DOI:
10.1039/D2MA00058J
(Paper)
Mater. Adv., 2022,
3, 3593-3599
MoS2 nanosheets for the detoxification of Hg2+ in living cells†
Received
19th January 2022
, Accepted 6th March 2022
First published on 8th March 2022
Abstract
A simple and green one-step method for the simultaneous exfoliation and functionalization of few-layered MoS2 nanosheets was developed via the ultrasonic treatment of bulk MoS2 powder in sodium phytate-containing aqueous solution. The as-prepared MoS2 nanosheets show high stability, low cytotoxicity and high adsorption capacity for Hg2+ in aqueous solution. The maximum adsorption capacity for Hg2+ is about 7.5 times and 3.7 times that of bulk MoS2 powder and unfunctionalized MoS2 nanosheets, respectively. The adsorption kinetics and thermodynamics indicate that the adsorption of Hg2+ is a monolayer chemical adsorption. Finally, the as-prepared MoS2 nanosheets were applied for the removal of Hg2+ in HepG2 cells and show a similar detoxification effect as that of the common antidote meso-2,3-dimercaptosuccinic acid, indicating the potential application of MoS2 nanosheets for biological detoxification.
1. Introduction
The mercury ion (Hg2+) is a highly toxic ion that can cause gastrointestinal mucosal and kidney failure, central nervous system damage and even death.1,2 Hg2+ contamination has become increasingly serious in our living environment and ecosystem. The development of efficient technologies for the removal of Hg2+ has received tremendous attention. Traditional Hg2+ removal technologies include chemical precipitation,3 electrochemical processes,4 adsorption,5 membrane separation,6 ion exchange7 and solvent extraction.8 Among these technologies, adsorption has the advantages of simple operation and low cost. Owing to the large number of sulfur (S) atoms exposed on the surface and the strong soft-soft interaction between Hg2+ and S, molybdenum disulfide (MoS2), a typical layered transition-metal dichalcogenide (TMDC)9 composed of a hexagonal plane with Mo atoms sandwiched by two sulfur atoms (S–Mo–S), can be used as a promising adsorbent for the removal of Hg2+.10 Therefore, MoS2 nanosheets with widened interlayer spacing,10 two-dimensional (2D) MoS2 nanosheets,11 oxygen-incorporated MoS2 nanosheets,12 Au/Fe3O4/MoS2 aerogels,13 d-MoS2/Fe3O4 nanohybrids,14 and cellulose/MoS2/Fe3O4 composites15 have been shown to be excellent adsorbents for the removal of Hg2+ from aqueous solutions. Although these MoS2-based adsorbents show high adsorption capacity for Hg2+, they are still in the proof-of-concept phase and none of the MoS2-based adsorbents have been applied to remove Hg2+ in real complex environmental and biological samples.
Bulk MoS2 has strong chemical bonding within its layers and weak van der Waals forces between its layers. To expose more surfaces with S atoms to the outside for adsorption, the exfoliation of bulk MoS2 into single- or few-layered nanosheets is necessary. To date, chemical exfoliation with alkali metal compounds and liquid-phase exfoliation are recognized as effective methods for the scale-up production of MoS2 nanosheets.16 However, chemical exfoliation requires a harsh and strong intercalating reagent (such as n-butyl lithium) followed by ultrasonic exfoliation under inert atmosphere.17,18 Moreover, the preparation procedure is expensive, dangerous, long and tedious. Liquid-phase exfoliation of bulk MoS2via facile ultrasonic treatment in organic solvents, such as ethanol,19,20 2-propanol,21N-methyl-2-pyrrolidone,22 acetone and alcohols (ethanol, methanol and isopropanol),23 or in aqueous solution with auxiliary reagents such as thiolated surfactants,24 polystyrene-polyacrylamide,25 bovine serum albumin,26 poly(3,4-ethylenedioxythiophene):poly-(styrenesulfonate),27 Nafion,28 and polymeric ionic liquids,29 has been successfully developed and is widely applied in the fields of catalysis, organic solar cells, antibacterial materials, conductive films, etc. However, these organic solvents, surfactants, and polymers are mostly toxic, and will thus cause adverse effects in biological applications. Therefore, a facile method for the exfoliation and functionalization of MoS2 nanosheets with a green and cost-effective auxiliary reagent to reinforce their biocompatibility and functionality for biological application is highly desired.30,31
Herein, we report a facile green method to simultaneously exfoliate and functionalize MoS2 nanosheets via ultrasonic treatment of bulk MoS2 in sodium phytate-containing aqueous solution (Scheme 1). Sodium phytate is the sodium salt of phytic acid (myo-inositol-1,2,3,4,5,6-hexakisphosphate) extracted from rice bran and is a natural chelating agent. The as-prepared MoS2 nanosheets show high stability in phosphate buffer solution in the pH range of 2.0–12.0 and low cytotoxicity. Taking advantage of the high binding affinity between Hg2+ and S sites on the surface of MoS2 sheets10,11 and the biocompatible, biodegradable, and nontoxic phytate,32 the as-prepared MoS2 nanosheets were used as an adsorbent for the removal of Hg2+ from water and as a detoxifier for the removal of Hg2+ in HepG2 cells. Our results indicate that the as-prepared MoS2 nanosheets display a detoxification effect that is comparable to that of meso-2,3-dimercaptosuccinic acid (DMSA), a heavy metal detoxifier approved by the Food and Drug Administration of USA for the treatment of lead and mercury toxicity both in children and adults. To the best of our knowledge, this work is the first example to use TMDC for heavy metal detoxification in living cells.
 |
| Scheme 1 Schematic illustration of the exfoliation of bulk MoS2 and the functionalization of MoS2 nanosheets by phytate. | |
2. Experimental
2.1. Chemical and materials
Bulk MoS2 powder and DMSA were purchased from Shanghai Aladdin Biochemical Technology. Sodium phytate was bought from Sangon Biotech (Shanghai) Co., Ltd. Hg2+ standard solution (1000 μg mL−1) in 1.0 mol L−1 HNO3 was obtained from the National Nonferrous Metals and Electronic Materials Analysis and Testing Center of China, and was diluted to the desired concentration with water. Dulbecco's modified Eagle's medium (DMEM), phosphate buffered solution (PBS, 10 mmol L−1, pH 7.4) and Cell Counting Kit-8 (CCK-8) were purchased from GE Healthcare Life Sciences HyClone laboratories, Inc. Without specification, the metal ions were nitrate salts and anions were sodium salts. All water used was ultrapure water (18.2 MΩ cm, Millipore).
2.2. Exfoliation and functionalization of MoS2 nanosheets by phytate
200 mg Bulk MoS2 powder was added to 40 mL aqueous solution containing 40 mg sodium phytate. The suspension was ultrasonically treated for 35 h. The dispersion was centrifuged at 5000 rpm for 20 min to remove large particles, and the supernatant was further centrifuged at 12
000 rpm for 10 min to remove excessive sodium phytate. The precipitate was collected and redispersed in water. The removal of sodium phytate in the supernatant was repeated three times, and the collected precipitate was redispersed in water for further use. In addition, bulk MoS2 powder was separately exfoliated in Na2HPO4 solution and water under the same conditions.
2.3. Adsorption of Hg2+ by MoS2 nanosheets.
MoS2 nanosheets exfoliated by sodium phytate (6.4 mg) were added to 30 mL Hg2+ solution with different concentrations (100 ng mL−1, 1 μg mL−1, 10 μg mL−1 and 20 μg mL−1). The mixture solution was shaken at a rate of 300 rpm at room temperature (25 °C). An aliquot of 2 mL mixture solution was taken out at a certain time interval. After centrifugation at 12
000 rpm for 20 min, the supernatant was collected and filtered by a 0.22 μm filter to remove large particles. The concentration of residual Hg2+ in the filtrate was determined by ICP-MS.
2.4. Cytotoxicity assay and detoxification experiment
For the cytotoxicity assay, human hepatoma cells (HepG2) were treated with 0.25% trypsin for 1 min. After the trypsin solution was discarded, HepG2 cells were dispersed in DMEM medium by blowing. Then, HepG2 cells (1 × 105 cell in each well) were plated in a 96-microwell microplate and cultured in DMEM at 37 °C in a humidified 5% CO2 incubator for 24 h. After the cells were washed with PBS (10 mmol L−1, pH 7.4) three times to remove the secretions and culture medium, 100 μL MoS2 nanosheets exfoliated by sodium phytate (0, 50, 100, 250, 500 and 1000 μg mL−1), Hg2+ (0, 0.5, 1, 2, 5 and 10 μg mL−1), or DMSA (0, 50, 100, 150 and 200 μg mL−1) in DMEM were each added and cultured at 37 °C in a humidified 5% CO2 incubator for 12 h. After being washed with PBS (10 mmol L−1, pH 7.4) three times, 10 μL CCK-8 and 90 μL DMEM were added to each well and the cells were incubated in the dark for 2 h. Finally, the absorbance of each well at 450 nm was recorded. Each experiment was repeated five times. The relative survival rate of the cells was calculated by taking the cell survival rate of the blank as 100%.
To investigate the detoxification effect of the MoS2 nanosheets exfoliated by sodium phytate, 100 μL Hg2+ (2 μg mL−1) in DMEM was added to HepG2 cells (1 × 105 cell) and incubated in the dark for 2 h. After the cells were washed with PBS (10 mmol L−1, pH 7.4) three times, 11 μL MoS2 nanosheets or DMSA in DMEM (0, 50, 100, 150 and 200 μg mL−1) was further added. After further incubation for 12 h, the cells were washed with PBS (10 mmol L−1, pH 7.4) three times. The CCK-8 assay was used to detect the relative survival rate of the cells. Each experiment was repeated five times.
3. Results and discussion
3.1. Optimization of the exfoliation conditions
Bulk MoS2 powder was ultrasonically exfoliated in sodium phytate aqueous solution. The exfoliation conditions (such as ultrasonication time, concentrations of sodium phytate and bulk MoS2 powder) were optimized. The absorbance of the MoS2 nanosheets gradually increased with increasing time, and reached the platform until the ultrasonic time was up to 35 h (Fig. S1A, ESI†). The exfoliation yield, namely the mass ratio of the collected MoS2 nanosheets to bulk MoS2 powder, was increased with the concentration of sodium phytate until its concentration reached 1 mg mL−1 (Fig. S1B, ESI†). However, when the concentration of sodium phytate exceeded 1 mg mL−1, the exfoliation yield was decreased. Similarly, the exfoliation yield was increased with the concentration of bulk MoS2 powder until its concentration reached 8 mg mL−1 (Fig. S1C, ESI†). The exfoliation yield was reduced instead with the further increase of bulk MoS2 powder. Therefore, the optimal conditions for the exfoliation of MoS2 nanosheets are 35 h ultrasonic time, 1 mg mL−1 sodium phytate and 8 mg mL−1 bulk MoS2 powder. Under the optimal conditions, the exfoliation yield is 18.1%, which is 8 times that of the MoS2 nanosheets exfoliated in pure water. As shown in Table S1 (ESI†), the ultrasonic exfoliation yield is also higher than those by ultrasonic exfoliation in most solvents. The effect of pH on the stability of MoS2 nanosheets was also investigated (Fig. S1D, ESI†). MoS2 nanosheets can be dispersed stably in phosphate buffer (10 mmol L−1) over a pH range of 2.0–12.0, indicating that the functionalization of phytate on the surface of the MoS2 nanosheets endows their high stability in aqueous solution.
3.2. Characterization of MoS2 nanosheets
There are two distinctive absorption peaks at 665 nm and 603 nm (A and B excitons, respectively) in the absorption spectrum of the MoS2 nanosheets solution (Fig. 1A), which are typical characteristics of the hexagonal symmetry space group of 2H-MoS2, corresponding to the direct excitonic transition of MoS2 at the K-points of the Brillouin zone.23,28 According to the relationship between the mean number of layers and the wavelength of the A-exciton (λA) proposed by Coleman and co-workers,31 the mean number of layers is estimated to be less than 3. The potential of MoS2 nanosheets was measured to be −33.4 mV (Fig. 1B), indicating that the functionalization by phytate endows the MoS2 nanosheets with abundant negative charges and high hydrophilicity and dispersity in aqueous solution. Moreover, the MoS2 nanosheets show monodispersity with the most probable size of about 90 nm (Fig. 1C).
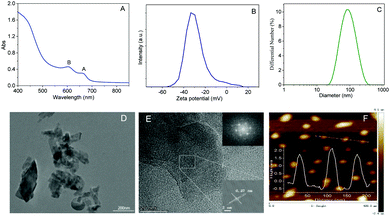 |
| Fig. 1 (A) Absorption spectrum, (B) zeta potential distribution, (C) size distribution, (D) TEM image, (E) HRTEM and (F) AFM images of MoS2 nanosheets exfoliated by sodium phytate. Insets of (E) and (F) are the SAED pattern and the height profile of MoS2 nanosheets exfoliated by sodium phytate, respectively. The concentration of MoS2 nanosheets in (A) is 1.12 mg mL−1. | |
As observed from the low-resolution TEM image (Fig. 1D), MoS2 nanosheets are composed of sheet-like structures from monolayers to few layers with lateral sizes from several dozens to a few hundreds of nanometers. The ordered lattice and parallel fringe can be clearly observed in the high-resolution TEM (HRTEM) image (Fig. 1E). The lattice spacing of 0.27 nm is indexed to the (100) plane of MoS2.10,27,30 The selected area electron diffraction (SAED) pattern (inset of Fig. 1E) further confirms the single crystalline nature of the hexagonal symmetry structure of MoS2 nanosheets. The thickness of the MoS2 nanosheets is less than 2 nm, which is observed from the AFM image (Fig. 1F). According to the Bragg equation, the interlayer distance of the MoS2 nanosheets was calculated to be 0.62 nm.23,33 Therefore, the layer number is about 2 to 3, which is in agreement with the result from the absorption spectrum.
As shown in the XRD patterns (Fig. S2A, ESI†), MoS2 nanosheets maintain high crystallinity as bulk MoS2 powder, indicating that there is no phase conversion during ultrasonic exfoliation. The diffraction peaks at 14.4°, 32.7°, 39.6°, 44.3°, 49.8°, 58.3° and 60.4° correspond to the (002), (100), (103), (006), (105), (110) and (008) crystal faces of 2H-MoS2 (JCPDS No. 24-0513), respectively. The relative intensity of the (002) peaks in the MoS2 nanosheets exfoliated by sodium phytate is weaker than bulk MoS2 powder, indicating the successful exfoliation of the MoS2 nanosheets.34 Raman spectra (Fig. S2B, ESI†) show the characteristic in-plane (E12g) and out-of-plane (A1g) vibrational modes of MoS2 at about 380 cm−1 and 405 cm−1.11,23 Compared with bulk MoS2 powder, the vibration modes of MoS2 nanosheets are red-shifted due to the weakening of the van der Waals force between the layers,35 which also suggests the successful exfoliation of bulk MoS2 into few-layered nanosheets.36 There is also a rather weak second-order scattering process near 450 cm−1, attributed to the longitudinal acoustic vibrational mode of the 2H phase monolayer MoS2 nanosheets.37
The survey XPS spectrum (Fig. 2A) indicates there are Mo, S, P, C and O elements in the MoS2 nanosheets exfoliated by phytate, indicating that phytate is functionalized on the surface of the MoS2 nanosheets. In the Mo 3d core-level XPS spectrum of MoS2 nanosheets exfoliated by sodium phytate (Fig. 2B), the peaks at 229.3 eV and 232.5 eV can be attributed to Mo4+ 3d5/2 and Mo4+ 3d3/2 of 2H-MoS2. The weak peaks at 235.7 eV and 233.0 eV can be ascribed to Mo6+ 3d3/2 and Mo6+ 3d5/2, respectively. This also can be observed in the Mo 3d core-level XPS spectra of the MoS2 nanosheets exfoliated by H2O and bulk MoS2 powder, and may come from MoO3 or MoO42−,23,30 indicating that the surface of the MoS2 nanosheets is partially oxidized on exposure to air.23,38 The weak feature peak at 226.6 eV originated from the S 2s.39 In the S 2p core-level XPS spectrum (Fig. 2C), the peaks at 162.2 eV and 163.4 eV can be attributed to S 2p3/2 and S 2p1/2, respectively. In addition, there exists a weak peak at 133.8 eV (Fig. 2D), corresponding to the binding energy of P 2p. From the above discussion, there exists a weak peak at 133.8 eV (Fig. 2D), corresponding to the binding energy of P 2p. From the above discussion, the MoS2 nanosheets are successfully exfoliated from bulk MoS2 powder in sodium phytate solution, and the phytate molecules are adsorbed onto the surface of the MoS2 nanosheets.
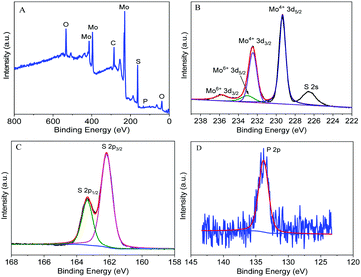 |
| Fig. 2 The survey (A), Mo 3d (B), S 2p (C) and P 2p (D) core-level XPS spectra of MoS2 nanosheets exfoliated by sodium phytate. | |
3.3. Exfoliation mechanism
To investigate the effect of phytate on the exfoliation of MoS2 nanosheets, bulk MoS2 powder was also ultrasonically exfoliated in Na2HPO4 solution and ultra-pure water with the same ultrasonic time. The exfoliation yield is positively correlated with the absorption intensity of the MoS2 nanosheets solution. As shown in Fig. S3 (ESI†), the exfoliation yield is on the order of ultra-pure water < Na2HPO4 solution < sodium phytate. The exfoliation yield in the Na2HPO4 solution is higher than that in ultra-pure water, indicating that the phosphate ion can promote the exfoliation of the MoS2 nanosheets. The exfoliation yield in sodium phytate is higher than that in Na2HPO4 solution, even though the molar concentration of Na2HPO4 is nearly 20-folds that of sodium phytate. There are six phosphate groups in the phytate molecule, which is more efficient than Na2HPO4 in adsorbing on the surface of the MoS2 nanosheets and inserting into the interlayers of bulk MoS2, resulting in high exfoliation yield. The exfoliation process of bulk MoS2 by sodium phytate is proposed in Scheme 1. The van der Waals force between each layer of bulk MoS2 is destroyed by ultrasonication, and phytate molecules intercalate into the edges of the crystal interlayers to facilitate the production of MoS2 nanosheets. After exfoliation, the adsorption of phytate on the MoS2 nanosheets can be stably dispersed in water.
3.4. Adsorption of Hg2+ by MoS2 nanosheets
The strong soft–soft interaction between Hg2+ and S atoms and the powerful chelation effect of phytate on the surface of the MoS2 nanosheets inspired us to utilize MoS2 nanosheets as adsorbents for the removal of Hg2+. The removal efficiency (RE) and adsorption capacity (qe) were calculated from eqn (1) and (2), respectively. |  | (1) |
| 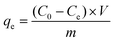 | (2) |
where C0 (μg mL−1) is the initial concentration of Hg2+, Ce (μg mL−1) is the equilibrium concentration of Hg2+ after adsorption, qe (mg g−1) is the adsorption capacity of the MoS2 nanosheets at equilibrium time, V (mL) is the solution volume, and m (g) is the mass of the adsorbent.
The effect of pH on the adsorption of Hg2+ by MoS2 nanosheets exfoliated by sodium phytate was first investigated. As shown in Fig. S4 (ESI†), the adsorption capacity is basically unchanged over the pH range of 4.0–6.0. When the pH is higher than 6.0, the adsorption capacity is slightly decreased due to the hydrolysis of Hg2+. Therefore, the following adsorption experiments were conducted under acidic condition. The effect of the initial concentration of Hg2+ on the adsorption by MoS2 nanosheets was investigated. As shown in Fig. 3A, the adsorption amount is gradually increased with the increasing initial concentration of Hg2+, and the adsorption reaches equilibrium after 1 h. However, the RE (Fig. S5, ESI†) is almost unchanged due to the low initial Hg2+ concentration (C0). The adsorption kinetics was fitted by the pseudo-second-order kinetic model (3).11,13
| 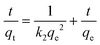 | (3) |
k2 (g mg
−1 h
−1) is the pseudo-second-order adsorption rate constant,
qt (mg g
−1) is the adsorption amount of Hg
2+ at time
t (h), and
qe (mg g
−1) is the adsorption capacity at equilibrium. As shown in
Fig. 3B and Table S2 (ESI
†), the calculated values (
qe,cal) are close to the experimental values (
qe,exp), which indicates that the adsorption of Hg
2+ by MoS
2 nanosheets is mainly chemical adsorption.
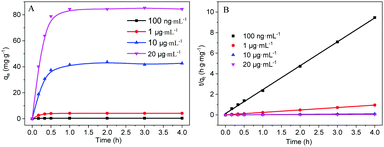 |
| Fig. 3 (A) Adsorption of Hg2+ by MoS2 nanosheets exfoliated by sodium phytate with different initial concentrations of Hg2+. (B) Pseudo-second-order kinetic curves for Hg2+ adsorption. | |
The adsorption thermodynamics was fitted by the Langmuir isotherm model (4).11,13
| 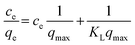 | (4) |
ce is the equilibrium concentration (μg mL
−1) of Hg
2+.
qe and
qmax are the equilibrium adsorption capacity and the maximum adsorption capacity (mg g
−1), respectively.
KL is the Langmuir constant. Three temperatures (25 °C, 30 °C, 40 °C) were selected to investigate the adsorption thermodynamics. The fitting results are shown in Fig. S6 (ESI
†) and listed in Table S3 (ESI
†). The adsorption isotherm fitted the Langmuir model well, indicating that the adsorption of Hg
2+ is a monolayer adsorption. The adsorption thermodynamics also indicates that the low temperature is in favor of Hg
2+ adsorption and the highest
qmax (313.48 mg g
−1) was obtained at room temperature (25 °C). The Langmuir isotherm model was also used to compare the maximum adsorption capacity of MoS
2 nanosheets exfoliated by sodium phytate, and MoS
2 nanosheets exfoliated by H
2O and bulk MoS
2 powder. The results are listed in Table S4 (ESI
†), The
qmax value obtained from the MoS
2 nanosheets exfoliated by sodium phytate is about 7.5 times that of the MoS
2 powder, and about 3.7 times that of the MoS
2 nanosheets exfoliated by H
2O. This result indicates that besides the complexation of Hg
2+ with the intrinsic S atoms of the MoS
2 nanosheets,
40 the chelating effect of phytate on the surface of the MoS
2 nanosheets plays the main role in Hg
2+ adsorption. The interaction between the adsorbed Hg
2+ ions and MoS
2 nanosheets exfoliated by sodium phytate were further confirmed by XPS spectrum. As shown in Fig. S7 (ESI
†), a new element (Hg) appears in the survey XPS spectrum of MoS
2 nanosheets after Hg
2+ adsorption. Furthermore, a new peak at 161.9 eV related to S 2p
1/2 of HgS is observed in the S 2p core-level XPS spectrum, and the peaks at 100.9 eV and 104.9 eV are attributed to Hg
2+ 4f
7/2 and Hg
2+ 4f
5/2 of HgS in the Hg 4f core-level XPS spectrum,
41 which indicate the complex between S and Hg
2+. The chelating effect of phytate can be verified by the complex between O and Hg
2+. In the Hg 4f core-level XPS spectrum, the peaks at 102.0 eV and 106.0 eV are assigned to HgO.
12 In the O 1s core-level XPS spectrum, the peaks at 530.7 eV and 532.5 eV are assigned to MoO
3 and HgO, respectively, after Hg
2+ adsorption.
11 However, without adsorption of Hg
2+, the peaks at 530.4 eV and 531.6 eV in the O 1s core-level XPS spectrum might be deconvoluted into MoO
3 and the adsorbed water, respectively.
11
The adsorption of MoS2 nanosheets exfoliated by sodium phytate toward other heavy metal ions (Pb2+, Cd2+, Cr3+, Mn2+ and Zn2+) and anions (NO2−, NO3−, SO42− and CO32−) and their mixture with Hg2+ were investigated. As shown in Fig. S8 (ESI†), the MoS2 nanosheets show a certain adsorption on Pb2+ and a small amount of adsorption on Cd2+, Cr3+, Mn2+ and Zn2+. However, the MoS2 nanosheets show the best adsorption on Hg2+. Moreover, the co-existing heavy metal ions and anions almost do not affect the adsorption of Hg2+. In the co-existence of heavy ions and anions, Hg2+ (100 ng mL−1) still could be reduced to less than 1 ng mL−1, which is the permission limit of mercury in drinking water provided by the national standard of China. These results indicate the high selectivity of MoS2 nanosheets exfoliated by sodium phytate for Hg2+ adsorption due to the high affinity between Hg2+ and S on the surface of MoS2 nanosheets.10,11
3.5. Cell detoxification application
Before the cell detoxification experiment, MTT experiments were conducted to detect the cytotoxicity of MoS2 nanosheets exfoliated by sodium phytate, Hg2+ and DMSA. As shown in Fig. S9A (ESI†), the HepG2 cells maintain more than 95% cell proliferation capacity even though the concentration of the MoS2 nanosheets is up to 250 μg mL−1, indicating that the MoS2 nanosheets are highly biocompatible and have low toxicity. Hg2+ can induce a significant cytotoxicity in a dose-dependent manner (Fig. S9B, ESI†). Even low concentrations of Hg2+ can cause intoxication in HepG2 cells. 2 μg mL−1 and 5 μg mL−1 Hg2+ resulted in the decrease of proliferation capacities of HepG2 cells to less than 70% and 30%, respectively. In addition, DMSA showed low cytotoxicity. The proliferation capacity was more than 90% in the DMSA concentration range of 0–200 μg mL−1 (Fig. S9C, ESI†).
The high adsorption capacity for Hg2+ and low cytotoxicity of MoS2 nanosheets inspired us to investigate their detoxification effect on Hg2+ poisoning. HepG2 cells were incubated with Hg2+ (2 μg mL−1) to induce moderate cytotoxicity (∼70% cell viability), and then incubated with different concentrations of MoS2 nanosheets exfoliated by sodium phytate. As shown in Fig. 4, with the increasing concentration of MoS2 nanosheets, the proliferation capacities of HepG2 cells gradually increased. When the concentration of MoS2 nanosheets reached 200 μg mL−1, the proliferation capacity of HepG2 cells was greater than 90%, which indicated that the cytotoxicity induced by Hg2+ was almost eliminated because the proliferation capacity of the HepG2 cells is comparable with that observed only in the presence of 200 μg mL−1 MoS2 nanosheets. Compared to the detoxifier DMSA, the MoS2 nanosheets show a similar detoxification effect, which indicates that the MoS2 nanosheets exfoliated by sodium phytate can be used as a potential detoxifier for Hg2+ poisoning.
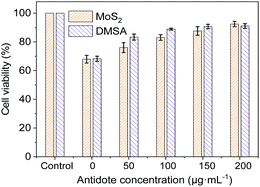 |
| Fig. 4 Viability of HepG2 cells pre-treated with Hg2+ (2 μg mL−1) for 2 h followed by incubation with different concentrations of MoS2 nanosheets exfoliated by sodium phytate or DMSA for 12 h. The control was normal HepG2 cells. | |
4. Conclusions
In summary, the simultaneous exfoliation and functionalization of few-layered MoS2 nanosheets were achieved via ultrasonic treatment by using sodium phytate as an auxiliary reagent. The functionalization of phytate not only can facilitate the exfoliation of MoS2 nanosheets, but also endows MoS2 nanosheets with high stability in aqueous solution over a broad pH range. This preparation procedure is simple, green, cost-effective, as well as high yield. The as-prepared MoS2 nanosheets show the effective and selective adsorption for Hg2+ in water, and can reduce Hg2+ ion (100 ng mL−1) to a concentration below the Chinese national permission limit of mercury (1 ng mL−1) in drinking water. The as-prepared MoS2 nanosheets also displayed low cytotoxicity for HepG2 cells, and showed a detoxification effect similar to that of the common detoxifier DMSA. The study may open a new application direction of TMDC in the development of novel therapeutics for the treatment of heavy metal poisoning.
Author contributions
Shanshan Xing: conceptualization, validation, investigation, data curation, writing – original draft; Chunqiu Xia: methodology, investigation, writing – original draft; Xinyi Liu: methodology, data curation; Liangqia Guo: conceptualization, funding acquisition, writing – review & editing, supervision; Fengfu Fu: conceptualization, writing – review & editing.
Conflicts of interest
There are no conflicts to declare.
Acknowledgements
This work was supported by the National Key Research and Development Program of China (2017YFC1600500), and the National Natural Science Foundation of China (21874023).
Notes and references
- H. H. Harris, I. J. Pickering and G. N. George, Science, 2003, 301, 1203 CrossRef CAS PubMed.
- L. Trasande, P. J. Landrigan and C. Schechter, Environ. Health Perspect., 2005, 113, 590–596 CrossRef CAS PubMed.
- Y. Huang, M. X. Wang, Z. J. Li, Y. Y. Gong and E. Y. Zeng, J. Hazard. Mater., 2019, 373, 783–790 CrossRef CAS PubMed.
- B. Manna and C. R. Raj, ACS Sustainable Chem. Eng., 2018, 6, 6175–6182 CrossRef CAS.
- J. P. Tang, C. J. Ptacek, D. W. Blowes, Y. Y. Liu, Y. Feng, Y. Z. Finfrock and P. Liu, Chem. Eng. J., 2022, 428, 131362 CrossRef CAS.
- K. Yakkala, S. Chappa, P. B. Rathod, R. N. Gurijala and A. K. Pandey, Mater. Today Chem., 2021, 22, 100507 Search PubMed.
- L. H. Dong, L. A. Hou, Z. S. Wang, P. Gu, G. Y. Chen and R. F. Jiang, J. Hazard. Mater., 2018, 359, 76–84 CrossRef CAS PubMed.
- L. X. Chen, Y. Wang, Y. S. Wan, Y. M. Cai, Y. Q. Xiong, Z. W. Fan, S. D. Conradson, H. Y. Fu, L. H. Yuan and W. Feng, Chem. Eng. J., 2020, 387, 124087 CrossRef CAS.
- X. Zhang, Z. C. Lai, C. L. Tan and H. Zhang, Angew. Chem., Int. Ed., 2016, 55, 8816–8838 CrossRef CAS PubMed.
- K. Ai, C. P. Ruan, M. X. Shen and L. H. Lu, Adv. Funct. Mater., 2016, 26, 5542–5549 CrossRef CAS.
- F. F. Jia, Q. M. Wang, J. S. Wu, Y. M. Li and S. X. Song, ACS Sustainable Chem. Eng., 2017, 5, 7410–7419 CrossRef CAS.
- W. Zhan, F. Jia, Y. Yuan, C. Liu, K. Sun, B. Yang and S. Song, J. Hazard. Mater., 2020, 384, 121382 CrossRef CAS PubMed.
- L. H. Zhi, W. Zuo, F. J. Chen and B. D. Wang, ACS Sustainable Chem. Eng., 2016, 4, 3398–3408 CrossRef CAS.
- Y. Song, M. Lu, B. Huang, D. Wang, G. Wang and L. Zhou, J. Alloys Compd., 2018, 737, 113–121 CrossRef CAS.
- P. Gao, J. Lei, J. Tan, G. Wang, H. Liu and L. Zhou, Compos. Commun., 2021, 25, 100736 CrossRef.
- E. D. Grayfer, M. N. Kozlova and V. E. Fedorov, Adv. Colloid Interface Sci., 2017, 245, 40–61 CrossRef CAS PubMed.
- Z. Y. Zeng, Z. Y. Yin, X. Huang, H. Li, Q. Y. He, G. Lu, F. Boey and H. Zhang, Angew. Chem., Int. Ed., 2011, 50, 11093–11097 CrossRef CAS PubMed.
- A. Ambrosi, Z. Sofer and M. Pumera, Small, 2015, 11, 605–612 CrossRef CAS PubMed.
- Y. Wang, Y. Zhou, G. Xie, J. Li, Y. Wang, X. Liu and Z. Zang, ACS Appl. Mater. Interfaces, 2021, 13, 25250–25259 CrossRef CAS PubMed.
- D. Wang, F. Wu, Y. Song, C. Li and L. Zhou, J. Alloys Compd., 2017, 728, 1030–1036 CrossRef CAS.
- M. Vera-Hidalgo, E. Giovanelli, C. Navío and E. M. Pérez, J. Am. Chem. Soc., 2019, 141, 3767–3771 CrossRef CAS PubMed.
- A. Jawaid, D. Nepal, K. Park, M. Jespersen, A. Qualley, P. Mirau, L. F. Drummy and R. A. Vaia, J. Am. Chem. Soc., 2016, 28, 337–348 CAS.
- X. Hai, K. Chang, H. Pang, M. Li, P. Li, H. M. Liu, L. Shi and J. H. Ye, J. Am. Chem. Soc., 2016, 138, 14962–14969 CrossRef CAS PubMed.
- S. Karunakaran, S. Pandit, B. Basu and M. De, J. Am. Chem. Soc., 2018, 140, 12634–12644 CrossRef CAS PubMed.
- J. F. Shen, Y. Pei, M. Wang, Y. C. Ge, P. Dong, J. H. Yuan, R. Baines, P. M. Ajayan and M. X. Ye, Adv. Mater. Interfaces, 2017, 4, 1600847 CrossRef.
- G. J. Guan, S. Y. Zhang, S. Liu, Y. Q. Cai, M. Low, C. P. Teng, I. Y. Phang, Y. Cheng, K. L. Duei, B. M. Srinivasan, Y. Zhang, Y. W. Zhang and M. Y. Han, J. Am. Chem. Soc., 2015, 137, 6152–6155 CrossRef CAS PubMed.
- W. Xing, Y. S. Chen, X. X. Wu, X. Z. Xu, P. Ye, T. Zhu, Q. Y. Guo, L. Q. Yang, W. W. Li and H. Huang, Adv. Funct. Mater., 2017, 27, 1701622 CrossRef.
- N. K. Oh, H. J. Lee, K. Choi, J. Seo, U. Kim, J. Lee, Y. Choi, S. Jung, J. H. Lee, H. S. Shin and H. Park, Chem. Mater., 2018, 30, 4658–4666 CrossRef CAS.
- X. W. Wang and P. Y. Wu, ACS Appl. Mater. Interfaces, 2018, 10, 2504–2514 CrossRef CAS PubMed.
- X. L. Liu, H. Chen, J. Lin, Y. Li and L. Q. Guo, Chem. Commun., 2019, 55, 2972–2975 RSC.
- C. Backes, R. J. Smith, N. McEvoy, N. C. Berner, D. McCloskey, H. C. Nerl, A. O’Neill, P. J. King, T. Higgins, D. Hanlon, N. Scheuschner, J. Maultzsch, L. Houben, G. S. Duesberg, J. F. Donegan, V. Nicolosi and J. N. Coleman, Nat. Commun., 2014, 5, 4576 CrossRef CAS PubMed.
- C. V. Sijla Rosely, A. M. Joseph, A. Leuteritz and E. Bhoje Gowd, ACS Sustainable Chem. Eng., 2020, 8, 1868–1878 CrossRef.
- B. Radisavljevic, A. Radenovic, J. Brivio, V. Giacometti and A. Kis, Nat. Nanotechnol., 2011, 6, 147–150 CrossRef CAS PubMed.
- D. D. Xuan, Y. Zhou, W. Nie and P. Chen, Carbohydr. Polym., 2017, 155, 40–48 CrossRef CAS PubMed.
- S. Park, A. T. Garcia-Esparza, H. Abroshan, B. Abraham, J. Vinson, A. Gallo, D. Nordlund, J. Park, T. R. Kim, L. Vallez, R. Alonso-Mori, D. Sokaras and X. L. Zheng, Adv. Sci., 2021, 8, 2002768 CrossRef CAS PubMed.
- H. L. Wang, P. f. Cheng, J. Shi, D. Wang, H. G. Wang, J. Pezoldt, M. Stich, R. f. Chen, A. P. A. van, W. Huang and P. Schaaf, Green Chem., 2021, 23, 3642–3648 RSC.
- F. I. Alzakia, W. Jonhson, J. Ding and S. C. Tan, ACS Appl. Mater. Interfaces, 2020, 12, 28840–28851 CrossRef CAS PubMed.
- A. A. Graf, M. J. Large, S. P. Ogilvie, Y. Rong, P. J. Lynch, G. Fratta, S. Ray, A. Shmeliov, V. Nicolosi, R. Arenal, A. A. K. King and A. B. Dalton, Nanoscale, 2019, 11, 15550–15560 RSC.
- J. I. Paredes, J. M. Munuera, S. Villar-Rodil, L. Guardia, M. Ayán-Varela, A. Pagán, S. D. Aznar-Cervantes, J. L. Cenis, A. Martínez-Alonso and J. M. D. Tascón, ACS Appl. Mater. Interfaces, 2016, 8, 27974–27986 CrossRef CAS PubMed.
- F. F. Jia, X. Zhang and S. X. Song, Phys. Chem. Chem. Phys., 2017, 19, 3837–3844 RSC.
- R. Aswathi and K. Y. Sandhya, J. Mater. Chem. A, 2018, 6, 14602 RSC.
Footnote |
† Electronic supplementary information (ESI) available: Adsorption spectra of MoS2 nanosheets at different conditions, XRD pattern, Raman spectra, adsorption spectra of MoS2 nanosheets with different reagents, effect of pH on the adsorption amount, Langmuir adsorption isotherms, XPS spectra of MoS2 nanosheets, adsorption selectivity, viability of HepG2 cells, comparison of yields of MoS2 nanosheets, adsorption dynamics model parameters, the maximal adsorption capacity and correlation coefficient of Langmuir isotherms, comparison of the maximum adsorption capacity of MoS2 for Hg2+. See DOI: 10.1039/d2ma00058j |
|
This journal is © The Royal Society of Chemistry 2022 |