DOI:
10.1039/D2MA00074A
(Paper)
Mater. Adv., 2022,
3, 5406-5417
Density effects of vertical graphene nanowalls on supercapacitor performance
Received
24th January 2022
, Accepted 17th May 2022
First published on 18th May 2022
1. Introduction
The rapid development of portable and electronic devices, including an integrated circuit and a micro-electromechanical system, requires readily accessible electrical power sources and demands energy storage systems with low cost, high energy and power density, and excellent cycling performance.1,2 Among numerous energy storage devices, supercapacitors are considered to be suitable power sources to fulfill the demands for the modern world due to their elevated energy density, excellent rate capability and long cycling life.3 Graphene nanowalls, with the characteristics of high electrical conductivity, high surface area and two-dimensional structures, are promising and appropriate scaffolds to construct supercapacitor electrodes.4–6 Meanwhile, pseudocapacitive materials, such as MnO2, ZnO, RuO2, and PANI, are being explored to improve the electrochemical performance for supercapacitors, which show excellent energy storage capability compared to EDLCs due to the energy-storage mechanism involving a faradaic redox reaction.7,8 Among those materials, MnO2 is considered to be one of the suitable transition metal oxides comprising supercapacitor electrodes because of the low cost, environmental friendliness, and high intrinsic capacitance (1370 F g−1).4,9–13 Meanwhile, the supercapacitor electrodes based on metal–MnO2 materials exhibit excellent electrochemical performance compared to MnO2-based pseudocapacitive materials.14–16 However, the poor electrical conductivity of MnO2 results in slow ion transfer rates and a surface redox reaction containing a very thin layer involved in the charge–discharge procedure, limiting the utilization of MnO2-based supercapacitor electrodes.17–19 Therefore, the MnO2/graphene composites have been widely investigated as the most promising candidates for supercapacitor electrodes. For example, a graphene oxide/MnO2/Ni foam composite via a mild fabrication method with a specific capacitance of 288 F g−1 at a current density of 0.5 A g−1 has been synthesized.20 The MnO2/graphene fabricated using a one-step hydrothermal synthesis exhibits a specific capacitance of 255 F g−1 at 0.5 A g−1 with a capacitance retention of 84.5% after 10
000 cycles.21 The needle-like structure of MnO2 coated on GO is formed, showing improved performance compared with the other structures of MnO2.22 An exfoliated graphene paper deposited by MnO2 nanoflowers with a specific capacitance of 385.2 F g−1 at 1 mV s−1 is reported. Meanwhile, the supercapacitor device with graphene paper/MnO2 as the positive electrode and graphene as the negative electrode is assembled, showing an area-normalized specific capacitance of 76.8 mF cm−2 at 0.05 mA cm−2 and a capacitance retention of 82.2% after 5000 cycles.23 The MnO2/graphene composites with the freestanding structures require the current collectors (e.g., Ni foam or PET) and binders (e.g., PVDF) to enhance the robustness of the fabricated electrodes. However, the aggregation of graphene materials jeopardizes the electrochemical performance of supercapacitor electrodes.24–26 More importantly, the poor controllability over the microstructures of the graphene scaffolds supporting and dispersing the active pseudocapacitive materials increases the difficulty of investigating the morphology and density effects on the performance of supercapacitor electrodes. The vertical graphene nanowalls deposited directly on substrates, followed by the coatings of pseudocapacitive materials, have been synthesized as supercapacitor electrodes with the highly controllable microstructures and well-dispersed nanowalls.5,27–33 Yu et al. have reported that the vertical graphene-based supercapacitor electrodes show a superior performance compared with lateral graphene structures.34 The VG nanowalls can be formed with a highly tunable morphology and density, which provides access to investigate the modifiable scaffolds influencing the performance of supercapacitor electrodes. Nevertheless, the density effects of graphene scaffolds on the electrochemical performance of supercapacitor electrodes have not been systematically studied. To investigate the density effects of scaffolds, the deposition of pseudocapacitive materials with highly distributed structures on vertical graphene nanowalls by using water–ethanol solvents to prepare electrolytes, instead of depositing pseudocapacitive materials on the top sides of vertical graphene using water-based electrolytes, have been made in our previous work.33
In this work, the vertical graphene nanowalls with controllable densities by adjusting the flow ratios of CH4
:
H2 are fabricated on silicon wafer substrates as supercapacitor electrodes via plasma-enhanced chemical vapor deposition (PECVD), followed by the electrochemical deposition of MnO2 under a constant condition. The silicon wafers are applied as the substrates with the advantages of (1) the ideally flat surface with easily observed status eliminates the roughness effects on the growth of VG; (2) the natural SiO2 nano-layers on the surface of silicon wafers enhance the bonding intensity of VG with substrates; (3) the MnO2/VG/Si constructions provide a novel approach for the widespread applications in the electronic and semiconductor fields.33 Although the manganese dioxide possesses a lower theoretical specific capacitance among the other types of metal oxides, such as RuO2 (1400–2000 F g−1), and Co3O4 (3560 F g−1), it has tunable microstructures and higher potential windows (∼1 V) than those of RuO2 and Co3O4.19,33,35,36 The improved distributions of MnO2 depositions achieved by using a mixture of ethanol and distilled water as the solvent facilitate the dispersion of MnO2 on VG nanowalls, which clearly reveal the density effects of VG scaffolds. The supercapacitor electrode with the medium-density of VG nanowalls shows the largest area-normalized specific capacitance among all the electrodes. Subsequently, the asymmetric supercapacitor device with MnO2/VG/Si as the positive electrode and carbon black as the negative electrode is assembled. The carbon black nanoparticles with a diameter of ∼30 nm possess the advantages of (1) high electric conductivity, (2) high surface area, and (3) nanostructures corresponding to the positive electrodes with VG nanowalls. The device demonstrates a greatly superior electrochemical performance and has strong potential for the applications powering micro-sensors or electronics.
2. Experimental method
2.1 Preparation of electrodes consisting of VG and MnO2 on silicon wafer substrates
The VG nanowalls with tunable densities were deposited via microwave plasma-enhanced chemical vapor deposition (MPECVD, Seki-AX5200S) on silicon wafer substrates by adjusting the flow ratios of CH4
:
H2. Briefly, the silicon wafers were loaded into the deposition chamber vacuumed for 7 hours before the deposition procedure. Then, the gas of H2 was introduced into the chamber under the plasma power at 650 W, increasing the pressure to 6000 Pa. Subsequently, the gas of CH4 as the carbon source with the flow rate of 20 sccm was injected into the growing chamber to initiate the VG growth for 30 min. The flow rates of H2 were set to 20 sccm, 50 sccm, and 100 sccm, corresponding to the CH4
:
H2 ratios of 1
:
1, 1
:
2.5, and 1
:
5 with the identification of VG1:1, VG1:2.5, and VG1:5, respectively. Furthermore, the pseudocapacitive materials of MnO2 were electrodeposited on VG nanowalls by using a three-electrode system with the as-prepared VG, platinum foil, and Ag/AgCl as the working, counter, and reference electrodes. The electrodeposition was conducted under a constant voltage of 0.6 V for 400 s in all the as-prepared VGs by using the electrolyte containing 0.1 M of Mn (CHCOO)2 and 0.1 M of Na2SO4 dissolved in the mixture of ethanol and distilled water (ratio 2
:
3). The MnO2-coated VGs were then named MnO2/VG1:1, MnO2/VG1:2.5, and MnO2/VG1:5.
2.2 Characterization
The microstructures and morphologies of VGs and MnO2-coated VG composites were investigated using scanning electron microscopy (Hitachi SU70, FE-SEM). The powders scratched from the MnO2/VG-deposited silicon wafer substrates, dispersed in ethanol, and dropped onto copper grids, were observed using transmission electron microscopy (TEM, JEOL JEM-2100F). The chemical compositions and structures were analyzed by X-ray photoelectron spectroscopy (XPS).
The electrochemical measurements with the three-electrode system in a typical aqueous solution of 1 M Na2SO4 for a single electrode and two-electrode system for a full device were conducted by using an electrochemical workstation (HZ-7000, Hokuto). The MnO2/VG, platinum, and Ag/AgCl were used as working, counter, and reference electrodes in the three-electrode system. The evaluations of a single electrode under the working potential window of 0–1 V and the full cell in the potential range of 0–1.8 V were performed employing cyclic voltammetry (CV) and galvanostatic (GV) charge–discharge curves. The CV tests were carried out under the typical scan rates varying from 5 mV s−1 to 100 mV s−1. The GV curves were obtained with current densities between 0.5 mA cm−2 and 5 mA cm−2. Finally, electrochemical impedance spectroscopy (EIS) (ALS Model 7082E) was conducted in a frequency between 0.1 Hz and 500 kHz with the sinusoid potential of 5 mV for a single electrode and full cell.
3. Results and discussion
3.1 SEM and TEM of VG and MnO2-coated VG
The tunable density of vertical graphene adjusted by the operation parameters, such as gas flow rates, chamber pressure, temperature, etc., has been obtained in the previous literature.37–40 As shown in Fig. 1(a)–(c), the SEM images exhibit the surface morphologies of the as-deposited VG nanowalls, corresponding to VG1:1, VG1:2.5, and VG1:5, respectively. The uniform distributions with the decreasing density of the nanosheets can be observed when the CH4 concentration decreases from 50 at% to 16.67 at%, suggesting the good and controllable growth of VG nanowalls. The translucent nanosheets, observed under the acceleration voltage of an electron beam (10 kV), with the similar thickness in all VG depositions indicate the ultra-thin nanowalls and homogenous thickness on the whole layers, which agrees with the previous reports that increasing concentration of CH4 leads to the higher nucleation tendency generating the higher density of VG nanowalls with the constant thickness of nanosheets.37,41,42 Furthermore, the BET and BJH measurements were conducted to demonstrate the surface area and average pore size. The surface area values of VG1:1, VG1:2.5, and VG1:5 are 40.93 m2 g−1, 27.12 m2 g−1, and 21.61 m2 g−1, respectively. The decreases of surface area, with the decreasing amounts of deposited VG, correspond to the reducing densities from VG1:1 to VG1:5. The BJH results with the values of 12.59 nm, 19.58 nm, and 35.05 nm for VG1:1, VG1:2.5 and VG1:5, agree with the changes from denser microstructures for VG1:1 to a sparser morphology for VG1:5. A more detailed information on the characterization of VG nanowalls can be found in our previous work.33Fig. 1(a′)–(c′) show the surface morphologies of MnO2-coated VGs formed via electrochemical deposition on VG1:1, VG1:2.5, and VG1:5 under the same conditions under the deposition potential of 0.6 V for 400 s. The homogeneous distributions of MnO2-coated nanowalls with a larger thickness of around 600 nm in MnO2/VG1:1 (Fig. 1(a′)) and a smaller thickness of approximately 400 nm in MnO2/VG1:2.5 and MnO2/VG1:5 (Fig. 1(b′) and (c′)) suggest the uniform growth of MnO2 on the surface of nanowalls. The identical thicknesses of MnO2-coated nanowalls in MnO2/VG1:2.5 and MnO2/VG1:5 indicate the same deposition rate on each nanowalls. As shown in the BET results, the higher density of VG nanowalls gives the larger accessible surface area to increase the current density, which produces a higher amount of MnO2 depositions. The MnO2 depositions merging with the adjacent nanowalls result in the increasing thickness of nanowalls in MnO2/VG1:1 due to the higher density of VG1:1.
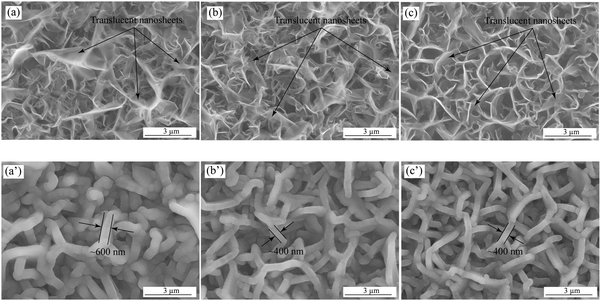 |
| Fig. 1 SEM images of as-deposited VG nanowalls of (a) VG1:1, (b) VG1:2.5, and (c) VG1:5; MnO2-coated VG nanowalls of (a′) MnO2/VG1:1, (b′) MnO2/VG1:2.5, and (c′) MnO2/VG1:5. | |
The TEM and HRTEM images illustrating the MnO2-coated VG, cross-sectional view of nanowalls, and crystals of MnO2 are shown in Fig. 2, which demonstrate the morphologies and microstructures of as-deposited MnO2 and VG in more detail. The MnO2-coated VG scratched from the substrates are dispersed in ethanol by ultrasonification treatment for preparing a TEM sample. The adhesive contacts of MnO2 on VG indicate the strong bonding between MnO2 and VG as shown in Fig. 2(a), which agrees with our previous investigations that the intimate adhesion is formed through the electrochemical deposition process.33 Furthermore, Fig. 2(b) shows the HRTEM images exhibiting the well-stacked layers ranging from 10 to 20 with the interplanar spacing of approximately 0.36 nm, which corresponds to the lattice constant of graphene, indicating the formation of graphene nanowalls.43 In addition, the layer distance of ∼0.43 nm observed in Fig. 2(c) conforms to the lattice parameter of β-MnO2, suggesting the formation of crystals of MnO2via electrochemical deposition.44,45 According to the HRTEM images, the VG nanowalls fabricated with a thickness ranging from ∼3 to ∼8 nm via the PECVD process are successfully achieved. Moreover, the interaction between MnO2 and VG nanowalls with robust bonding can improve the supercapacitor performance.
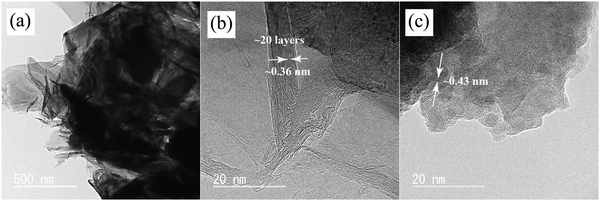 |
| Fig. 2 TEM and HRTEM images of MnO2/VG1:2.5: (a) MnO2-coated VG; (b) cross-section view of VG nanowalls; and (c) crystal structures of MnO2. | |
3.2 XPS and Raman analysis
XPS analysis is further conducted to reveal the elemental compositions and valence states of MnO2/VG1:2.5. As shown in Fig. 3(a), the broad-ranged XPS spectra exhibit the main peaks of Mn 2s, Mn 2p, O 1s, and C 1s, indicating the formation of carbon-based scaffolds and manganese oxide. In Fig. 3(b), the C 1s spectrum can be deconvoluted into three peaks centered at 284.6 eV, 286.1 eV, and 288.0 eV, corresponding to the bonding types of C–C, C
O, and O–C
O, respectively.46,47 The C–C bonding agrees with the SEM and TEM results, showing the successful growth of VG nanowalls.48 Furthermore, the two peaks deconvoluted from the O 1s high-resolution spectrum with the binding energy at the centers of 529.7 eV and 530.9 eV present the Mn–O–Mn and C–O bonds as shown in Fig. 3(c).49,50 Moreover, the spin energy separation of 11.7 eV in Mn 2p split into Mn 2p1/2 (654.6 eV) and Mn 2p3/2 (642.9 eV) suggests the presence of Mn4+, indicating the formation of MnO2via electrochemical methods.33,47
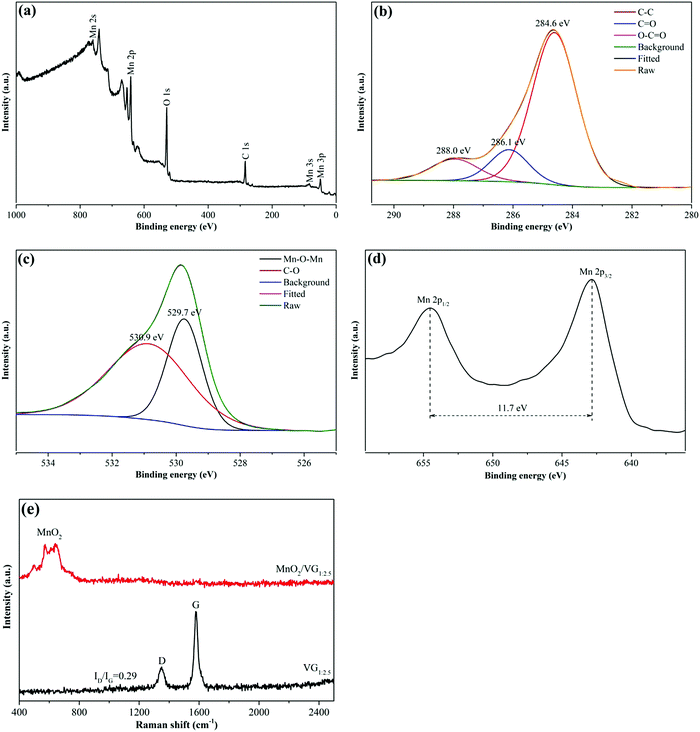 |
| Fig. 3 XPS spectra for MnO2/VG1:2.5: (a) survey spectrum; (b) C 1s; (c) O 1s; and (d) Mn 2p. (e) Raman spectra of MnO2/VG1:2.5 and VG1:2.5. | |
Fig. 3(e) shows the Raman spectra of VG1:2.5 and MnO2/VG1:2.5. The two distinct peaks, centered at 1347 cm−1 and 1577 cm−1 in VG1:2.5, assigned to D and G bands of graphene, exhibit the intensity ratio ID/IG of as low as 0.29, indicating the low level of disorder and defect degree of graphene and the formation of superior graphene nanowalls.31,51,52 The low-intensity ratio of ID/IG suggests the fabrication of graphene nanowalls with a smaller amount of sp3-type defects in agreement with our TEM images, which may lead to stronger mechanical and better electrical properties.20,52,53 Furthermore, the three peaks located at 502.1 cm−1, 571.7 cm−1, and 640.8 cm−1 in the range of 400 cm−1 and 800 cm−1 in MnO2/VG1:2.5 are attributed to the Mn–O stretching vibration, suggesting the successful formation of MnO2.20,54,55
3.3 Electrochemical properties of the MnO2/VG1:1, MnO2/VG1:2.5, and MnO2/VG1:5 electrodes
The CV and GV curves are obtained to evaluate the electrochemical performance of MnO2/VG1:1, MnO2/VG1:2.5, and MnO2/VG1:5 electrodes in a typical 1 M Na2SO4 aqueous solution with a three-electrode system operated in the potential window of 0–1 V. As shown in Fig. 4(a)–(c), the rectangular-shaped CV curves at scan rates from 5 mV s−1 to 100 mV s−1 for all the three electrodes suggest the excellent pseudocapacitive performance and faradaic redox reaction during the charge–discharge process.23,53 Meanwhile, the CV curves at the fast scan rate of 100 mV s−1 retain a quasi-rectangular configuration, indicating the good rate capacitance for all the three electrodes.19 The superior electrochemical behaviors are associated with the nanostructures composed of the VG nanowalls and highly dispersed MnO2 depositions as discussed in our previous study.33 For comparison, the CV curves for the three electrodes at a scan rate of 5 mV s−1 are illustrated in Fig. 3(d). The three CV curves belonging to the electrodes present different current densities from the negative to positive current flows. It is well-known that the integrated loop area of CV curves represents the specific capacitance of supercapacitor electrodes in ideal capacitive behaviors. For the cases of non-ideality, the forward scan may have a higher area than the reverse scan. Therefore, the calculation for specific capacitance in non-ideality can be made with the data from the discharging scan.56 In the lowest current density of CV curves for our three types of electrodes, the CV curves with the nearly symmetric shapes suggests that the areas of CV loops can represent the specific capacitance of supercapacitor electrodes. The lowest current density of the MnO2/VG1:5 exhibit the smallest integrated loop area, indicating an inferior specific capacitance.5 On the other hand, the electrode of MnO2/VG1:2.5 with the VG growth in a medium level of density demonstrates the strongest current density in CV curves, suggesting the largest specific capacitance. The better electrochemical performance of MnO2/VG1:2.5 can be ascribed to the VG scaffold with the medium density, which provides plenty of surface area compared to VG1:5. On the other hand, MnO2/VG1:2.5 holds sufficient distances between nanowalls, leaving enough space for the growth of MnO2 instead of forming thicker MnO2 depositions, compared to MnO2/VG1:1. As shown in Fig. 1(a) and (a′), the smaller gaps between nanowalls (VG1:1) and thicker MnO2 depositions (MnO2/VG1:1), which may increase the inner resistance due to the poor electrical conductivity of MnO2 and hinder the access of electrolytes to active materials, affect the charge transport and redox reaction, resulting in the lower negative and positive current density. In contrast, the weakest CV current density is owing to the VG and MnO2-coated VG with a lowest level of density giving a smaller amount of active materials, as observed in Fig. 1(c) and (c′) for VG1:5 and MnO2/VG1:5. These results suggest that the medium levels of graphene densities are able to offer a balance between the accessibility of electrolytes to active materials and the adequacy of pseudocapacitive materials, producing the supercapacitor electrodes with promoted electrochemical performance.
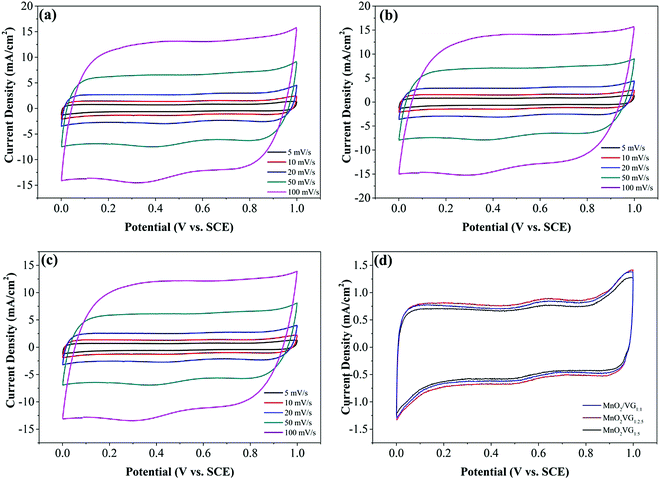 |
| Fig. 4 CV curves of (a) MnO2/VG1:1, (b) MnO2/VG1:2.5, and (c) MnO2/VG1:5 at various scan rates from 5 mV s−1 to 100 mV s−1; (d) comparison of the three electrodes at a scan rate of 5 mV s−1. | |
As shown in Fig. 5(a)–(c), further electrochemical evaluations with GV charge–discharge curves obtained at current densities sweeping from 0.5 mA cm−2 to 5 mA cm−2 for the three electrodes are conducted. The nearly symmetrical and quasi-triangular profiles for all the GV curves suggest superior reversibility and pseudocapacitive behaviors.57,58 Among the three electrodes, the longest discharging time of MnO2/VG1:2.5 (Fig. 5(b)) suggests the better electrochemical performance, which complies with the CV curves showing the largest negative-to-positive current density. The area-normalized specific capacitance based on GV measurements can be calculated by using the formula shown below.59,60
where
I and Δ
t are the discharging current density and duration.
A and Δ
V represent the operation area of an electrode and potential window. As shown in
Fig. 4(d), the area-normalized specific capacitance of the three electrodes of MnO
2/VG
1:1, MnO
2/VG
1:2.5, and MnO
2/VG
1:5 can be calculated to be 148 mF cm
−2, 166 mF cm
−2, and 142 mF cm
−2 at a current density of 0.5 mA cm
−2, respectively. The specific capacitance of MnO
2/VG
1:2.5 at higher current rates up to 5 mA cm
−2 retains the highest values compared to the other two electrodes, indicating the improved charge–discharge properties under all the working conditions. The reduction of the area-normalized specific capacitance of MnO
2/VG
1:2.5 from 166 mF cm
−2 to 142 mF cm
−2 occurs due to the increase of the charge–discharge rate from 0.5 mA cm
−2 to 5 mA cm
−2, which demonstrates an excellent capacitance retention of 86.04%. Besides, the other two electrodes of MnO
2/VG
1:1 and MnO
2/VG
1:5 exhibit similar retentions of 87.4% and 85.84% respectively, indicating the superior rate capability with the increasing charge–discharge rates.
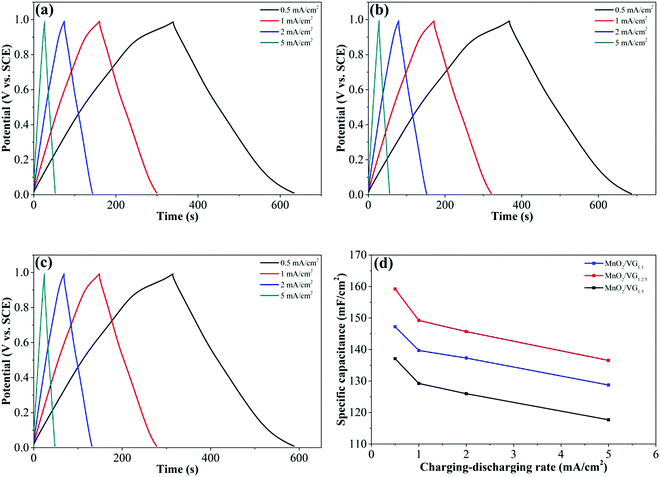 |
| Fig. 5 GV curves of (a) MnO2/VG1:1, (b) MnO2/VG1:2.5, (c) MnO2/VG1:5, and (d) area-normalized specific capacitance of three electrodes at different charge–discharge rates from 0.5 mA cm−2 to 5 mA cm−2. | |
The ratios of gaseous precursors, densities of VG nanowalls, and area-normalized specific capacitance are summarized in Table 1. It has to be mentioned that the growing time, chamber pressure, and operation power of plasma, etc., which are also the factors determining the growing densities of VG nanowalls, are set as constants for all the three electrodes. The quantitative values of VG layers agree with the observations in Fig. 1(a)–(c) that the increasing distances of gaps are observed from VG1:1 to VG1:5. With increasing ratios of H2, the VG densities on substrates are reduced due to the etching function of H-atoms, as discussed in the previous reports.37,38 The lowest densities of VG1:5 and MnO2/VG1:5 exhibit the poorest specific capacitance because of the less available nanowalls for growing active materials. Compared with MnO2/VG1:5, the increase of specific capacitance (∼17%), with the larger density of VG1:2.5 followed by the MnO2 deposition, is observed due to the increasing amount of nanowalls and active materials in MnO2/VG1:2.5. However, the inversion of specific capacitance turning to a decrease in MnO2/VG1:1 with the largest density of VG nanowalls occurs, when the optimal VG density with a medium level in MnO2/VG1:2.5 is reached. The higher density of MnO2/VG1:1 leads to the worse access of electrolytes to the active materials, resulting in the poor redox reaction and lower specific capacitance (∼10%). Consequently, MnO2/VG1:2.5 shows the highest area-normalized specific capacitance of 166 mF cm−2, compared to the other two electrodes.
Table 1 Summarization of ratios, densities and area-normalized specific capacitance at 0.5 mA cm−2
Ratio of CH4 : H4 |
VG density (mg cm−2) |
Specific capacitance (mF cm−2) |
1 : 1 |
3.63 |
148 |
1 : 2.5 |
2.35 |
166 |
1 : 5 |
0.83 |
142 |
Electrochemical impedance spectroscopy (EIS) measurements representing the electrochemical properties between electrodes and electrolytes in the charge–discharge procedure are conducted for the three electrodes. The Nyquist plots of MnO2/VG1:1, MnO2/VG1:2.5, and MnO2/VG1:5 are displayed in Fig. 6. The intersections between the curves and x-axis are ascribed to equivalent series resistance (Rs) including the resistance of electrodes, electrolytes, and the contact resistances of electrodes and substrates.61 All the three electrodes show the small Rs with ∼3.2 Ω (MnO2/VG1:1), ∼3.0 Ω (MnO2/VG1:2.5), and ∼4.0 Ω (MnO2/VG1:5), suggesting that the low resistance contributes to the direct growth and VG scaffolds on silicon wafer substrates and intimate contacts between VG and active materials as discussed in our previous work.33 The lowest Rs of MnO2/VG1:2.5 can be interpreted by the medium density of nanowalls and the appropriate thickness of active materials. In contrast, the higher thickness of MnO2 depositions and smaller distances between VG nanowalls result in a larger Rs, due to the weak electrical conductivity of MnO2 in MnO2/VG1:1. The lowest density of VG scaffolds (MnO2/VG1:5) gives the fewer nanowalls serving as electrical conductors, leading to the higher equivalent series resistance. Furthermore, the diameters of semicircle arcs named RCT correspond to the resistance of electrolytes diffusing into the pores of electrodes and the charge transfer resistance.62–65 The similar RCT values at around 0.5 Ω for the three electrodes indicate that the fast interactions between electrodes and electrolytes, contributed to the highly distributed active materials and porous structure of MnO2 depositions. In the low-frequency region, the nearly perpendicular lines for all the three electrodes suggest the ideal capacitive behavior (either EDLC or pseudocapacitive) and low Warburg resistance (W), indicating the excellent ion diffusivity and capacitive behaviors.19,57 The slightly inclined curve of MnO2/VG1:2.5, compared to that of the other two electrodes, reveals the more pseudocapacitive behaviors leading to the highest area-normalized specific capacitance, because of the increasing amount of active materials involved in the faradaic redox reaction.
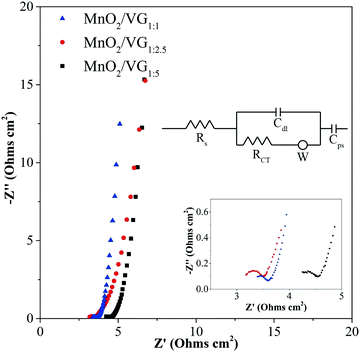 |
| Fig. 6 Nyquist plots of MnO2/VG1:1, MnO2/VG1:2.5, and MnO2/VG1:5. Insets: Equivalent circuit. | |
The cycling stability of the MnO2/VG1:2.5 electrode tested in a three-electrode system is evaluated at a constant charge–discharge rate of 5 mA cm−2 with the working window of 0–1 V for consecutive 5000 cycles to confirm the long-term charge–discharge performance. As shown in Fig. 7, the high retention (98.68%) of the original capacitance at the end of 5000 cycles suggests the superior durability ascribed the direct growth of VG on silicon substrates and strong bonding between active materials and scaffolds. The surface morphologies of MnO2/VG1:2.5 after 500, 2000, and 5000 cycles, showing the changes in microstructures under long-term cycles, are demonstrated in the insets. The redistribution of active materials from the initial status to 500 cycles can be observed, which results in the changes in the microstructures. However, the reversible charge–discharge process of MnO2 leads to the constant specific capacitance at the initial cycling stage. The ignorable morphology changes in the SEM images for 500, 2000, and 5000 cycles suggest the stable and robust adhesion of MnO2, achieve the long-term stability of the supercapacitor electrode.
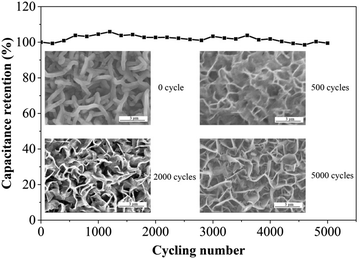 |
| Fig. 7 Durability test for MnO2/VG1:2.5 electrode at a constant charge–discharge rate of 5 mA cm−2 for consecutive 5000 cycles. Insets: Surface morphologies of MnO2/VG1:2.5 at the initial status and after 500, 2000, and 5000 cycles. | |
3.4. Assembly of asymmetric supercapacitors with MnO2/VG/Si and carbon black electrodes
The feasibility study of the supercapacitor electrodes containing the directly grown VG and electrochemically deposited MnO2 in a supercapacitor device is of importance investigating the practical potentials of the prepared supercapacitor electrodes. The asymmetric supercapacitor devices with MnO2/VG/Si as the positive electrode and carbon black as the negative electrode are assembled in a coin cell including 1 M Na2SO4 aqueous solution as the electrolyte and a piece of filter paper as the separator. The shortened deposition time of MnO2 (400 s) in the above-mentioned electrodes benefits the microstructural observations, due to the longer duration of electrochemical depositions resulting in the complete filling of the gaps.33 In contrast, the increasing amounts of active materials deposited on electrodes provide a higher specific capacitance, which can be used to assemble supercapacitor devices with a better energy storage capability. Meanwhile, the robust adhesions of VG on silicon wafer substrates can afford heavy amounts of MnO2 depositions to obtain supercapacitor electrodes with an excellent electrochemical performance.33 To demonstrate the stability of VG scaffolds and the good electrochemical properties, the positive electrode with VG1:2.5 as the scaffold and MnO2 electro-deposited under a constant potential of 0.6 V for 4000 s is prepared for the assembly of the asymmetric supercapacitor. The porous structures of MnO2 depositions with longer growing times, which may fill the gaps between nanowalls as observed in our previous study, are still able to provide free access of ions in the electrolyte to interact with electrodes.33 The deposition amount of MnO2 for 4000 s with the operation potential of 0.6 V is calculated as 3.93 mg. The mass balance of carbon black nanoparticles loaded in the negative electrode is about 34.1 mg. Due to the energy storage nature of EDLC in negative electrodes, the loading amounts of carbon black are greatly larger than those of MnO2 in positive electrodes, in order to give the corresponding energy storage capability. Fig. 8(a) shows the electrochemical performance of positive and negative electrodes conducting charge–discharge process in the Na2SO4 electrolyte with three electrode system before assembling in coin cells. The positive electrodes with the working potential ranging from ∼0 to ∼1.3 V and negative electrodes with the potential windows of ∼0 and ∼−0.7 V with all the current densities between 0.5 and 5 mA cm−2, have the expected potential ranges to store electrical power. The CV curves performed with the scan rates of 5, 10, 20, 50, and 100 mV s−1 under the voltage window of 0–1.8 V for the device are shown in Fig. 8(b). The absence of the polarization phenomenon at all the CV curves indicates that the device can reach the working voltage up to 1.8 V.66 The GV charge–discharge plots with the symmetrical and linear profiles for all the current densities varying from 0.5 to 5 mA cm−2 suggest the good electrochemical performance of the as-prepared supercapacitor device as shown in Fig. 8(c). The small IR drops for all the curves at the inverting points from the charge–discharge procedure imply the small Rs, which are ascribed to the optimum preparation process of the device.5 More importantly, the direct growth of VG on silicon wafers forms the tight and intimate connections between active materials and current collectors, which greatly reduce the Rs. Furthermore, the specific capacitance of the supercapacitor device can be calculated with the equation as mentioned above. The area-normalized specific capacitances with the values of 230.93 mF cm−2 (0.5 mA cm−2) and 164.92 mF cm−2 (5 mA cm−2) are obtained, presenting the excellent capacitance retention of 71.42% with the increasing current density from 0.5 mA cm−2 to 5 mA cm−2. Furthermore, the energy and power density are calculated by following the formulas67
where E, P, and CA are energy density, power density, and specific capacitance, respectively. ΔV and Δt represent potential window and discharge time. The maximum energy density and power density are calculated as E = 103.92 μW h cm−2 (P = 0.45 mW cm−2 and 0.5 mA cm−2) and P = 4.5 mW cm−2 (E = 74.22 μW h cm−2 and 5 mA cm−2). The asymmetric supercapacitor device exhibits a superior electrochemical performance, compared to the reported studies as listed in Table 2.
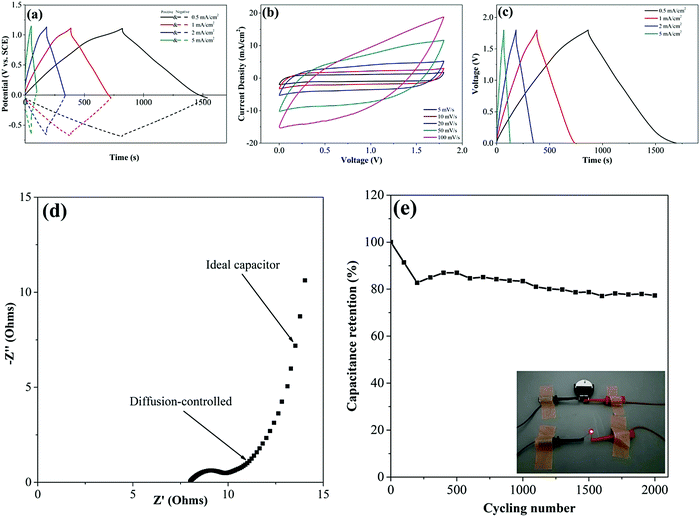 |
| Fig. 8 (a) GV curves of positive and negative electrodes in the Na2SO4 electrolyte before assembling in coin cells; (b) CV curves; (c) GV curves; (d) Nyquist plot; and (e) cycling stability with inset of powering a red LED of the asymmetric supercapacitor device. | |
Table 2 Comparing asymmetric supercapacitor device with the previous reported literature
Material |
Energy density (μW h cm−2) |
Power density (mW cm−2) |
Specific capacitance (mF cm−2) |
Capacitance retention (%) [cycles] |
Ref. |
MnO2/VGNs/Ni |
7.7 |
5 |
56 |
80 [2000] |
5
|
GNW/RuOx |
15.1 |
2.49 |
109 |
N/A |
31
|
CF/MnO2 |
2.7 |
8.3 |
4.9 |
89 [3000] |
69
|
MnO2/VG/Si |
103.9 |
4.5 |
230.9 |
77.3 [2000] |
This work |
The properties of the supercapacitor device measured using electrochemical impedance spectroscopy (EIS) using the Nyquist plot are evaluated as shown in Fig. 8(d). The low Rs of ∼8 Ω indicates the good equivalent series resistance, which is attributed to the intimate contacts between the VG scaffold and active materials. The RCT (∼2 Ω) illustrating that the ion transfer resistance suggests the superior interactions between electrodes and electrolytes. Furthermore, the slope angle with 45 °C at the middle-frequency region, which shows the diffusion-controlled process, is ascribed by the longer deposition time producing thicker MnO2 layer and the porous structures of MnO2.33,68 The nearly vertical line at the low-frequency area, representing the ideal capacitive behavior, shows excellent pseudocapacitive performance. The durability test of the supercapacitor device is conducted to show the relatively high capacitance retention of 77.32% over 2000 cycles. The asymmetric supercapacitor is able to power a red LED as demonstrated in the inset of Fig. 8(e).
4. Conclusions
In summary, tunable densities of vertical graphene nanowalls deposited on silicon wafer substrates via PECVD have been achieved, which breaks the limits that the traditional fabrication methods have difficulties controlling the gap distances between graphene nanowalls. The improvement of MnO2 electrochemical deposition, distributed on the surface of nanowalls uniformly by using a mixture of ethanol and distilled water as the solvent, provides access to reveal the density effects of scaffolds on the electrochemical performance of supercapacitor electrodes. The vertical graphene scaffolds with different densities are controlled by varying the flow ratios of precursor gaseous materials to be 1
:
1, 1
:
2.5, and 1
:
5 for CH4
:
H2, followed by the electrochemical deposition of MnO2 under a constant condition. We have found that the electrode of MnO2/VG1:2.5 with the medium density of VG scaffold shows the maximum area-normalized specific capacitance of 166 mF cm−2 among the three electrodes. The electrode of MnO2/VG1:2.5 exhibits excellent rate capacitance with the retention of 86% when the charge–discharge rate increases from 0.5 mA cm−2 to 5 mA cm−2. To investigate the applicable potentials, the asymmetric supercapacitor device with MnO2/VG/Si as the positive electrode and carbon black as the negative electrode has been assembled. The supercapacitor device demonstrates an excellent area-normalized specific capacitance of 230.9 mF cm−2 with a maximum energy density of 103.9 μW h cm−2 and power density of 4.5 mW cm−2. The relatively high cycling stability with the capacitance retention of 77.3% under consecutive 2000 cycles is achieved. This approach represents the fundamental understanding of the density effects of scaffolds on the pseudocapacitive supercapacitor electrodes. Meanwhile, the superior electrochemical performance of the asymmetric supercapacitor device demonstrates the promising potentials to fabricate energy-storage electronics by using the electrodes based on vertical graphene and pseudocapacitive materials.
Conflicts of interest
There are no conflicts to declare.
Acknowledgements
This work was mainly performed in the Micro/Nanomachining Research Education Center (MNC) of Tohoku University and Micro System Integration Center, Tohoku University. This work is supported by Cross-ministerial Strategic Innovation Promotion Program (SIP), Japan (The New Energy and Industrial Technology Development Organization, NEDO).
References
- P. B. Koeneman, I. J. Busch-Vishniac and K. L. Wood, Feasibility of Micro Power Supplies for MEMS, J. Microelectromech. Syst., 1997, 6(4), 355–362 CrossRef.
- X. Wang and G. Shi, Flexible graphene devices related to energy conversion and storage, Energy Environ. Sci., 2015, 8, 790–823 RSC.
- H. E. Brouji, O. Briat, J.-M. Vinassa, H. Henry and E. Woirgard, Analysis of the dynamic behavior changes of supercapacitors during calendar life test under several voltages and temperatures conditions, Microelectron. Reliab., 2009, 49(9–11), 1391–1397 CrossRef.
- Y. Liu, X. Cai, B. Luo, M. Yan, J. Jiang and W. Shi, MnO2 decorated on carbon sphere intercalated graphene film for high-performance supercapacitor electrodes, Carbon, 2016, 107, 426–432 CrossRef CAS.
- Y. Zhou, X. Cheng, F. Huang, Z. Sha, Z. Han, J. Chen, W. Yang, Y. Yu, J. Zhang, S. Peng, S. Wu, A. Rider, L. Dai and C. H. Wang, Hierarchically structured electrodes for moldable supercapacitors by synergistically hybridizing vertical graphene nanosheets and MnO2, Carbon, 2021, 172, 272–282 CrossRef CAS.
- Z. Wu, K. Parvez, S. Li, S. Yang, Z. Liu, S. Liu, X. Feng and K. Mullen, Alternating Stacked Graphene-Conducting Polymer Compact Films with Ultrahigh Areal and Volumetric Capacitances for High-Energy Micro-Supercapacitors, Adv. Mater., 2015, 27, 4054–4061 CrossRef CAS PubMed.
- Y. Jiang and J. Liu, Definitions of Pseudocapacitive Materials: A Brief Review, Energy Environ., 2019, 2(1), 30–37 Search PubMed.
- Z. Fahimi and O. Moradlou, Fabrication of ZnO@C foam: a flexible free-standing electrode for energy storage devices, Mater. Des., 2020, 189, 108525 CrossRef CAS.
- M. Kim, Y. Hwang and J. Kim, Process dependent graphene/MnO2 composites for supercapacitors, Chem. Eng. J., 2013, 230, 482–490 CrossRef CAS.
- W. Guo, C. Yu, S. Li, Z. Wang, J. Yu, H. Huang and J. Qiu, Strategies and insights towards the intrinsic capacitive properties of MnO2 for supercapacitors: Challenges and perspectives, Nano Energy, 2019, 57, 459–472 CrossRef CAS.
- D. Yan, Z. Guo, G. Zhu, H. Yang, R. Wei, H. Xu and A. Yu, Electrochemical properties of 3D MnO2 film prepared by chemical bath deposition at room temperature, Mater. Lett., 2012, 82, 156–158 CrossRef CAS.
- G. Yu, L. Hu, N. Liu, H. Wang, M. Vosgueritchian, Y. Yang, Y. Cui and Z. Bao, Enhancing the supercapacitor performance of graphene/MnO2 nanostructured electrodes by conductive wrapping, Nano Lett., 2011, 11, 4438–4442 CrossRef CAS PubMed.
- X. Zhou, T. Meng, F. Yi, D. Shu, Z. Li, Q. Zeng, A. Gao and Z. Zhu, Supramolecular assisted fabrication of Mn3O4 anchored nitrogen-doped reduced graphene oxide and its distinctive electrochemical activation process during supercapacitive study, Electrochim. Acta, 2021, 370, 137739 CrossRef CAS.
- N. Jabeen, Q. Xia, S. V. Savilov, S. M. Aldoshin, Y. Yu and H. Xia, Enhanced Pseudocapacitive Performance of a-MnO2 by Cation Preinsertion, ACS Appl. Mater. Interfaces, 2016, 8(49), 33732–33740 CrossRef CAS PubMed.
- X. Zhu, Q. Xia, X. Liu, Q. Zhang, J. Xu, B. Lin, S. Li, Y. Zhuang, C. Qiu, L. Xue, L. Gu and H. Xia, Retarded layered-to-spinel phase transition in structure reinforced
birnessite with high Li content, Sci. Bull., 2021, 66(3), 219–224 CrossRef CAS.
- N. J.-A. Hussain, Q. Xia, S. Sun, J. Zhu and H. Xia, High-Performance 2.6 V Aqueous Asymmetric Supercapacitors based on In Situ Formed Na0.5MnO2 Nanosheet Assembled Nanowall Arrays, Adv. Mater., 2017, 29(32), 1700804 CrossRef PubMed.
- W. Wei, X. Cui, W. Chen and D. G. Ivey, Manganese oxide-based materials as electrochemical supercapacitor electrodes, Chem. Soc. Rev., 2011, 40, 1697–1721 RSC.
- Z. Li, Y. Mi, X. Liu, S. Liu, S. Yang and J. Wang, Flexible graphene/MnO2 composite papers for supercapacitor electrodes, J. Mater. Chem., 2011, 21, 14706–14711 RSC.
- Z. Ye, T. Li, G. Ma, X. Peng and J. Zhao, Morphology controlled MnO2 electrodeposited on carbon fiber paper for high-performance supercapacitors, J. Power Sources, 2017, 351, 51–57 CrossRef CAS.
- Z. Zhao, T. Shen, Z. Liu, Q. Zhong and Y. Qin, Facile fabrication of binder-free reduced graphene oxide/MnO2/Ni foam hybrid electrode for high-performance supercapacitors, J. Alloys Compd., 2020, 812, 15124 CrossRef.
- Q. Zhang, X. Wu, Q. Zhang, F. Yang, H. Dong, J. Sui and L. Dong, One-step hydrothermal synthesis of MnO2/graphene composite for electrochemical energy storage, J. Electroanal. Chem., 2019, 837, 108–115 CrossRef CAS.
- S. Chen, J. Zhu, X. Wu, Q. Han and X. Wang, Graphene Oxide-MnO2 Nanocomposites for Supercapacitors, ACS Nano, 2010, 4(5), 2822–2830 CrossRef CAS PubMed.
- O. Sadak, W. Wang, J. Guan, A. K. Sundramoorthy and S. Gunasekaran, MnO2 Nanoflowers Deposited on Graphene Paper as Electrode Materials for Supercapacitors, ACS Appl. Nano Mater., 2019, 2, 4386–4394 CrossRef CAS.
- A. Oz, D. Gelman, E. Goren, N. Shomrat, S. Baltianski and Y. Tsur, A novel approach for supercapacitors degradation characterization, J. Power Sources, 2017, 355, 74–82 CrossRef CAS.
- Q. Zhang, J. Sun, Z. Pan, J. Zhang, J. Zhao, X. Wang, C. Zhang, Y. Yao, W. Lu, Q. Li, Y. Zhang and Z. Zhang, Stretchable fiber-shaped asymmetric supercapacitors with ultrahigh energy density, Nano Energy, 2017, 39, 219–228 CrossRef CAS.
- T. Liu and Y. Li, Addressing the Achilles' heel of pseudocapacitive materials: Long-term stability, InfoMat, 2020, 2(5), 807–842 CrossRef CAS.
- H. Shen, H. Li, M. Li, C. Li, L. Qian, L. Su and B. Yang, High-performance aqueous symmetric supercapacitor based on polyaniline/vertical graphene/Ti multilayer electrodes, Electrochim. Acta, 2018, 283, 410–418 CrossRef CAS.
- Z. J. Han, S. Pineda, A. T. Murdock, D. H. Seo, K. Ostrikov and A. Bendavid, RuO2-coated vertical graphene hybrid electrodes for high-performance solid-state supercapacitors, J. Mater. Chem. A, 2017, 5(33), 17293–17301 RSC.
- Z. Bo, Z. Wen, H. Kim, G. Lu, K. Yu and J. Chen, One-step fabrication and capacitive behavior of electrochemical double layer capacitor electrodes using vertically-oriented graphene directly grown on metal, Carbon, 2012, 50(12), 4379–4387 CrossRef CAS.
- J. Li, N. V. Toan, Z. Wang and T. Ono, Metal-assisted-chemical-etching of silicon nanowires for templating 3D graphene growth towards energy storage in microsystems, J. Micromech. Microeng., 2019, 29, 055007 CrossRef CAS.
- J. Li, M. Zhu, Z. An, Z. Wang, M. Toda and T. Ono, Constructing in-chip micro-supercapacitors of 3D graphene nanowall/ruthenium oxides electrode through silicon-based microfabrication technique, J. Power Sources, 2018, 401, 204–212 CrossRef CAS.
- J. Li, M. Zhu, Z. Wang and T. Ono, Engineering micro-supercapacitors of graphene nanowalls/Ni heterostructure based on microfabrication technology, Appl. Phys. Lett., 2016, 109, 153901 CrossRef.
- H. Sui, N. V. Toan and T. Ono, Vertically-oriented graphene electrodeposited with MnO2 on native SiO2/Si for high-performance supercapacitor electrodes, J. Electroanal. Chem., 2021, 895, 115507 CrossRef CAS.
- Y. Zhang, Q. Zou, H. S. Hsu, S. Raina, Y. Xu, J. B. Kang, J. Chen, S. Deng, N. Xu and W. P. Kang, Morphology Effect of Vertical Graphene on the High Performance of Supercapacitor Electrode, ACS Appl. Mater. Interfaces, 2016, 8, 7363–7369 CrossRef CAS PubMed.
- D. Majumdar, T. Maiyalagan and Z. Jiang, Recent Progress in Ruthenium Oxide-Based Composites for Supercapacitor Applications, ChemElectroChem, 2019, 6(17), 4343–4372 CrossRef CAS.
- X. Hu, L. Wei, R. Chen, Q. Wu and J. Li, Reviews and Prospectives of Co3O4-Based Nanomaterials for Supercapacitor Application, ChemistrySelect, 2020, 5(17), 5268–5288 CrossRef CAS.
- J. Wang, M. Zhu, R. A. Outlaw, X. Zhao, D. M. Manos and B. C. Holloway, Synthesis of carbon nanosheets by inductively coupled radio-frequency plasma enhanced chemical vapor deposition, Carbon, 2004, 42(14), 2867–2872 CrossRef CAS.
- Y. Wu, B. Yang, B. Zong, H. Sum, Z. Shen and Y. Feng, Carbon nanowalls and related materials, J. Mater. Chem., 2004, 14, 469–477 RSC.
- Z. Bo, K. Yu, G. Lu, P. Wang, S. Mao and J. Chen, Understanding growth of carbon nanowalls at atmospheric pressure using normal glow discharge plasma-enhanced chemical vapor deposition, Carbon, 2011, 49(6), 1849–1858 CrossRef CAS.
- V. Krivchenko, P. Shevnin, A. Pilevsky, A. Egorov, N. Suetin, V. Sen, S. Evlashin and A. Rakhimov, Influence of the growth temperature on structural and electron field emission properties of carbon nanowall/nanotube films synthesized by catalyst-free PECVD, J. Mater. Chem., 2012, 22, 16458–16464 RSC.
- Z. Bo, Y. Yang, J. Chen, K. Yu, J. Yan and K. Cen, Plasma-enhanced chemical vapor deposition synthesis of vertically oriented graphene nanosheets, Nanoscale, 2013, 5, 5180–5204 RSC.
- Y. Zhang, J. Du, S. Tang, P. Liu, S. Deng, J. Chen and N. Xu, Optimize the field emission character of a vertical few-layer graphene sheet by manipulating the morphology, Nanotechnology, 2012, 23, 015202 CrossRef PubMed.
- L. Wang, B. Wei, P. Dong, Q. Miao, Z. Liu, F. Xu, J. Wu, J. Lou, R. Vajtai and W. Fei, Large-scale synthesis of few-layer graphene from magnesium and different carbon sources and its application in dye-sensitized solar cells, Mater. Des., 2016, 92, 462–470 CrossRef CAS.
- Q. Zhang, S. Li, S. Sun, X. Yin and J. Yu, Lithium selective adsorption on 1-D MnO2 nanostructure ion-sieve, Adv. Powder Technol., 2009, 20(5), 432–437 CrossRef CAS.
- B. R. Chen, W. Sun, D. A. Kitchaev, J. S. Mangum, V. Thampy, L. M. Garten, D. S. Ginley, B. P. Gorman, K. H. Stone, G. Ceder, M. F. Toney and L. T. Schelhas, Understanding crystallization pathways leading to manganese oxide polymorph formation, Nat. Commun., 2018, 9, 2553 CrossRef PubMed.
- Z. Niu, T. Yue, W. Hu, W. Sun, Y. Hu and Z. Xu, Covalent bonding of MnO2 onto graphene aerogel forwards: Efficiently catalytic degradation of organic wastewater, Appl. Surf. Sci., 2019, 496, 143585 CrossRef CAS.
- S. Qiu, R. Li, Z. Huang, Z. Huang, C. P. Tsui, C. He, X. Han and Y. Yang, Scalable sonochemical synthesis of petal-like MnO2/graphene hierarchical composites for high-performance supercapacitors, Composites, Part B, 2019, 161, 37–43 CrossRef CAS.
- C. Yang, H. Bi, D. Wan, F. Huang, X. Xie and M. Jiang, Direct PECVD growth of vertically erected graphene walls on dielectric substrates as excellent multifunctional electrodes, J. Mater. Chem. A, 2013, 1, 770–775 RSC.
- S. Y. Huang, P. A. Le, P. J. Yen, Y. C. Lu, S. K. Sahoo, H. W. Cheng, P. W. Chiu, T. Y. Tseng and K. H. Wei, Cathodic plasma–induced syntheses of graphene nanosheet/MnO2/WO3 architectures and their use in supercapacitors, Electrochim. Acta, 2020, 342, 136043 CrossRef CAS.
- L. Lu, H. Tian, J. He and Q. Yang, Graphene-MnO2 Hybrid Nanostructure as a New Catalyst for Formaldehyde Oxidation, J. Phys. Chem. C, 2016, 120, 23660–23668 CrossRef CAS.
- S. Ghosh, B. Gupta, K. Ganesan, A. Das, M. Kamruddin, S. Dash and A. K. Tyagi, MnO2-Vertical graphene nanosheets composite electrodes for energy storage devices, Mater. Today: Proc., 2016, 3(6), 1686–1692 Search PubMed.
- A. Zandiatashbar, G. H. Lee, S. J. An, S. Lee, N. Mathew, M. Terrones, T. Hayashi, C. R. Picu, J. Hone and N. Koratkar, Effect of defects on the intrinsic strength and stiffness of graphene, Nat. Commun., 2014, 5, 3186 CrossRef PubMed.
- S. Jadhav, R. S. Kalubarme, C. Terashima, B. B. Kale, V. Godbole, A. Fujishima and S. W. Gosavi, Manganese dioxide/reduced graphene oxide composite an electrode material for high-performance solid state supercapacitor, Electrochim. Acta, 2019, 299, 34–44 CrossRef CAS.
- C. Julien, M. Massot, R. Baddour-Hadjean, S. Franger, S. Bach and J. P. Pereira-Ramos, Raman spectra of birnessite manganese dioxides, Solid State Ion., 2003, 159(3–4), 345–356 CrossRef CAS.
- B. Ouyang, Y. Zhang, Z. Zhang, H. J. Fan and R. S. Rawat, Green synthesis of vertical graphene nanosheets and their application in high-performance supercapacitors, RSC Adv., 2016, 6, 23968–23973 RSC.
- A. Noori, M. F. El-Kady, M. S. Rahmanifar, R. B. Kaner and M. F. Mousavi, Towards establishing standard performance metrics for batteries, supercapacitors and beyond, Chem. Soc. Rev., 2019, 48(5), 1272–1341 RSC.
- X. Su, L. Yu, G. Cheng, H. Zhang, M. Sun and X. Zhang, High-performance α-MnO2 nanowire electrode for supercapacitors, Appl. Energy, 2015, 153, 94–100 CrossRef CAS.
- Q. Cheng, J. Tang, J. Ma, H. Zhang, N. Shinya and L. Qin, Graphene and nanostructured MnO2 composite electrodes for supercapacitors, Carbon, 2011, 49(9), 2917–2925 CrossRef CAS.
- S. Zhao, T. Liu, D. Hou, W. Zeng, B. Miao, S. Hussain, X. Peng and M. S. Javed, Controlled synthesis of hierarchical birnessite-type MnO2 nanoflowers for supercapacitor applications, Appl. Surf. Sci., 2015, 356, 259–265 CrossRef CAS.
- X. Wang, Y. Yin, C. Hao and Z. You, A high-performance three-dimensional micro supercapacitor based on ripple-like ruthenium oxide–carbon nanotube composite films, Carbon, 2015, 82, 436–445 CrossRef CAS.
- B. A. Mei, O. Munteshari, J. Lau, B. Dunn and L. Pilon, Physical Interpretations of Nyquist Plots for EDLC Electrodes and Devices, J. Phys. Chem. C, 2018, 122, 194–206 CrossRef CAS.
- I. Yang, S. G. Kim, S. H. Kwon, M. S. Kim and J. C. Jung, Relationships between pore size and charge transfer resistance of carbon aerogels for organic electric double-layer capacitor electrodes, Electrochim. Acta, 2017, 223, 21–30 CrossRef CAS.
- B. Fang and L. Binder, A modified activated carbon aerogel for high-energy storage in electric double layer capacitors, J. Power Sources, 2006, 163(1), 616–622 CrossRef CAS.
- C. Liu, W. Dong, G. Cao, J. Song, L. Liu and Y. Yang, Influence of KOH followed by oxidation pretreatment on the electrochemical performance of phenolic based activated carbon fibers, J. Electroanal. Chem., 2007, 611(1–2), 225–231 CrossRef CAS.
- C. Lei, F. Markoulidis, Z. Ashitaka and C. Lekakou, Reduction of porous carbon/Al contact resistance for an electric double-layer capacitor (EDLC), Electrochim. Acta, 2013, 92, 183–187 CrossRef CAS.
- M. He, K. Fic, E. Fr
ckowiak, P. Novák and E. J. Berg, Ageing phenomena in high-voltage aqueous supercapacitors investigated by in situ gas analysis, Energy Environ. Sci., 2016, 9, 623–633 RSC.
- M. Biswal, A. Banerjee, M. Deo and S. Ogale, From dead leaves to high energy density supercapacitors, Energy Environ. Sci., 2013, 6, 1249–1259 RSC.
- Q. Li, Y. Q. Zhu and S. J. Eichhorn, Structural supercapacitors using a solid resin electrolyte with carbonized electrospun cellulose/carbon nanotube electrodes, J. Mater. Sci., 2018, 53, 14598–14607 CrossRef CAS.
- J. Noh, C. M. Yoon, Y. K. Kim and J. Jang, High performance asymmetric supercapacitor twisted from carbon fiber/MnO2 and carbon fiber/MoO3, Carbon, 2017, 116, 470–478 CrossRef CAS.
|
This journal is © The Royal Society of Chemistry 2022 |