity, rapidity, simplicity, low cost and high sensitivity compared to conventional methods.67 In recent years nanomaterials used in the preparation of biosensors further improved their performance.68 Nanostructured metal oxides (NMOs) are already essential material components of biosensors, such as the SiO2–CeO2 nanocomposite, CHIT-nano-ZrO2/ITO, and ZnO nanoparticles (ZnO NPs). And they all exhibit excellent detection performance.69–71 Researchers have developed an electrochemical biosensor to detect total triglycerides in human serum samples. The sensor consists of a composite material of lipase immobilized on chitosan-coated magnetic nanoparticles (CNP-L). The level of TGs in serum was determined by measuring the level of glycerol produced by electrochemical oxidation of TGs with lipase enzymatic digestion. This biosensor has high stability and sensitivity in the detection of triglycerides.72
2.1.5 Serum creatinine.
In general, elevated serum creatinine is an essential parameter for poor prognosis in AP. Serum creatinine is closely associated with necrosis of the pancreas 48 hours after the onset of AP. Since pancreatic necrosis is a severe complication of acute pancreatitis, a rapid and sensitive test is essential.73,74 Wen et al. proposed a high-efficiency nano-Ag/Au@Au film composite SERS substrate to rapidly detect human serum creatinine. Due to the multidimensional plasma coupling effect between AuNPs, AgNPs and Au membranes, the local electromagnetic field strength is significantly increased, and a higher enhancement factor is acquired. The results demonstrated that the nanocomplex was simple to prepare, sensitive and had a detection limit of 5 × 10−6 for serum creatinine.75 Moreover, Zhybak et al. developed a novel current-based biosensor based on a novel PANi–Nafion–Cu complex and immobilized hydrolase for the detection of creatinine and urea characterization. Its characteristics are rapid analysis of sample creatinine, 15 second response, high sensitivity and specificity, and the limit value of the test is 0.5 μM.76
2.2 Imaging
The typical imaging manifestations of AP are some of the diagnostic criteria. Still, the imaging features at the initial onset of the disease do not reflect the severity of the disease and may even lead to misdiagnosis.77–79 Early detection is of great importance to reduce AP mortality. However, there is a lack of effective early detection tools in clinical practice. MRI is indicated for patients with iodine allergy and renal insufficiency, and its sensitivity in detecting pancreatic edema is better than that of CT.80 Improvements in imaging methods through nanomaterials may provide a new basis for early AP diagnosis in the future. Zhang et al. synthesized a gadolinium-based MRI nanoparticle probe as a diagnostic tool for early AP. The probe is not only low toxic to the organism but also biodegradable. The Gd–DTPA–FA nanomaterials have a T1 MRI signal amplification effect in the environment of lipase. The MRI signal was found to be the strongest at hours 1, 6, and 12 when applied in rat models (Fig. 5).81
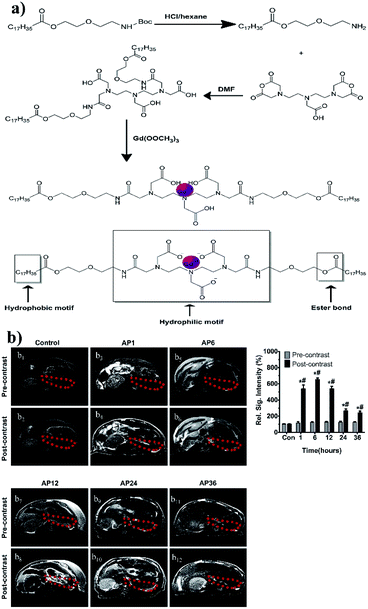 |
| Fig. 5 (a) Schematic illustration of the preparation and synthesis of Gd–DTPA–FA nanoparticles. (b) Representative magnetic resonance images of SD rats before and after tail vein injection of Gd–DTPA–FA. T1-weighted images of the pancreases were acquired from the AP 1 h, 6 h, 12 h, 24 h and 36 h groups and 0.9% NaCl groups. Areas marked with dashed red circles indicate the location of pancreases. The signal intensities of the pancreatic location on T1-weighted imaging in the AP 1 h, 6 h, 12 h, 24 h and 36 h post-contrast groups were significantly higher than that of the pre-contrast groups and that of the control group. *p < 0.01, vs. the control group; #p < 0.01, vs. the pre-contrast group. Reproduced with permission from ref. 81. Copyright ©2013 Elsevier. | |
Similarly, Long et al. constructed a diethylenetriaminepentaacetic chelate based nanoprobe for MRI imaging of early AP. It was labeled using a novel p-selectin-targeting, near-infrared fluorescent dye. It has the same T1 MRI signal enhancement effect.82 Holbrook et al. also developed Gd(III)-NPs for T1 MRI of the pancreas. This contrast agent is a multivalent DNA–gold NP conjugate rich in gadolinium(III) (Gd III). Specifically, coupling of Gd(III) to the AuNP surface does not require the action of reducing agents which can accumulate in the pancreatic tissue and have high contrast in MRI.83
Unfortunately, in contrast to the widespread application of nanotechnology in laboratory examinations of AP, the application in imaging examinations is only limited to MRI, ultrasound and CT used for AP diagnosis; however, the enhancement of ultrasound and CT using NPs has not been reported. More in-depth and extensive studies of nanomaterials in the imaging of AP are expected in the future.
3. Treatment
Most patients with mild AP are treated conservatively and can get better in 1–2 weeks. Moderate to severe acute pancreatitis may require long-term specialist care, more aggressive monitoring and treatment measures.84 They are best treated by an integrated multidisciplinary team, with imaging physicians, intensivists, and dieticians in addition to surgeons. Treatment modalities include fasting, fluid replacement, and monitoring of vital signs and nutritional support.1 To date, there are no clinically approved drugs to improve pancreatic exocrine dysfunction. With the research of nanomaterials, new treatments are brought for pancreatitis. They can play an anti-inflammatory and pancreatic tissue-protective role by inhibiting oxidative stress, inhibiting pancreatic enzyme secretion and reducing reactive oxygen species. They can be a vehicle for drug therapy and gene therapy.
Since human pancreatic tissue is difficult to obtain during an acute pancreatitis episode, animal models can mimic the pathophysiological mechanisms of AP and help in drug development and testing.85 The most commonly used model is acute pancreatitis in rats or mice induced by repeated administration of supraphysiological doses of cerulein. It is a cholecystokinin analogue that is cost effective and highly reproducible in animal models of AP. However, the drawbacks of this animal model include not only the limited relevance of the clinical occurrence mechanism but also the different distribution of local parenchymal injury compared to that in humans.86 In addition, the L-arginine induced AP model in rats has a slower progression of pancreatic injury.87 Furthermore, AP can also be induced using catheter cannulation and perfusion methods. Still the disadvantages of these models include the need for open surgery and anesthesia, which is challenging to operate.88 Encouragingly, despite the fact that animal models of AP are somewhat different from humans, researchers have achieved good therapeutic results in the treatment with nanomaterials in animal models. A foundation has been laid for the use of nanoparticles in the treatment of AP.
3.1 NPs for direct inhibition of oxidative stress and anti-inflammatory effects
In the early stage of AP, pancreatic damage is due to trypsin-mediated cell death. At the same time, multiple mechanisms of injury occur in parallel, including inflammatory cascade activation, autophagy, apoptosis and ER stress.54 Inflammatory cells such as macrophages and neutrophils recruited to damaged cells of the pancreas secrete cytokines such as IL-1β, IL-6, IL-17, and TNF-α, causing disturbances in oxidative homeostasis.89,90 In addition, the increase of reactive oxygen species (ROS) and reactive nitrogen species (RNS) further aggravated the cellular damage.91,92 Inhibition of the nuclear factor-κ-light-chain enhancer (NF-κB) and activation of nuclear factor (erythroid-derived 2)-like 2 (Nrf2) in activated B cells have been shown to have an ameliorating effect on the cellular stress observed during AP.93 Intercellular adhesion molecule-1 (ICAM-1), an adhesion molecule secreted downstream of NF-κB activation, is associated with the migration and aggregation of neutrophils and macrophages. Reducing its expression can reduce the inflammatory response.94 Furthermore, heat shock proteins (Hsp), such as Hsp27 and Hsp70, are upregulated during AP to counteract inflammatory injury, and in addition, histone acetylation is upregulated in an ordered manner during severe AP.95,96 Khurana et al. found that nanoceramics (NC) possess powerful superoxide dismutase activity and mimic peroxidase activities, anti-pancreatitis activity, and have a positive therapeutic effect on a cerulein-induced pancreatitis model in mice. The nanoparticles had the following four effects: (1) reduced the generation of ROS; (2) good DPPH clearance activity which reduced oxidative stress and nitrosation in macrophages; (3) reduced pancreatic enzyme secretion and lowered liver enzyme levels; and (4) exerted protective effects by upregulating Nrf2, SOD1 and NQO1, while downregulating inducible nitric oxide synthase, p65 NF-κB, Hsp27 and Hsp70.97 In the same year, they also found that nanoyttria (NY) not only has potent free radical scavenging activity, but also reduces plasma amylase and lipase levels. Likewise it inhibits the aggregation of inflammatory cells in damaged pancreatic follicular cells. Furthermore, NY intervention interferes with AP processes by reducing ER stress markers and molecular chaperones.98 Yao et al. developed bilirubin encapsulated silk fibrin nanoparticles (BRSNPs), which can be selectively delivered to inflammatory lesions in the pancreas and bilirubin is released in an enzymatic response to reduce oxidative stress and decrease the expression of pro-inflammatory factors (TNF-α and ICAM-1) to treat AP.99
Moreover, there is a structure similar to the blood–brain barrier between the pancreatic tissue and the blood – the blood–pancreatic barrier (BPB), which between the pancreatic tissue and blood further limits the role of drugs in the pancreas.100 Zhou et al. developed celastrol-loaded PEG–PLGA NPs wrapped by neutrophil membranes with a particle size of 150 nm. Their greatest advantage is that they can penetrate the blood–pancreatic barrier (BBB) and accumulate to the maximum extent in the pancreas. The NPs attenuate acute pancreatitis by down-regulating serum amylase and pancreatic myeloperoxidase levels in AP rats.101 Similarly, generation 5 (G5) polyamine dendrimers – G4.5-COOH and G5-OH, which have different surface groups, significantly reduced pancreatic pathological changes, decreased macrophage aggregation in pancreatic tissue, and inhibited pro-inflammatory cytokine levels (Fig. 6).102Table 1 shows the effects and mechanisms of different types of nanoparticles in the treatment of AP.
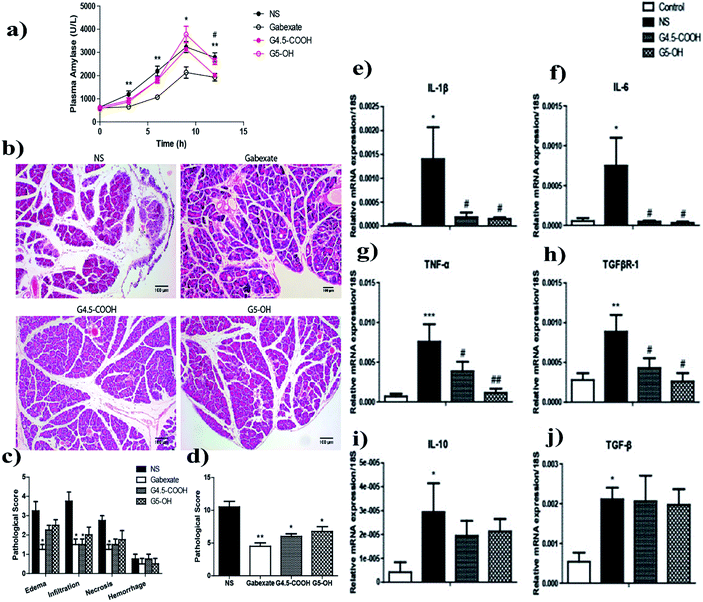 |
| Fig. 6 Plasma amylase (a), pancreatic morphological changes (b), and pathological scores (c and d) of caerulein-induced AP mice. Quantification of cytokine mRNA levels in pancreatic tissues from AP mice. Pancreatic mRNA expression levels of pro-inflammatory cytokines: IL-1β (e), IL-6 (f), TNF-α (g), and TGFβR-1 (h), and anti-inflammatory cytokines: IL-10 (i) and TGF-β (j) in pancreatic tissues from NS, G4.5-COOH, or G5-OH pretreated and AP-induced mice were measured and compared with that of normal control mice. Reproduced with permission from ref. 102. Copyright ©2014 American Chemical Society. | |
Table 1 Different nanoparticles used in the treatment of AP. (1) G4.5-COOH, G5-OH: generation 5 polyamidoamine dendrimers with two different surface groups; (2) NY: nanoyttria; (3) NC: nanoceria; (4) PEG–PLGA NNPs/CLT: celastrol (CLT)-loaded PEG–PLGA nanoparticles (NPs/CLT) coated with neutrophil membranes (NNPs/CLT); (5) CA-NPs: cinnamic acid nanoparticles; (6) BRSNPs: bilirubin encapsulated silk fibrin nanoparticles; (7) FA–SF–NPs: ferulic acid (FA)–silk fibroin (SF)-NPs (nanoparticles)
Category |
Types of NPs (size) |
Effects |
Mechanisms |
Animal model of AP |
Ref. |
Dendrimers |
G4.5-COOH, G5-OH (5 nm) |
Anti-inflammatory, protection of pancreas |
Restrain NF-κB nuclear translocation, reduce expression of IL-1β, IL-6, and TNF-α |
Caerulein/mice |
102
|
Metallic oxide |
NY (159 ± 7.5 nm) |
Anti-oxidative stress, anti-inflammatory, protection of pancreas |
Reduce ER stress markers (BiP, IRE1 and Ero1-Lα), molecular chaperones Hsp27 and Hsp70 |
Caerulein/mice |
98
|
Metallic oxide |
NC (82 ± 5 nm) |
Anti-oxidative stress, anti-inflammatory, reduction of nitrosative stress, protection of pancreas |
Remove DPPH; reduce ROS, upregulate Nrf2, SOD1 and NQO1, downregulate p65-NF-κB, Hsp27, and Hsp70 |
Caerulein/mice |
97
|
Complex |
PEG–PLGA NNPs/CLT (150 nm) |
Overcoming the blood–pancreas barrier, anti-oxidative stress, anti-inflammatory |
Downregulate serum amylase and MPO levels, restrain NF-κB, reduce expression of IL-6 and TNF-α |
Sterile laparotomy/rats |
101
|
Nonmetallic elemental |
Nano-Se (20–60 nm) |
Anti-inflammatory, antioxidant |
Restrain NF-κB, reduce expression of IL-1β, IL-6, and TNF-α |
L-Arginine/rats |
103
|
Organic acid |
CA-NPs (50–90 nm) |
Reduction of pancreatic enzyme secretion, anti-oxidative stress, anti-inflammatory |
Downregulate NLRP3, NF-κB and ASK1/MAPK signaling pathways; decrease caspase-3 levels |
L-Arginine and gamma radiation/rats |
104
|
Complex |
BRSNPs (268 ± 6 nm) |
Anti-oxidative stress, anti-inflammatory |
Regulate NF-κB and Nrf2/HO-1 pathways, reduce expression of CD68, MPO, ICAM-1, and TNF-α |
L-Arginine/rats |
99
|
Complex |
Se@SiO2 (50 nm) |
Reduction of pancreatic enzyme secretion, anti-inflammatory, anti-oxidative stress, protection of distant organs |
Restrain the TLR4/Myd88/p-p65 pathway, increase the levels of NQO1, Nrf2, and HO-1 protein |
Caerulein/mice |
105
|
Complex |
FA–SF-NPs (186 nm) |
Anti-oxidative stress, anti-inflammatory, protection of pancreas |
Inhibit NF-κB nuclear translocation, reduce expression of IL-1β, IL-6, and TNF-α |
Biliopancreatic duct ligation/rats |
106
|
3.2 NPs for anti-inflammatory drug delivery
Currently, nanoparticles are widely used as drug carriers for the treatment of diseases. Some mesoporous materials (e.g. silica, manganese dioxide) are used as carriers for tumor chemotherapy drugs or antibiotics to enhance the anti-tumor and anti-bacterial effects and reduce the toxic side effects of drugs or combined with loads of photosensitizers and photothermal agents to form a multi-modal killing effect on tumors or bacteria.107–111 Carbon-based nanomaterials (e.g. fullerenes, graphene) also have the same carrier function.112–114 For patients, oral administration is the most convenient and comfortable method, but the poor water solubility of many small molecules in clinical practice reduces their oral bioavailability.115 Chuang et al. developed a self-assembled enteral drug delivery system for oral capsule delivery, where the capsule can be dissolved in the intestine. The system includes an acid initiator, foaming agent, surfactant and low water-soluble drug (curcumin; CUR). This system becomes CUR-laden nanoemulsions in the intestinal environment, which can passively target intestinal M cells and subsequently transport them into pancreatic tissue via the intestinal lymphatic system, increasing the concentration approximately 12-fold in the pancreas of AP rats to exert pharmacological anti-inflammatory effects.116 Previous studies have shown that carbon monoxide (CO) treatment of macrophages increases the secretion of anti-inflammatory cytokines and suppresses pro-inflammatory cytokine levels. However, CO is difficult to control in the body. For this purpose, researchers developed CO-bound hemoglobin vesicles as a novel donor for CO, a red blood cell-like structure with good intra-organismal compatibility and long half-life cycle, which inhibits AP by suppressing pro-inflammatory cytokine production and reduces oxidative damage of pancreatic tissue. Satisfactory results were obtained in the treatment of AP in mice. In addition, it reduces the injury to other vital organs including the lungs, heart, liver and kidneys by neutrophil infiltration inhibition.117
3.3 NPs for gene therapy
Recently, gene therapy has provided a new way of treating pancreatic diseases.118,119 It has been reported that gene therapy for pancreatitis may be more effective than drug therapy in exerting anti-inflammatory effects.120,121 Back in 2002, a plasmid-hIL-10 construct (pcDNA3-hIL-10) in combination with cationic liposomes was used for the treatment of AP rats. It improved histological lesions of the pancreas and other organs and inhibited the elevation of pancreatic enzymes, reducing mortality.122 Specifically, gene therapy for pancreatitis pain exhibited significant analgesic effects over the duration of transgene expression (approximately 4–6 weeks).123 A nanoparticle complex Ca–CQ (chloroquine diphosphate)–pDNA–PLGA-NPs, with good targeting and therapeutic effects on mouse pancreatic tumor and pancreatitis models, was developed by Yang et al. After they constructed plasmid pVITRO2 expressing mSurvivin-T34A protein, it was mixed with Ca–CQ solution to form a complex. This complex can enhance gene therapy of AP while also exerting CQ anti-inflammatory effects.124 Nanotechnology combined with gene therapy is a hot research area in the future.
3.4 NPs for anti-infection therapy of AP patients
As the disease progresses, 20% of patients with AP develop necrotizing pancreatitis (NP). Infectious complications are present in approximately 40–70% of NP patients, and usually imply a poor prognosis.125,126 The inflammatory response during acute pancreatitis can lead to intestinal flora disorders, bacterial translocation and impaired intestinal barrier function and is considered to be an important cause of AP complications such as pancreatic necrosis and peripancreatic infection.127,128 Infection is a distinct marker of complications in patients with NP. Most of the bacteria in AP infections are of intestinal origin: Gram-negative (G−) bacteria such as Escherichia coli and Klebsiella pneumoniae, some Gram-positive (G+) bacteria such as Staphylococcus aureus, but also anaerobic bacteria.129 However, fungal infections increased from 5–8% before treatment to 20–30% after long-term treatment with multiple broad-spectrum antibiotics.130 Traditional small-molecule antibiotics work mainly by interfering with bacterial cell wall formation, disrupting the cell membrane structure, and inhibiting protein synthesis and DNA replication. With the misuse of antibiotics, both have significantly increased the level of bacterial resistance worldwide.131
Nanotechnology applied to the development of new antibiotics can effectively avoid the development of bacterial drug resistance. Nanoparticles (NPs) of some metals, such as silver and gold, inhibit the growth of microorganisms by a series of effects resulting from a wide range of molecular interactions with them.132 There are also silver-based nanoparticles whose main antimicrobial effect is the generation of reactive oxygen species (ROS) such as H2O2, hydroxyl and superoxide radicals that cause oxidative damage.133 Photothermal therapy (PTT) kills bacteria by using a photothermal sensor that converts near infrared (NIR) light into heat.134 Many nanomaterials have been developed to play a photothermal antibacterial role, such as Pd–Cu nanoalloy, Prussian blue NPs, etc.135,136 Furthermore, there are a few composite nanoplatforms with multimodal antimicrobial effects that not only enhance the antimicrobial effect of their loaded antimicrobial drugs, but also have photothermal or photodynamic effects.137,138
Nanomaterial antimicrobial therapy is effective against multi-drug resistant bacteria and less likely to develop drug resistance, and hence it brings new ideas to anti-infection treatment. Although the effectiveness of NPs for systemic sepsis in mice has been reported in the literature, little has been reported for the treatment of acute pancreatic necrotizing infections.139,140
4 Summary and outlook
AP is a common digestive disease that can be life-threatening in severe cases. Even those who survive may have a poor quality of life with chronic pancreatitis and diabetes for a long time. It places a heavy burden on the health care system every year. Early diagnosis and effective treatment are of great importance to the prognosis of the disease. With the development of nanotechnology, new modalities have been provided for the diagnosis and treatment of AP.
Nanomaterials and nanomaterial-based biosensors have high sensitivity, short duration and uncomplicated procedures compared to traditional clinical detection methods. They can greatly improve the efficiency of amylase, trypsin and lipase detection in human serum and urine samples, providing great assistance in the early diagnosis of AP. The rapid and accurate detection of triglycerides and serum creatinine is of great help to the etiological diagnosis and prognosis of acute pancreatitis. Gd based nanomaterials can be used for enhanced contrast in MRI diagnosis of early AP, which can compensate for the insensitivity of CT diagnosis.
For decades, the exact pathophysiology of acute pancreatitis has been uncertain, except for the recognition that it may be an autoimmune digestive disease. Extensive work on animal models has revealed several critical pathophysiological mechanisms that may represent therapeutic targets. Nanomaterials exert protective effects by inhibiting trypsin secretion, reducing reactive oxygen species production, anti-oxidative stress, anti-inflammatory factors, and ER stress, by upregulating Nrf2, SOD1, and NQO1, and downregulating inducible nitric oxide synthase, p65 NF-κB, Hsp27, and Hsp70. In addition, NPs can also be used as anti-inflammatory drugs and gene transporters to treat AP. It is better than antibiotics alone in antibacterial and anti-infection therapy.
Although partial progress has been made, nanomaterials are still quite far from widespread use in clinics. The detection of ex vivo samples is initially carried out in clinics. A serious issue is the safety of nanomaterials in living organisms (especially humans). Although numerous in vitro and animal experiments have demonstrated the safety of nanomaterials, their potential threat remains unknown. There is an urgent need to develop nanomaterials that are more compatible and safer for living organisms, and to find suitable targets for the development of targeted nanomaterials through more mechanistic studies. In addition, there are nanomaterials for pancreatic tissue reconstruction and protection after acute pancreatitis waiting to be developed.
Author contributions
Conceptualization, W. J. and L. X.; writing – original draft preparation, W. J. and L. X.; writing – review and editing, W. J, L. X. and W. X.; suggestions on revision, Y. M.; funding acquisition, Y. Z. All authors have read and agreed to the published version of the manuscript. All authors have contributed substantially to the work reported.
Conflicts of interest
There are no conflicts to declare.
Acknowledgements
This work was funded by the National Natural Science Foundation of China, grant number 81872255, 62041101, and the Key Medical Talents Foundation of Jiangsu Province, grant number 2016KJQWZDRC-03.
References
- L. Boxhoorn, R. P. Voermans, S. A. Bouwense, M. J. Bruno, R. C. Verdonk, M. A. Boermeester, H. C. van Santvoort and M. G. Besselink, Lancet, 2020, 396, 726–734 CrossRef.
- N. J. Schepers, O. J. Bakker, M. G. Besselink, U. Ahmed Ali, T. L. Bollen, H. G. Gooszen, H. C. van Santvoort and M. J. Bruno, Gut, 2019, 68, 1044–1051 CrossRef CAS PubMed.
- S. van Dijk, N. Hallensleben, H. van Santvoort, P. Fockens, H. van Goor, M. Bruno and M. Besselink, Gut, 2017, 66, 2024–2032 CrossRef CAS PubMed.
- S. E. Roberts, S. Morrison-Rees, A. John, J. G. Williams and D. G. Samuel, Pancreatology, 2017, 17, 155 CrossRef PubMed.
- W. G. I. A. A. P. Guidelines and J. D. Waele, Pancreatology, 2013, 13, e1–e15 CrossRef PubMed.
- M. Bhatia, Br. J. Surg., 2010, 82, 6–13 Search PubMed.
- S. Pandol, A. Saluja, C. Imrie and P. Banks, Gastroenterology, 2007, 132, 1127–1151 CrossRef CAS PubMed.
- C. Popa, J. Med. Life, 2014, 7, 525–528 CAS.
- P. J. Lee and G. I. Papachristou, Nat. Rev. Gastroenterol. Hepatol., 2019, 16, 479–496 CrossRef PubMed.
- A. Aghdassi, D. John, M. Sendler, F. Weiss, T. Reinheckel, J. Mayerle and M. Lerch, J. Biol. Chem., 2018, 293, 1018–1029 CrossRef CAS PubMed.
- J. Gerasimenko, O. Gryshchenko, P. Ferdek, E. Stapleton, T. Hébert, S. Bychkova, S. Peng, M. Begg, O. Gerasimenko and O. Petersen, Proc. Natl. Acad. Sci. U. S. A., 2013, 110, 13186–13191 CrossRef CAS.
- R. Mukherjee, O. Mareninova, I. Odinokova, W. Huang, J. Murphy, M. Chvanov, M. Javed, L. Wen, D. Booth, M. Cane, M. Awais, B. Gavillet, R. Pruss, S. Schaller, J. Molkentin, A. Tepikin, O. Petersen, S. Pandol, I. Gukovsky, D. Criddle, A. Gukovskaya and R. Sutton, Gut, 2016, 65, 1333–1346 CrossRef CAS PubMed.
- Y. Zeng, X. Wang, W. Zhang, K. Wu and J. Ma, Hepato-Gastroenterology, 2012, 59, 2318–2326 CAS.
- A. Lugea, D. Tischler, J. Nguyen, J. Gong, I. Gukovsky, S. French, F. Gorelick and S. Pandol, Gastroenterology, 2011, 140, 987–997 CrossRef CAS PubMed.
- L. Antonucci, J. Fagman, J. Kim, J. Todoric, I. Gukovsky, M. Mackey, M. Ellisman and M. Karin, Proc. Natl. Acad. Sci. U. S. A., 2015, 112, E6166–E6174 CrossRef CAS PubMed.
- M. Sendler, A. Dummer, F. Weiss, B. Krüger, T. Wartmann, K. Scharffetter-Kochanek, N. van Rooijen, S. Malla, A. Aghdassi, W. Halangk, M. Lerch and J. Mayerle, Gut, 2013, 62, 430–439 CrossRef CAS PubMed.
- W. Guidelines and M. M. Lerch, Pancreatology, 2013, 4, e1–15 Search PubMed.
- P. A. Banks, T. L. Bollen, C. Dervenis, H. G. Gooszen, C. D. Johnson, M. G. Sarr, G. G. Tsiotos and S. S. Vege, Gut, 2012, 62, 102–111 CrossRef PubMed.
- H. van Santvoort, O. Bakker, T. Bollen, M. Besselink, U. Ahmed Ali, A. Schrijver, M. Boermeester, H. van Goor, C. Dejong, C. van Eijck, B. van Ramshorst, A. Schaapherder, E. van der Harst, S. Hofker, V. Nieuwenhuijs, M. Brink, P. Kruyt, E. Manusama, G. van der Schelling, T. Karsten, E. Hesselink, C. van Laarhoven, C. Rosman, K. Bosscha, R. de Wit, A. Houdijk, M. Cuesta, P. Wahab and H. Gooszen, Gastroenterology, 2011, 141, 1254–1263 CrossRef.
- K. S. Gurusamy, A. P. Belgaumkar, A. Haswell, S. P. Pereira and B. R. Davidson, Cochrane Database of Systematic Reviews, 2016, 109, 1176–1194 Search PubMed.
- J. Y. Bang, C. M. Wilcox, J. P. Arnoletti and S. Varadarajulu, Dig. Endosc., 2020, 32, 298–308 CrossRef PubMed.
- Y. Marlida, N. Saari, Z. Hassan, S. Radu and J. Bakar, Food Chem., 2000, 71, 221–227 CrossRef CAS.
- J. Qin, K. Li, C. Peng, X. Li, J. Lin, K. Ye, X. Yang, Q. Xie, Z. Shen, Y. Jin, M. Jiang, G. Zhang and X. Lu, Biomaterials, 2013, 34, 4914–4925 CrossRef CAS.
- A. Raza, F. B. Sime, P. J. Cabot, J. A. Roberts, J. R. Falconer, T. Kumeria and A. Popat, ACS Biomater. Sci. Eng., 2021, 7, 1836–1853 CrossRef CAS.
- O. A. Madkhali, S. Sivagurunathan Moni, M. H. Sultan, H. A. Bukhary, M. Ghazwani, N. A. Alhakamy, A. M. Meraya, S. Alshahrani, S. S. Alqahtani, M. A. Bakkari, M. I. Alam and M. E. Elmobark, Sci. Rep., 2021, 11, 9914 CrossRef CAS PubMed.
- K. Parekh, K. Hariharan, Z. Qu, P. Rewatkar, Y. Cao, M. Moniruzzaman, P. Pandey, A. Popat and T. Mehta, Int. J. Pharm., 2021, 608, 121079 CrossRef CAS PubMed.
- W. Xu, X. Qing, S. Liu, Z. Chen and Y. Zhang, J. Mater. Chem. B, 2022, 10, 1343–1358 RSC.
- B. Sun, X. Zhen and X. Jiang, Biomater. Sci., 2021, 9, 3603–3620 RSC.
- G. Liu, S. Zhang, Y. Shi, X. Huang, Y. Tang, P. Chen, W. Si, W. Huang and X. Dong, Adv. Funct. Mater., 2018, 28, 1804317 CrossRef.
- Q. Tang, W. Si, C. Huang, K. Ding, W. Huang, P. Chen, Q. Zhang and X. Dong, J. Mater. Chem. B, 2017, 5, 1566–1573 RSC.
- N. Yang, H. Guo, C. Cao, X. Wang, X. Song, W. Wang, D. Yang, L. Xi, X. Mou and X. Dong, Biomaterials, 2021, 275, 120918 CrossRef CAS PubMed.
- D. Chen, Y. Tang, J. Zhu, J. Zhang and X. Dong, Biomaterials, 2019, 221, 119422 CrossRef CAS PubMed.
- R. Yang, M. Hou, Y. Gao, L. Zhang, Z. Xu, Y. Kang and P. Xue, Nanoscale, 2019, 11, 5717–5731 RSC.
- D. Chen, Q. Tang, J. Zou, X. Yang, W. Huang, Q. Zhang, J. Shao and X. Dong, Adv. Healthcare Mater., 2018, 7, e1701272 CrossRef PubMed.
- P. Liang, Q. Tang, Y. Cai, G. Liu, W. Si, J. Shao, W. Huang, Q. Zhang and X. Dong, Chem. Sci., 2017, 8, 7457–7463 RSC.
- A. Gour and N. K. Jain, Artif. Cells, 2019, 47, 844–851 CAS.
- S. Zhang, Q. Li, N. Yang, Y. Shi and X. Dong, Adv. Funct. Mater., 2019, 29, 1906805 CrossRef CAS.
- W. Xu, X. Qing, S. Liu, D. Yang, X. Dong and Y. Zhang, Small, 2022, e2106511, DOI:10.1002/smll.202106511.
- M. Patil, D. S. Mehta and S. Guvva, J. Indian Soc. Periodontol., 2008, 12, 34–40 CrossRef PubMed.
- J. Zou, P. Wang, Y. Wang, G. Liu, Y. Zhang, Q. Zhang, J. Shao, W. Si, W. Huang and X. Dong, Chem. Sci., 2019, 10, 268–276 RSC.
- S. Zhang, C. Cao, X. Lv, H. Dai, Z. Zhong, C. Liang, W. Wang, W. Huang, X. Song and X. Dong, Chem. Sci., 2020, 11, 1926–1934 RSC.
- C. J. Lech and A. T. Phan, Nucleic Acids Res., 2017, 45, 6265–6274 CrossRef CAS PubMed.
- M. d'Amora, A. Camisasca, A. Boarino, S. Arpicco and S. Giordani, Colloids Surf., B, 2020, 188, 110779 CrossRef PubMed.
- Y. Wang, X. Huang, Y. Tang, J. Zou, P. Wang, Y. Zhang, W. Si, W. Huang and X. Dong, Chem. Sci., 2018, 9, 8103–8109 RSC.
- W. Xiao, P. Wang, C. Ou, X. Huang, Y. Tang, M. Wu, W. Si, J. Shao, W. Huang and X. Dong, Biomaterials, 2018, 183, 1–9 CrossRef CAS PubMed.
- J. Bariwal, H. Ma, G. A. Altenberg and H. Liang, Chem. Soc. Rev., 2022, 51, 1702–1728 RSC.
- J. Gao, Z. Wang, Q. Guo, H. Tang, Z. Wang, C. Yang, H. Fan, W. Zhang, C. Ren and J. Liu, Theranostics, 2022, 12, 1286–1302 CrossRef CAS PubMed.
- H. Lv, T. Wang, F. Ma, K. Zhang, T. Gao, R. Pei and Y. Zhang, Biomed. Mater., 2022, 17, 024108 CrossRef PubMed.
- H. Fang, Y. Sha, L. Yang, J. Jiang, L. Yin, J. Li, B. Li, B. Klumperman, Z. Zhong and F. Meng, ACS Appl. Mater. Interfaces, 2022, 14, 8824–8837 CrossRef PubMed.
- W. R. Matull, S. P. Pereira and J. W. O'Donohue, J. Clin. Pathol., 2006, 59, 340–344 CrossRef CAS PubMed.
- M. S. Attia and N. S. Al-Radadi, Biosens. Bioelectron., 2016, 86, 413–419 CrossRef CAS PubMed.
- J. Shi, Q. Deng, Y. Li, M. Zheng, Z. Chai, C. Wan, Z. Zheng, L. Li, F. Huang and B. Tang, Anal. Chem., 2018, 90, 13775–13782 CrossRef CAS PubMed.
- N. Mandal, M. Bhattacharjee, A. Chattopadhyay and D. Bandyopadhyay, Biosens. Bioelectron., 2019, 124–125, 75–81 CrossRef CAS PubMed.
- A. Saluja, V. Dudeja, R. Dawra and R. P. Sah, Gastroenterology, 2019, 156, 1979–1993 CrossRef CAS PubMed.
- A.-M. Coelho, V. Ossovskaya and W. N. Bunnett, Curr. Med. Chem.: Cardiovasc. Hematol. Agents, 2003, 1, 61–72 CAS.
- L. Hu, S. Han, S. Parveen, Y. Yuan, L. Zhang and G. Xu, Biosens. Bioelectron., 2012, 32, 297–299 CrossRef CAS PubMed.
- C. Y. Poon, Q. Li, J. Zhang, Z. Li, C. Dong, A. W. Lee, W. H. Chan and H. W. Li, Anal. Chim. Acta, 2016, 917, 64–70 CrossRef CAS PubMed.
- E. Lucas, R. Knoblauch, M. Combs-Bosse, S. E. Broedel Jr and C. D. Geddes, Spectrochim. Acta, Part A, 2020, 228, 117739 CrossRef CAS PubMed.
- S. Hou, T. Feng, N. Zhao, J. Zhang, H. Wang, N. Liang and L. Zhao, J. Pharm. Anal., 2020, 10, 482–489 CrossRef PubMed.
- S. Guan, J. Yue, W. Sun, W. Xu, C. Liang and S. Xu, Anal. Bioanal. Chem., 2021, 413, 4939–4945 CrossRef CAS PubMed.
- S. Fedeli, J. Im, S. Gopalakrishnan, J. Elia, A. Gupta, D. Kim and V. Rotello, Chem. Soc. Rev., 2021, 50, 13467–13480 RSC.
- X. Lin, Z. Zhu, D. Lin, Q. Bao, Y. Gao, Q. Liu, A. Liu, L. Lin and X. Lin, Talanta, 2021, 234, 122647 CrossRef CAS PubMed.
- M. Kylänpääbäck, E. Kemppainen, P. Puolakkainen, J. Hedström, R. Haapiainen, A. Korvuo and U. H. Stenman, Gastroenterology, 2002, 118, A162 CrossRef.
- S. Z. Husain and A. I. Srinath, Nat. Rev. Gastroenterol. Hepatol., 2017, 14, 366–372 CrossRef PubMed.
- D. Chakraborty, D. Sarkar, A. K. Ghosh and P. K. Das, Soft Matter, 2021, 17, 2170–2180 RSC.
- J. Shi, Q. Deng, C. Wan, M. Zheng, F. Huang and B. Tang, Chem. Sci., 2017, 8, 6188–6195 RSC.
- C. S. Pundir and V. Narwal, Biosens. Bioelectron., 2018, 100, 214–227 CrossRef CAS PubMed.
- A. Murali, G. Lokhande, K. Deo, A. Brokesh and A. Gaharwar, Mater. Today, 2021, 50, 276–302 CrossRef CAS PubMed.
- A. K. Gupta, A. Kaushik, P. R. Solanki, C. Dhand, S. Bhansali and B. D. Malhotra, Nanosci. Lett., 2014, 4, 3–7 Search PubMed.
- S. Solanki, C. M. Pandey, A. Soni, G. Sumana and A. M. Biradar, Microchim. Acta, 2016, 183, 167–176 CrossRef CAS.
- J. Narang and C. S. Pundir, Int. J. Biol. Macromol., 2011, 49, 707–715 CrossRef CAS PubMed.
- A. Di Tocco, S. N. Robledo, Y. Osuna, J. Sandoval-Cortez, A. M. Granero, N. R. Vettorazzi, J. L. Martinez, E. P. Segura, A. Ilina, M. A. Zon, F. J. Arevalo and H. Fernandez, Talanta, 2018, 190, 30–37 CrossRef CAS PubMed.
- V. Muddana, D. C. Whitcomb, A. Khalid, A. Slivka and G. I. Papachristou, Am. J. Gastroenterol., 2009, 104, 164–170 CrossRef CAS PubMed.
- P. G. Lankisch, B. Weber-Dany, P. Maisonneuve and A. B. Lowenfels, Am. J. Gastroenterol., 2010, 105, 1196–1200 CrossRef CAS PubMed.
- P. Wen, F. Yang, C. Ge, S. Li, Y. Xu and L. Chen, Nanotechnology, 2021, 32, 395502 CrossRef CAS PubMed.
- M. Zhybak, V. Beni, M. Y. Vagin, E. Dempsey, A. P. Turner and Y. Korpan, Biosens. Bioelectron., 2016, 77, 505–511 CrossRef CAS PubMed.
- G. Gravante, G. Garcea, S. L. Ong, M. S. Metcalfe, D. P. Berry, D. M. Lloyd and A. R. Dennison, Pancreatology, 2009, 9, 601–614 CrossRef CAS PubMed.
- H. Xing, W. Bu, S. Zhang, X. Zheng, M. Li, F. Chen, Q. He, L. Zhou, W. Peng and Y. Hua, Biomaterials, 2012, 33, 1079–1089 CrossRef CAS PubMed.
- R. Pezzilli, Expert Opin. Pharmacother., 2009, 10, 2999–3014 CrossRef CAS.
- S. J. Mcpherson, D. A. O'Reilly, M. T. Sinclair and N. Smith, Br. J. Radiol., 2017, 90, 20170224 CrossRef PubMed.
- H. W. Zhang, L. Q. Wang, Q. F. Xiang, Q. Zhong, L. M. Chen, C. X. Xu, X. H. Xiang, B. Xu, F. Meng, Y. Q. Wan and D. Y. Deng, Biomaterials, 2014, 35, 356–367 CrossRef CAS PubMed.
- L. Long, L. Deng, L. Wang, S. Wen, L. Luo, L. Liang, L. Ding, J. Wu, Z. Ye and D. Y. B. Deng, ACS Biomater. Sci. Eng., 2020, 6, 5857–5865 CrossRef CAS PubMed.
- R. J. Holbrook, N. Rammohan, M. W. Rotz, K. W. MacRenaris, A. T. Preslar and T. J. Meade, Nano Lett., 2016, 16, 3202–3209 CrossRef CAS PubMed.
- P. Banks, T. Bollen, C. Dervenis, H. Gooszen, C. Johnson, M. Sarr, G. Tsiotos and S. Vege, Gut, 2013, 62, 102–111 CrossRef.
- F. Gorelick and M. Lerch, Cell. Mol. Gastroenterol. Hepatol., 2017, 4, 251–262 CrossRef PubMed.
- M. Lerch and F. Gorelick, Gastroenterology, 2013, 144, 1180–1193 CrossRef PubMed.
- R. Dawra, R. Sharif, P. Phillips, V. Dudeja and A. K. Saluja, Am. J. Physiol.: Gastrointest. Liver Physiol., 2007, 292, G1009–G1018 CrossRef CAS PubMed.
- A. Gukovskaya, E. Vaquero, V. Zaninovic, F. Gorelick, A. Lusis, M. Brennan, S. Holland and S. Pandol, Gastroenterology, 2002, 122, 974–984 CrossRef CAS PubMed.
- S. R. Dai, L. Zhen and J. B. Zhang, Int. J. Artif. Organs, 2015, 38, 192 CrossRef CAS PubMed.
- E. Fisic, G. Poropat, L. Bilic-Zulle, V. Licul and D. Stimac, Gastroenterol. Res. Pract., 2013, 2013, 282645 CAS.
- S. Pérez, J. Pereda, L. Sabater and J. Sastre, Redox Biol., 2015, 5, 1–14 CrossRef PubMed.
- S. Cuzzocrea, E. Mazzon, L. Dugo, I. Serraino, T. Centorrino, A. Ciccolo, D. Van, D. Britti, A. P. Caputi and C. Thiemermann, Shock, 2002, 17, 416–422 CrossRef PubMed.
- P. Santofimia-Castano, D. Clea Ruy, L. Garcia-Sanchez, D. Jimenez-Blasco, M. Fernandez-Bermejo, J. Bolaños, G. Salido and A. Gonzalez, Free Radicals Biol. Med., 2015, 87, 226–236 CrossRef CAS PubMed.
- A. Dabrowski, J. Osada, M. I. Dabrowska, U. Wereszczynska-Siemiatkowska and A. Siemiatkowski, Adv. Med. Sci., 2014, 59, 102–107 CrossRef PubMed.
- R. T. Ethridge, R. A. Ehlers, M. R. Hellmich, S. Rajaraman and B. M. Evers, Pancreas, 2000, 21, 248–256 CrossRef CAS PubMed.
- J. Sandoval, J. Pereda, J. L. Rodriguez, J. Escobar, J. Hidalgo, L. Joosten, L. Franco, J. Sastre and G. López-Rodas, Expert Opin. Pharmacother., 2010, 67, 1687–1697 CAS.
- A. Khurana, P. Anchi, P. Allawadhi, V. Kumar, N. Sayed, G. Packirisamy and C. Godugu, Nanomedicine, 2019, 14, 1805–1825 CrossRef CAS PubMed.
- A. Khurana, P. Anchi, P. Allawadhi, V. Kumar, N. Sayed, G. Packirisamy and C. Godugu, Nanomedicine, 2019, 18, 54–65 CrossRef CAS PubMed.
- Q. Yao, X. Jiang, Y. Y. Zhai, L. Z. Luo, H. L. Xu, J. Xiao, L. Kou and Y. Z. Zhao, J. Controlled Release, 2020, 322, 312–325 CrossRef CAS PubMed.
- E. L. Bradley, Am. J. Surg., 1989, 158, 472–477 CrossRef PubMed ; discussion 477–478..
- X. Zhou, X. Cao, H. Tu, Z. R. Zhang and L. Deng, Mol. Pharm., 2019, 16, 1397–1405 CrossRef CAS PubMed.
- Y. Tang, Y. Han, L. Liu, W. Shen, H. Zhang, Y. Wang, X. Cui, Y. Wang, G. Liu and R. Qi, Biomacromolecules, 2015, 16, 174–182 CrossRef CAS PubMed.
- E. A. Abdel-Hakeem, H. A. Abdel-Hamid and S. M. N. Abdel Hafez, J. Trace Elem. Med. Biol., 2020, 60, 126480 CrossRef CAS PubMed.
- O. A. R. Abozaid, F. S. M. Moawed, E. S. A. Ahmed and Z. A. Ibrahim, Biochim. Biophys. Acta, Mol. Basis
Dis., 2020, 1866, 165904 CrossRef CAS PubMed.
- J. J. Fan, Q. X. Mei, G. Y. Deng, Z. H. Huang, Y. Fu, J. H. Hu, C. L. Huang, Y. Y. Lu, L. G. Lu, X. P. Wang and Y. Zeng, J. Dig. Dis., 2021, 22, 363–372 CrossRef CAS PubMed.
- P. Hassanzadeh, E. Arbabi and F. Rostami, Life Sci., 2021, 270, 119128 CrossRef CAS PubMed.
- X. Xu, J. Duan, Y. Liu, Y. Kuang, J. Duan, T. Liao, Z. Xu, B. Jiang and C. Li, Acta Biomater., 2021, 126, 445–462 CrossRef CAS PubMed.
- C. Wang, Y. Xiao, W. Zhu, J. Chu, J. Xu, H. Zhao, F. Shen, R. Peng and Z. Liu, Small, 2020, 16, e2000589 CrossRef PubMed.
- Y. Cheng, Y. Zhang, W. Deng and J. Hu, Colloids Surf., B, 2020, 186, 110744 CrossRef CAS PubMed.
- C. Cao, W. Ge, J. Yin, D. Yang, W. Wang, X. Song, Y. Hu, J. Yin and X. Dong, Small, 2020, 16, e2000436 CrossRef PubMed.
- Y. Tao, J. Wang and X. Xu, Frontiers in Bioengineering and Biotechnology, 2020, 8, 184 CrossRef PubMed.
- J. Liu, L. Cui and D. Losic, Acta Biomater., 2013, 9, 9243–9257 CrossRef CAS PubMed.
- M. Serda, G. Szewczyk, O. Krzysztynska-Kuleta, J. Korzuch, M. Dulski, R. Musiol and T. Sarna, ACS Biomater. Sci. Eng., 2020, 6, 5930–5940 CrossRef CAS PubMed.
- X. J. Liang, H. Meng, Y. Wang, H. He, J. Meng, J. Lu, P. C. Wang, Y. Zhao, X. Gao, B. Sun, C. Chen, G. Xing, D. Shen, M. M. Gottesman, Y. Wu, J. J. Yin and L. Jia, Proc. Natl. Acad. Sci. U. S. A., 2010, 107, 7449–7454 CrossRef CAS PubMed.
- F. Kesisoglou, S. Panmai and Y. Wu, Adv. Drug Delivery Rev., 2007, 59, 631–644 CrossRef CAS PubMed.
- E. Y. Chuang, K. J. Lin, T. Y. Huang, H. L. Chen, Y. B. Miao, P. Y. Lin, C. T. Chen, J. H. Juang and H. W. Sung, ACS Nano, 2018, 12, 6389–6397 CrossRef CAS PubMed.
- K. Taguchi, S. Nagao, H. Maeda, H. Yanagisawa, H. Sakai, K. Yamasaki, T. Wakayama, H. Watanabe, M. Otagiri and T. Maruyama, Drug Delivery, 2018, 25, 1266–1274 CrossRef CAS PubMed.
- K. Kamimura, T. Yokoo and S. Terai, Int. J. Mol. Sci., 2018, 19, 3415 CrossRef PubMed.
- K. N. Westlund, Gene Ther., 2009, 16, 483–492 CrossRef CAS PubMed.
- G. Seifert, R. P. Kurzinger, U. T. Hopt and U. A. Wittel, J. Surg. Res., 2013, 180, e83–e90 CrossRef CAS.
- W. G. Zou, D. S. Wang, M. F. Lang, D. Y. Jin and X. Y. Liu, J. Surg. Res., 2002, 103, 121–126 CrossRef CAS PubMed.
- W. Zou, D. Wang, M. Lang, D. Jin, D. Xu, Z. Zheng, Z. Wu and X. Liu, J. Surg. Res., 2002, 103, 121–126 CrossRef CAS PubMed.
- H. Rasmussen, O. Irtun, S. Olesen, A. Drewes and M. Holst, World J. Gastroenterol., 2013, 19, 7267–7275 CrossRef PubMed.
- C. Yang, T. Hu, H. Cao, L. Zhang, P. Zhou, G. He, X. Song, A. Tong, G. Guo, F. Yang, X. Zhang, Z. Qian, X. Qi, L. Zhou and Y. Zheng, Mol. Pharm., 2015, 12, 2167–2179 CrossRef CAS PubMed.
- S. M. V. Dijk, N. D. L. Hallensleben, H. C. V. Santvoort, P. Fockens and M. G. Besselink, Gut, 2017, 66, 2024–2032 CrossRef PubMed.
- M. Werge, S. Novovic, P. N. Schmidt and L. L. Gluud, Pancreatology, 2016, 698–707 CrossRef PubMed.
- H. Zhou, J. Gao, W. Wu, L. Liu, L. Wei and D. Zou, Pancreas, 2011, 40, 1226–1233 CrossRef CAS PubMed.
- N. L. Horst, R. G. Marques, C. F. Diestel, B. D. Matzke, C. Caetano, F. C. Simões, A. Andrade, W. I. Lobão, L. Vaz and M. C. J. C. T. R. C. Portela, Experimental, 2009, 70, 136–148 Search PubMed.
- N. Mowbray, B. Ben-Ismaeil, M. Hammoda, G. Shingler and B. Al-Sarireh, Hepatobiliary Pancreatic Dis. Int., 2018, 17, 456–460 CrossRef PubMed.
- P. Moka, P. Goswami, A. Kapil, I. Xess, V. Sreenivas and A. Saraya, Pancreas, 2018, 47, 489 CrossRef PubMed.
- J. Ali, Q. A. Rafiq and E. Ratcliffe, Future Sci. OA, 2018, 4, FSO290 CrossRef CAS PubMed.
- P. V. Asharani, H. M. Prakash and S. Valiyaveettil, BMC Cell Biol., 2009, 10, 65 CrossRef CAS PubMed.
- J. Kim, E. Kuk, K. Yu, J. Kim, S. Park, H. Lee, S. Kim, Y. Park, Y. Park, C. Hwang, Y. Kim, Y. Lee, D. Jeong and M. Cho, Nanomedicine, 2007, 3, 95–101 CrossRef CAS PubMed.
- B. Zhao, H. Wang, W. Dong, S. Cheng, H. Li, J. Tan, J. Zhou, W. He, L. Li, J. Zhang, G. Luo and W. Qian, J. Nanobiotechnol., 2020, 18, 137 CrossRef PubMed.
- Z. Wang, Y. Peng, Y. Zhou, S. Zhang, J. Tan, H. Li, D. He and L. Deng, Acta Biomater., 2021, 137, 276–289 CrossRef PubMed.
- S. Sharma, N. Chakraborty, D. Jha, H. K. Gautam and I. Roy, Mater. Sci. Eng., C, 2020, 113, 110982 CrossRef CAS PubMed.
- Z. Su, D. Sun, L. Zhang, M. He, Y. Jiang, B. Millar, P. Douglas, D. Mariotti, P. Maguire and D. Sun, Materials, 2021, 14, 2351 CrossRef CAS PubMed.
- R. R. Castillo and M. Vallet-Regi, Int. J. Nanomed., 2021, 16, 4409–4430 CrossRef PubMed.
- L. Ge, Q. Hu, J. Chen, M. Shi, H. Yang and G. Zhu, Mater. Sci. Eng., C, 2017, 77, 405–410 CrossRef CAS PubMed.
- Y. Wu, W. Jiang, S. Huo, S. Li, Y. Xu, S. Ding, J. Zhou, H. Liu, W. Lv and Y. Wang, Biomaterials, 2021, 279, 121237 CrossRef CAS PubMed.
Footnote |
† Weilu Jia and Linfeng Xu contributed equally to this work. |
|
This journal is © The Royal Society of Chemistry 2022 |