DOI:
10.1039/D1MA01227D
(Paper)
Mater. Adv., 2022,
3, 2495-2504
A fluorescent colorimetric vanillin di-Schiff base chemosensor for detection of Cu(II) and isolation of trinuclear Cu(II)–dihydrazide†
Received
22nd December 2021
, Accepted 26th January 2022
First published on 26th January 2022
Abstract
In this work, we developed a simple fluorescent colorimetric chemosensor H2L [6,6′-((1,1′)-hydrazine-1,2-diylidene bis(methanylylidene)) bis(2-(6-methoxy)) phenol] for rapid detection of Cu2+ in aqueous solution. The method for synthesis of H2L is very simple and environment friendly. This organic Schiff base probe was characterized by 1H-NMR, FT-IR and ESI-MS spectroscopy along with single-crystal XRD analysis. It exhibited binding-induced colour change with Cu2+ion from colourless to intense yellow and fluorescence enhancement. The LOD values of H2L towards Cu2+ were calculated to be 7.1 × 10−8 M (colorimetrically) and 3.8 × 10−8 M (fluorometrically). The interactions between H2L and Cu2+ were studied by Job's plot, ESI-MS, FT-IR spectroscopy and DFT calculations. The crystal structure of the L–Cu2+ adduct was also determined by single-crystal X-ray analysis, and it was found that two molecules of L coordinate with three molecules of Cu2+ ions. The receptor H2L could operate in a wide pH range and can be successfully applied for detection and quantification of Cu2+ ions in environmental samples and logic applications.
Introduction
Copper(II) is one of the essential metal ions in the human body, and it plays an important role in various biological and environmental processes.1–5 The first-row transition element Cu is the third most important tracer element in biological processes, and Cu concentrations up to 1.3 mg L−1 in drinking water are tolerable, according to the U.S. Environmental Protection Agency (EPA).6–8 Cu(II) is present in hemocyanin, which functions as oxygen transporter in insects. In the human body, Cu(II) is present in several enzymes, including superoxide dismutase, tyrosinase, and dopamine hydroxylase. In cytochrome c oxidase and ferritin, Cu(II) presents in combination with another metal, iron (Fe2+). Although excess copper can result in mitochondrial damage, DNA breakage, neuronal injury, and Alzheimer's and Wilson's diseases, deficiency of Cu(II) causes serious illness such as anemia, as it is an important part of ceruloplasmin.9–13 Major concentrations of Cu(II) are found in the liver, kidney, heart and brain. Cu(II) is a key component in the body needed for regulation of many biological functions. However, its presence in excess affects some biologically important enzymes by binding with their active sites and displacing other nutrient minerals.14–17 Cu(II) can disturb nutrient absorption and transport, thus destroying plant and aquatic ecosystems.18–20
Conventional methods such as atomic absorption spectrometry (AAS),21,22 inductively coupled plasma–mass spectrometry (ICP-MS),23 flame atomic absorption spectrometry (FAAS)24,25 and electrochemical assays26,27 are generally used for the detection of metal ions like Cu2+. Although these traditional methods are responsive, accurate and selective, they require special sampling techniques, manual operation, and professional handling, and they are time consuming, expensive and sometime inconclusive. To overcome these problems, researchers are searching for easily employable optical sensors.
Optical sensors have several advantages such as sensitivity, selectivity, cost effectiveness and simplicity.28,29 Colorimetric and fluorometric optical chemosensors offer real-time analyte recognition, easy operation, controllability and lower response time.30–34 A variety of colorimetric and fluorometric sensors for Cu(II)35–44 have been reported. Despite these, considering the effectiveness and low detection limit, the design and synthesis of more effective chemosensors are still desirable.
In our ongoing research, we have already reported several chemosensors for Cu(II) based on amides,45 triazole,46 dihydrazone,47 dihydrophenylquinazolinone,48 thiosemicarbazone,49 and benzohydrazide.50,51 Taking another step in the process of searching for suitable chemosensors, we focused on dihydrazone-based chemosensors. Herein, we have successfully designed and synthesized 6,6′-((1,1′)-hydrazine-1,2-diylidene bis(methanylylidene)) bis(2-(6-methoxy)) phenol (H2L), a dihydrazone of ortho-vanillin and hydrazine, which selectively and sensitively detects Cu2+ both colorimetrically and fluorometrically among a series of different metal ions.
Experimental
General information
All the required materials used for synthesis were obtained from Sigma-Aldrich and directly used. Analytical-grade solvents were used for the overall experiments, and freshly prepared double-deionized water was used for dilution and preparing tris HCl buffer (10 μM, pH = 7.2) solution. The metal ion solutions were prepared from their nitrate salts. 1H NMR and 13C NMR spectra were recorded on a Bruker DRX spectrometer operating at 400 MHz in CDCl3 solvent, and chemical shifts were recorded in ppm relative to TMS. Melting point was determined on an X-4 digital melting-point apparatus and was not corrected. Absorption spectra were recorded on a Shimadzu UV 1800 spectrophotometer using 10 mm path length quartz cuvettes with the wavelength in the range of 200–800 nm. Fluorescence spectra were recorded on a Hitachi spectrophotometer. Electrospray ionisation mass spectra (ESI-MS) were recorded on a Waters mass spectrometer using the mixed HPLC grade solvent methanol and triple distilled water. The pH adjustments were monitored using a digital pH meter (Merck) in buffer solution and adjusting with dilute hydrochloric acid and sodium hydroxide. Solutions of the receptor H2L (1 × 10−5 M) and metal salts (1 × 10−4 M) were prepared in methanol–tris-HCl buffer (10 mM, pH 7.2) medium (1
:
1 v/v) and H2O, respectively.
X-Ray data collection and structure determination
Single-crystal X-ray data were collected using MoKα (λ = 0.7107 Å) radiation on a Bruker APEX II diffractometer equipped with a CCD area detector. Data collection, data reduction, and structure solution/refinement were carried out using the software package of SMART APEX.52 The structures were solved by direct methods (SHELXS-97) and standard Fourier techniques, and refined on F2 using full-matrix least-squares procedures (SHELXL-97) using the SHELX-97 package53 incorporated in WinGX.54 In most cases, non-hydrogen atoms were treated anisotropically. Hydrogen atoms were fixed geometrically at their calculated positions following the riding atom model. The crystallographic data of H2L and its Cu(II) complex (1) are listed in Table 1. Structural information for H2L and 1 was deposited at the Cambridge Crystallographic Data Center (CCDC 2087278 and 2087279, respectively).
Table 1 Crystallographic data and refinement parameters of the probe H2L and its trinuclear Cu(II) complex (1)
Identification code |
H2L
|
1
|
Empirical formula |
C16H16N2O4 |
C32H32Cu3N6O16 |
CCDC number |
2087278
|
2087279
|
Formula weight |
300.31 |
947.25 |
Temperature/K |
296.15 |
296.45 |
Crystal system |
Monoclinic |
Triclinic |
Space group |
P21 |
P![[1 with combining macron]](https://www.rsc.org/images/entities/char_0031_0304.gif) |
a/Å |
5.701(3) |
8.157(7) |
b/Å |
17.733(10) |
9.902(9) |
c/Å |
6.535(4) |
11.983(9) |
α/° |
90 |
75.79(2) |
β/° |
106.388(9) |
85.79(2) |
γ/° |
90 |
87.08(3) |
Volume/Å3 |
633.8(6) |
935.2(14) |
Z
|
2 |
1 |
ρ
calc /g cm−3 |
1.574 |
1.682 |
μ/mm−1 |
0.115 |
1.771 |
F(000) |
316.0 |
481.0 |
Crystal size/mm3 |
0.28 × 0.21 × 0.19 |
0.32 × 0.24 × 0.18 |
Radiation |
MoKα (λ = 0.71073) |
MoKα (λ = 0.71073) |
2θ range for data collection/° |
4.594 to 53.832 |
4.81 to 50.75 |
Index ranges |
−7 ≤ h ≤ 7, −22 ≤ k ≤ 22, −8 ≤ l ≤ 8 |
−9 ≤ h ≤ 9, −11 ≤ k ≤ 10, −14 ≤ l ≤ 14 |
Reflections collected |
8607 |
6361 |
Independent reflections |
2687 [Rint = 0.0634, Rsigma = 0.0851] |
3325 [Rint = 0.0866, Rsigma = 0.1465] |
Data/restraints/parameters |
2687/13/203 |
3325/0/262 |
Goodness-of-fit on F2 |
1.240 |
1.024 |
Final R indexes [I >=2σ (I)] |
R
1 = 0.0537, wR2 = 0.1114 |
R
1 = 0.0748, wR2 = 0.1706 |
Final R indexes [all data] |
R
1 = 0.1483, wR2 = 0.1456 |
R
1 = 0.1347, wR2 = 0.2081 |
Largest diff. peak/hole/e Å−3 |
0.21/−0.25 |
0.71/−0.84 |
Synthesis of H2L
The ligand was synthesized by following a reported procedure.55o-Vanillin (0.304 g, 2 mmol) was dissolved in 10 mL dry methanol, and to this 0.05 g hydrazine hydrate (1 mmol) was added dropwise with constant stirring. Then, the reaction mixture was refluxed for 4 h under dry condition. After reflux, the reaction mixture was kept in air for evaporation; a light-yellow solid crystal separated out. Yield, 0.255 g, 85%. Anal. calc. For C16H16N2O4: C, 63.99; H, 5.37; N, 9.33%. Found: C, 63.86; H, 5.49; N, 9.26%. ESI–MS: m/z 301.1 (H3L+) (Fig. S1, ESI†). FTIR/cm−1 (KBr): 3275 (vb, O–H), 1662 (vs, C
N), 1589 (m), 1446 (m), 1217 (s), 984 (m), 864 (m), 686 (s) (Fig. S2, ESI†).1H NMR (400 MHz, CDCl3, TMS): δ 11.55 (s, 2H, –OH), 8.71 (s, 2H, –C
NH), 7.20–6.91 (m, 6H, ArH), 3.95 (s,6H, OCH3) (Fig. S3, ESI†).13C NMR (100 MHz, CDCl3, TMS): δ 164.79, 149.75, 148.39, 124.08, 119.42, 117.39, 115.22, 56.24 (Fig. S4, ESI†).
Synthesis of H2L–Cu2+ complex [Cu3L2(NO3)2(H2O)2]) (1)
An aqueous–methanolic solution (10 mL, 1
:
1 v/v) of Cu(NO3)2·9H2O (0.166 g, 0.5 mmol) was added dropwise to the hot stirring solution of H2L (0.30 g, 1 mmol) in methanol–H2O (10 mL, 1
:
1 v/v). Immediate colour change was observed from colourless to reddish yellow. The solution was then stirred for 5 min at room temperature. The clear solution was kept for evaporation to obtain a red crude product. Yield, 0.510 g, 53.61%. The obtained product was recrystallised through diffusion from different phases via making a series of setups with different concentrations in DCM and diethyl ether; a red crystal suitable for single-crystal XRD analysis was obtained. Anal. calc. for C32H34N6O16Cu3: C, 40.49; H, 3.61; N, 8.85%. Found: C, 40.65; H, 4.43; N, 8.97%. ESI–MS: m/z 722.5 (2L + 3Cu2+) (Fig. S5, ESI†). FTIR/cm−1 (KBr): 3064 (wb), 1650 (vs), 2565 (s), 1275 (m), 1178 (s) (Fig. S6, ESI†).
Computational details
The program package GAUSSIAN-09 Revision C.01 was employed for all calculations.56 The gas phase geometries of the compound were fully optimized with symmetry restrictions in singlet ground state and the gradient-corrected DFT level coupled with B3LYP.57 Basis set LanL2DZ was used for the whole molecules H2L and [Cu3L2(NO3)2(H2O)2] (1). The HOMOs and LUMOs of molecular ions were calculated with the same basis set and functional.
Preparation of stock solution for photophysical measurements
Receptor H2L solution was prepared initially at the concentration of 1 × 10−3 M in 10 mL methanol–tris HCl buffer medium (10 mM, pH 7.2) solution (1
:
1 v/v), then diluted to the desired concentration. Stock solutions of guest ions were prepared separately from their nitrate salts (except for the sulphate salt of Fe2+, ammonium heptamolybdate salt for Mo6+ and the potassium salts for Cr6+ and Mn7+) at a concentration of 1 × 10−3 M in 10 mL double-deionised water and further diluted to their desired concentrations. After mixing H2L with each of the metal ions for a few seconds, absorption and fluorescence spectra were obtained at room temperature.
Job's plot measurements
A methanol–tris-HCl buffer (1
:
1 v/v, 10 mM, pH 7.2) solution containing H2L (10 μM) and an aqueous solution of Cu(NO3)2 were prepared separately. Then, the mole ratio of H2L was changed from 0.1 to 0.9 in such a manner that the sum of the total volume of metal ions and H2L remained constant (2 mL). All solutions were diluted to 3 mL. After shaking them for a minute, UV–vis spectra were obtained at room temperature.
Results and discussion
Structure establishment of ligand (H2L)
The condensation reaction between o-vanillin and hydrazine in a 2
:
1 proportion produced Schiff base receptor H2L as a yellow solid in good yield, and it was mainly characterised by elemental analysis, FTIR spectra, ESI-MS, 1H-NMR spectra and single-crystal XRD analysis. The compound showed excellent solubility in common solvents such as acetonitrile (ACN), dimethyl sulphoxide (DMSO), methanol (MeOH) and dichloromethane (DCM) but not in pure water (Scheme 1). In our present study, methanol is used to improve water solubility of the probe H2L. To investigate the stability of ligand H2L in 1
:
1 MeOH–H2O medium at room temperature, ESI-mass spectrum was recorded (Fig. S1, ESI†). Here, the presence of an intense molecular ion peak at m/z = 300.1 confirms the solution stability of H2L. In the FTIR spectra of H2L, the broad band appearing at 3480 cm−1 is due to phenolic OH, and the sharp peak at 1620 cm−1 is due to the C
N group. From the 1H-NMR spectra, characteristic singlet peaks were obtained at 11.62, 9.89 and 1.32 ppm respectively due to –OH, –C
N and –OMe groups, along with peaks between 7.62 to 7.36 ppm for aromatic protons. The compound H2L displayed intense absorption bands at 313 nm and 225 nm, and a small hump at 390 nm, which imparts its very light-yellow color in 1
:
1 aqueous methanol. Here, the band at 313 nm and 225 nm originated because of the n–π* and π–π* electronic transitions. Also, in the fluorescence study of the receptor H2L in this medium, a broad maximum was obtained around 360 nm when excited at 310 nm.
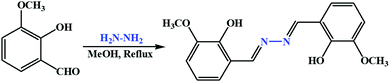 |
| Scheme 1 Synthesis of the probe H2L. | |
Slow evaporation of a moderately concentrated methanolic solution of the probe H2L yielded a fine, needle-shaped crystalline form suitable for single-crystal XRD. The shortest imine-based salen derivative, the probe H2L is a 3-methoxy 2-hydroxy aldazine in which the two units of salicylaldehyde derivative are linked directly through imine nitrogen atoms. There are rotational degrees of freedom about the central N–N bond. H2L is crystallized in the monoclinic system with space group P21. The SCXRD studies revealed that L molecule is planar. The ORTEP view of H2L with the atom numbering scheme is shown in Fig. 1. The bond length and bond angles are within the expected ranges. The methoxy groups, as well as the hydroxyl groups, are anti to each other. So, the molecule of H2L adopts an anti-configuration in solid state. The N1–C1 (1.240 Å) and N1–N2 (1.346 Å) distances indicate agreement to double and single bonds, respectively. The torsion angle N1–C1–C2–C2 [−175.4°] specifies the molecule is essentially planar. Intra-molecular O3–H⋯N1 and symmetry equivalent O4–H⋯N2 hydrogen bonds play an important role in stabilizing the geometry of H2L, and in the crystal, the molecules are interlinked and stabilized through C–H⋯π (C4–C6) interactions in addition to van der Waals forces (Fig. S7, ESI†).
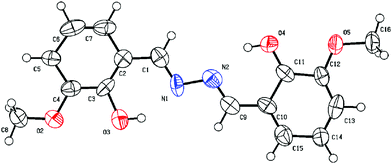 |
| Fig. 1 ORTEP diagrams of H2L showing the atom-labeling scheme and 50% thermal ellipsoids. | |
Single-crystal structure of the Cu(II) complex of H2L (1)
1 crystallized in the triclinic system with space group P1. The SCXRD studies revealed that 1 is trinuclear. The ORTEP view of 1 with the atom numbering scheme is shown in Fig. 2. In the crystal structure of the Cu(II) complex, two different coordination environments of the Cu(II) centres are observed with two di-deprotonated ligands of H2L (C16H16N2O4) and three copper(II) ions. Two peripheral Cu(II) ions are fifth coordinated distorted trigonal bipyramidal, while the inner Cu(II) centre is sixth coordinated octahedral geometry. The TBP geometries of the peripheral Cu(II) ions have also been confirmed by the calculations of Addison parameter value τ = 0.883 (τ = β − α/60).58 Fifth coordination of each of the two peripheral Cu(II) ions is satisfied by one imine–N atom, two phenoxide O atoms, and one O-atom of one –OCH3 group of two different molecules of the ligand H2L and the O atom of the coordinated H2O molecule, with the corresponding bond distances Cu(II)–N(imi) = 1.934 Å, Cu(II)–O(pheoxide 1) = 1.875 Å, Cu(II)–O(pheoxide 2) = 1.974 Å, Cu(II)–O(OCH3) = 2.083 Å and Cu(II)–O(OH2) = 2.122 Å. The two peripheral Cu(II) ions are above and below the plane of the inner Cu(II) centre. The di-deprotonations of the two ligands (H2L) balance the charges of the peripheral Cu(II) centres. The sixth coordination of the two inner Cu(II) ions is satisfied by two imine –N atoms and two phenoxide O atoms of different ligand molecules and two Cu(II)–O(NO3) bonds, with the respective distances of 1.978 Å, 1.949 Å and 2.516 Å. All the bond distances are in good agreement with previously reported structures. Three copper(II) ions are not in a straight line in the complex, and the atomic distance of Cu⋯Cu is 3.344 Å. Both Cu–O–Cu bond angles are 116.96°, whereas the Cu–N–N–Cu torsional angles are 30.18°. The metal ions are surrounded by the organic ligand and do not interact with one another. There is perfect face-to-face π–π stacking with a distance 3.755 Å, and in the crystal structure of 1, there are several OH⋯H hydrogen-bonding interactions between coordinated H2O molecules and NO3 counter-ions (Fig. S8, ESI†).
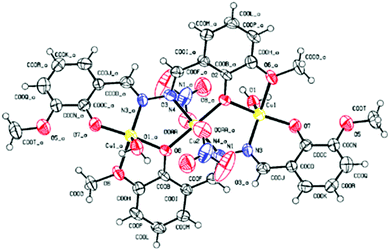 |
| Fig. 2 ORTEP diagrams of 1 showing the atom-labeling scheme and 50% thermal ellipsoids. | |
Absorption selectivity studies
Initially, the selectivity of chemosensor H2L towards various metal ions was observed by naked eyes, along with absorption studies at room temperature in methanol–tris-HCl buffer (1
:
1 v/v, 10 mM, pH 7.2) solution. On systematic addition of 5 equiv. of different metal ions (e.g., Fe3+, Co2+, Ni2+, Cu2+, Zn2+, Cd2+, Hg2+, Pb2+, Mo6+, Cr3+, Ag+, Al3+, Mn2+, Fe2+, Cr6+ and Mn7+) to a fixed amount of H2L, no colour change from colourless to yellow (Fig. 3) and no spectral change (Fig. 4) were observed in all cases except Cu2+ and Mo6+. An intense peak, developed at 274 nm, and increased absorbance intensity at 313 nm for H2L were observed in presence of 5 equiv. Mo6+ ion; in the case of the addition of Cu2+ ion, a new peak developed at 434 nm. In the competitive experiments, the metal (Cu2+)-dependent absorption spectra of H2L was not disturbed in the presence of the remaining metal ions. The characteristic absorption band at 434 nm for H2L–Cu2+ remained almost consistent, with negligible loss of intensity, when 5-fold higher concentrations of interfering ions were added in the host–guest solution. Even the 10-fold higher concentration of Mo6+ ion does not interfere with the absorption of H2L–Cu2+ (Fig. 5).
 |
| Fig. 3 Colour change in visible light of probe H2L on addition of different metal ions. | |
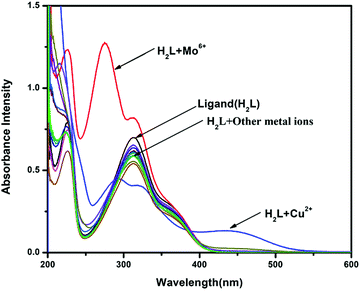 |
| Fig. 4 Absorption spectra of H2L (10 μM) changes in the presence of 5 equiv. of different metal ions. | |
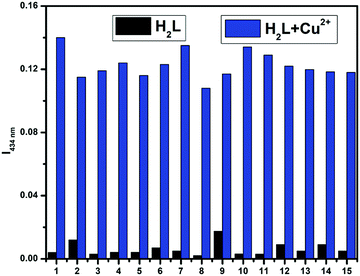 |
| Fig. 5 Competitive experiment of H2L–Cu2+ with other metal ions where 1 = H2L, 2 = Mo6+, 3 = Cd2+, 4 = Pb2+, 5 = Fe3+, 6 = Fe2+, 7 = Ni2+, 8 = Al3+, 9 = Zn2+, 10 = Co2+, 11 = Hg2+, 12 = Cr3+, 13 = Ag+, 14 = Cr6+, 15 = Mn7+. | |
Absorption titration and LOD
In the absorption titration experiments, upon incremental addition of aqueous Cu2+, the absorbance intensity at 313 was steadily decreased with the generation of a new peak at 434 nm, accompanied by 121 nm bathochromic shift. Thus, two clear isosbestic points at 355 nm and 390 nm developed, which indicate that H2L and its Cu2+ adduct are present in equilibrium (Fig. 6a). The limit of detection (LOD) for Cu2+ was calculated from these titration curves by linear fitting of the intensity at 434 nm vs. [Cu2+]. The obtained LOD value is 7.1 × 10−8 M for Cu2+, calculated using the formula 3(SD/S), where ‘SD’ is the measured standard deviation of the blank sample and ‘S’ is the slope of the linear fitted calibration curve (Fig. 6b).
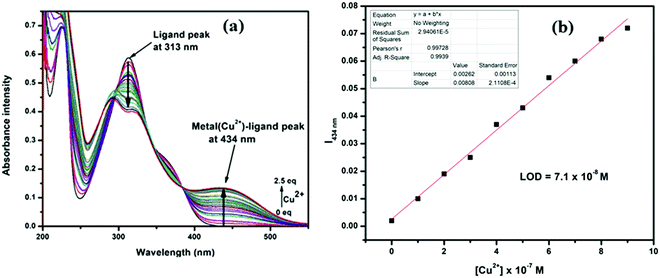 |
| Fig. 6 (a) UV-vis titration of H2L with Cu2+, (b) detection limit. | |
Stoichiometry and association constant
Conventional Job's continuation method of variation indicates that two H2L molecules can coordinate with three Cu2+ ions (Fig. S9, ESI†), which was further established by ESI-MS analysis. The base peak was obtained at m/z = 722.5, which corresponds to [Cu3L2]2+ (Fig. S5, ESI†). To support the Cu3L2 complexation in solid phase, isolation in single-crystal form is also necessary, as it is more acceptable in current research. Thus, sincere efforts were made to isolate the L–Cu2+ complex in the form of single crystal at the experimental condition. Mixing of each receptor–analyte in a 2
:
1 molar ratio, a block-shaped red crystal was separated out, which was suitable for single-crystal XRD (vide supra).
Considering the binding ratio, the association constant was calculated to be 7.7 × 107 M−3/2 by the linear fitting of Benesi–Hildebrand (B–H) equation 1/(A − A0) vs. 1/[Cu2+]3/2 (Fig. S10, ESI†). However, the association constant values for other ions were too low to be calculated. This high binding value indicates the strong affinity of Cu2+ ion towards the proposed chemosensor H2L.
pH Experiment
The analyte binding affinity of receptor H2L is also susceptible to the pH of the working media, as it contains –OH, >C
N, and –OMe groups, which are very sensitive to pH. Thus, receptor solutions with pH ranging from 2 to 13 were prepared in separate vials by adjusting with 0.1 M HCl and 0.1 M NaOH. Hence, each vial contains the same amount of H2L at different pH values; absorption spectra were taken before and after analyte addition. From Fig. 7, the absorbance value of free ligand H2L and its metal complex gradually increases from neutral to basic medium due to effective electrostatic interaction between the negatively charged H2L and the positively charged metal ion. Therefore, all the sensing studies were performed at the physiological pH of 7.2, maintained by using tris-HCl buffer, where the acid–base sensitive groups cannot be disturbed.
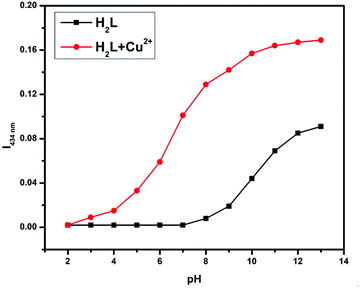 |
| Fig. 7 pH effect of H2L and H2L–Cu2+ at 434 nm. | |
Reversibility studies
The reversible behaviour of the chemosensor H2L was verified using Na2EDTA. When the H2L +Cu2+ adduct was treated with Na2EDTA (Fig. 8), the metal-induced absorption bands at 434 nm immediately vanished, with the simultaneous appearance of the original absorption band of free H2L at 313 nm, accompanied by visual color change from yellow to colourless. With excessive addition of Cu2+ ion into the resultant solution, absorbance intensity was almost recovered. The absorption band switching between H2L and H2L–Cu2+ was regularly observed for more than 5 cycles with negligible loss of intensity. This observation clearly indicates that metal ions coordinate via chelation rather than any acid catalyst irreversible reaction.
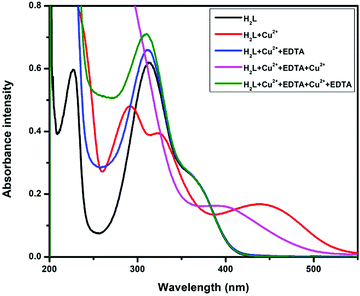 |
| Fig. 8 Reversible investigation of H2L and Cu2+ with EDTA. | |
Fluorescence property
To check the emission selectivity of H2L towards metals, steady-state fluorescence experiments were separately performed with Fe3+, Co2+, Ni2+, Zn2+, Cd2+, Hg2+, Pb2+, Mo6+, Cr3+, Ag+, Al3+, Mn2+, Fe2+, Cr6+ and Mn7+ in methanol–tris-HCl buffer (1
:
1 v/v, 10 mM, pH 7.2) solution. The receptor H2L displayed moderate fluorescence at 360 nm when excited at 310 nm (fluorescence quantum yield, Φ = 0.007). This emission intensity was enhanced only in the presence of Cu2+ ions (fluorescence quantum yield, Φ = 0.013), whereas Mo6+, Hg2+ and Fe3+ ions showed selective quenching behavior (Fig. 9). The other metal ions showed negligible change in the emission spectra of H2L, even in the presence of more than 5-fold higher amounts than Cu2+. In the titration experiments, with gradual addition of Cu2+ ions to the receptor solution, the emission intensity continuously enhanced and became saturated when [Cu2+] reached 1.5 equiv. Here, emission was enhanced due to restriction of both PET and C
N-isomerisation process after metal binding; thus, fluorescence was enhanced due to the chelation enhancement fluorescence (CHEF) process. The fluorometric detection limit was 0.038 μM (Fig. 10), which is slightly different from values obtained from UV-Vis spectra.
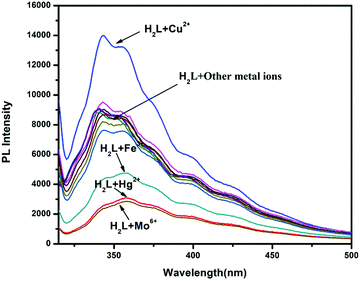 |
| Fig. 9 Fluorescence spectra of H2L with different cations. | |
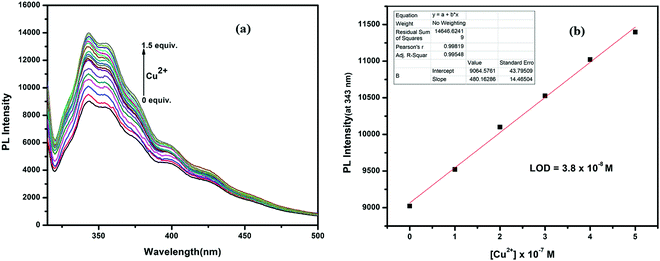 |
| Fig. 10 (a) Fluorescence titration of H2L with Cu2+ and (b) fluorescence detection limit. | |
DFT studies on fluorescence properties
The probe H2L shows a straightforward approach for the selective detection of Cu2+, with enhancement of fluorescence in conjunction with red shift (10 nm) because of chelation-enhanced fluorescence (CHEF) and internal charge transfer (ICT) processes after interaction with Cu2+. The energy gap between the highest occupied molecular orbital (HOMO) and lowest unoccupied molecular orbital (LUMO) of H2L and its Cu(II) complex (1) are 5.55 eV and 2.11 eV, respectively. The complex 1, compared to H2L, established easy electronic transition and obtains the additional stability from 1 (Fig. 11). The contours of the electronic distribution in HOMO and LUMO states of these molecules suggest significant energy difference, 3.44 eV, between H2L and (1). Specifically, both the HOMO and LUMO states of the copper complex 1, in comparison to H2L, reveal that the electrons are more delocalized in the 1 molecule than the H2L, in accordance with the barrier of photo-induced electron transfer process, which may result in the enhancement of fluorescence through CHEF.
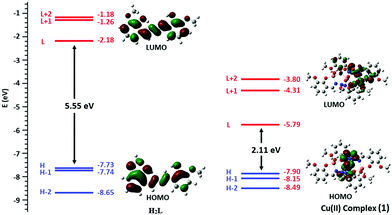 |
| Fig. 11 Energy level diagram for the frontier MOs of H2L (left) and Cu3L2 complex (1) (right). | |
Application of chemosensor H2L in real samples
To explore the Cu2+ sensing ability of the present chemosensor H2L in real water samples, we initially tested using laboratory tap water and distilled water samples. The ion concentration of these samples could not be measured by this method as the laboratory tap water and distilled water samples didn't content Cu2+. We then tested the probe H2L in waste water, and we obtained some results. To check its sensing in different samples, tap water samples artificially contaminated with Cu2+ were prepared by adding known amounts of standard Cu2+ solutions. The prepared samples were analyzed by the same UV-vis spectrometry method, and the recovery amounts were calculated using the calibration curve (intensity vs. conc.) with the help of Beer–Lambert law. As shown in Table 2, the Cu2+ concentrations recovered for each sample are in close agreement with the spiked amounts, with good precision. Thus, the receptor H2L is suitable for detecting Cu2+ ions quantitatively in real water samples, indicating that it could be applied in real environments.
Table 2 Analysis of Cu2+ (μM) in water samples by the developed method (mean ± standard deviation, n = 3)
Sample |
Cu2+ added |
Cu2+ found |
Recovery (%) |
Relative error (%) |
ND = not detected. |
Waste water |
0 |
0.72 ± 0.89 |
— |
— |
|
5 |
5.13 ± 0.97 |
102.6 |
2.6 |
|
10 |
10.15 ± 1.54 |
101.5 |
1.5 |
|
20 |
19.70 ± 0.87 |
98.5 |
1.5 |
Tap water |
0 |
ND |
— |
— |
|
5 |
4.81 ± 1.3 |
96.2 |
3.8 |
|
10 |
10.12 ± 0.93 |
101.2 |
1.2 |
|
20 |
20.06 ± 1.2 |
100.3 |
0.6 |
Distilled water |
0 |
ND |
— |
— |
|
5 |
5.02 ± 1.5 |
100.5 |
0.4 |
|
10 |
9.87 ± 1.1 |
98.7 |
1.3 |
|
20 |
19.96 ± 1.5 |
99.8 |
1.0 |
Comparison of H2L with other fluorescent and colorimetric chemosensors
The performance of the sensing action of the probe H2L towards Cu2+ was compared with some other reported chemosensors, as listed in Table 3. Compared to the other systems, our system has several attractive analytical features, such as high sensitivity, wide linear range, high selectivity, lower detection limit, simple operation technology, good solubility, sensitive visualization and good practical applicability. Moreover, the synthesis of our proposed chemosensor H2L requires only two steps and less hazardous reagents, and no hazardous by-product is formed.
Table 3 Comparison of H2L with other reported chemosensors
Type of sensors |
Ion sensing |
Limit of detection for Cu2+(M) |
Fluorometrically |
References |
Colorimetrically |
Azine based |
Cu2+ |
7.1 × 10−7 |
3.8 × 10−7 (Turn-On) |
Present work |
|
Cu2+, Fe3+ |
9.8 × 10−7 |
— |
59
|
|
Cu2+ |
2.5 × 10−6 |
— |
60
|
Dihydrazone based |
Cu2+ |
5.3 × 10−7 |
— |
48
|
Chromone-based |
Cu2+ |
4.6 × 10−7 |
— |
61
|
Quinoline-based |
Cu2+, CN− |
5.3 × 10−7 |
— |
62
|
|
Cu2+, Fe3+ |
— |
1.35 × 10−7 (Turn-Off) |
63
|
Glycine based |
Cu2+ |
5.0 × 10−7 |
— |
64
|
Triazole based |
Cu2+, Pb2+ |
3.7 × 10−6 |
1.24 × 10−6 (Turn-On) |
46
|
|
Cu2+ |
— |
3.6 × 10−9 (Turn-On) |
65
|
Naphthalene-based |
Cu2+, cysteine |
1.07 × 10−6 |
— |
66
|
Molecular logic gate application
The interesting optical behaviour of the probe H2L was demonstrated by establishing a molecular logic gate. Herein, the logic characteristics of probe H2L can also be viewed as a two-input-one-output ‘‘IMPLICATION’’ and “INHIBIT” logic gate in water media. Taking the idea from different absorption intensities in the UV-Vis spectra of developed probe H2L and its behavior with metal ions, logic operations with Cu2+ as chemical input and absorption spectra as a channel output may be achieved. With addition of Cu2+ to this ligand, a prominent absorbance band is observed at 434 nm, but upon addition of Na2EDTA, the absorbance band at 434 nm vanishes. Hence, the INHIBIT logic gate is achieved. On the other hand, at 313 nm, decreased intensity was observed on addition of Cu2+, which reappears on Na2EDTA addition. Thus, with two inputs, H2L has the ability to exhibit INHIBIT and IMPLICATION functions via absorbance output. The corresponding truth table, along with a sketch of the logic gate, is presented in Fig. 12.
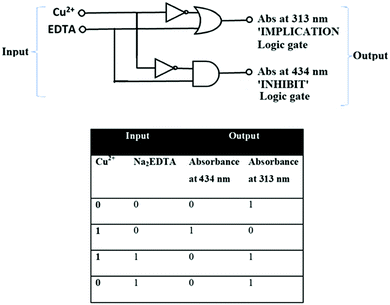 |
| Fig. 12 IMPLICATION and INHIBIT logic gates and truth table. | |
Conclusions
In summary, we have developed a novel azine-based fluorescent colorimetric sensor H2L for the selective and sensitive dual detection of Cu2+. The chemosensor established the presence of the cation Cu2+ by both UV-visible and fluorescence spectra, with an instant change of colour from colourless to intense yellow. LOD values of H2L towards Cu2+ were calculated to be 7.1 × 10−8 M (colorimetrically) and 3.8 × 10−8 M (fluorometrically). The interaction between H2L and Cu2+ were studied by Job's plot, ESI-MS, FT-IR spectroscopy and DFT calculations. Single-crystal structures both for the ligand H2L and its Cu(II) complex 1 were reported. Our developed probe H2L is much more efficient in comparison to other reported ligands. H2L can operate in a wide range of pH and be successfully applied for detection and quantification of Cu2+ in various environmental samples, as well as for constructing INHIBIT and IMPLICATION type molecular logic gates.
Conflicts of interest
Authors declare no conflicts of interest.
Acknowledgements
G. K. P. would like to thank the Department of Science and Technology (SR/FST/CSI-264/2014 and EMR/2017/0001789), Government of India, New Delhi, for financial support. M. S. and A. K. M. gratefully acknowledge CSIR (New Delhi), Government of India, for financial support in the form of the research fellowships.
References
- R. Chandra, A. Ghorai and G. K. Patra, Sens. Actuators, B, 2018, 255, 701 CrossRef CAS
.
- P. Li, X. Duan, Z. Chen, Y. Liu, T. Xie, L. Fang, X. Li, M. Yin and B. Tang, Chem. Commun., 2011, 47, 7755 RSC
.
- N. J. Robinson and D. R. Winge, Biochemistry, 2010, 79, 537 CAS
.
- X. Qi, E. J. Jun, L. Xu, S.-J. Kim, J. S. J. Hong, Y. J. Yoon and J. Yoon, J. Org. Chem., 2006, 71, 2881 CrossRef CAS PubMed
.
- Z. Shi, X. Tang, X. Zhou, J. Cheng, Q. han, J. Zhou, B. Wang, Y. Yang, W. Liu and D. Bai, Inorg. Chem., 2013, 52, 12668 CrossRef CAS PubMed
.
- P. G. Georgopoulos, A. Roy, M. J. Yonone-Lioy, R. E. Opiekun and P. J. Lioy, J. Toxicol. Environ. Health, Part B, 2001, 4, 341 CAS
.
-
H. Sigel and M. Dekker, Metal ions in biological systems in Properties of Copper, New York, USA, 1981.
-
J. A. Cowan, Inorganic Biochemistry: An Introduction, Wiley VCH, New York, NY, USA, 1997 Search PubMed
.
- H. Kozlowski, M. Luczkowski, M. Remelli and D. Valensin, Coord. Chem. Rev., 2012, 256, 2129 CrossRef CAS
.
- J. Xiong, X. Liu, Q. Y. Cheng, S. Xiao, L.-X. Xia, B.-F. Yuan and Y.-Q. Feng, ACS Chem. Biol., 2017, 12, 1636 CrossRef CAS PubMed
.
- Z. Shi, X. Tang, X. Zhou, J. Cheng, Q. Han, J.-A. Zhou, B. Wang, Y. Yang, W. Liu and D. Bai, Inorg. Chem., 2013, 52, 12668 CrossRef CAS PubMed
.
- X. Zhang, Y. Shiraishi and T. Hirai, Org. Lett., 2007, 9, 5039 CrossRef CAS PubMed
.
- E. Gaggelli, H. Kozlowski, D. Valensin and G. Valensin, Chem. Rev., 2006, 106, 1995 CrossRef CAS PubMed
.
- T. V. O’Halloran and V. C. Culotta, J. Biol. Chem., 2000, 275, 25057 CrossRef PubMed
.
- D. Strausak, J. F. B. Mercer, H. H. Dieter, W. Stremmel and G. Multhaup, Brain Res. Bull., 2001, 55, 15 CrossRef
.
- A. C. Rosenzweig and T. V. O’Halloran, Curr. Opin. Chem. Biol., 2000, 4, 140 CrossRef CAS PubMed
.
- A. Singh, Q. Yao, L. Tong, W. C. Still and D. Sames, Tetrahedron Lett., 2000, 41, 9601 CrossRef CAS
.
- G. E. Batley and T. M. Florence, Mar. Chem., 1976, 4, 347 CrossRef CAS
.
- V. K. Gupta, A. K. Jain, G. Maheshwari, H. Lang and Z. Ishtaiwi, Sens. Actuators, B, 2006, 117, 99 CrossRef CAS
.
- X. Chen, M. J. Jou, H. Lee, S. Kou, J. Lim, S. W. Nam, S. Park, K. M. Kim and J. Yoon, Sens. Actuators, B, 2009, 137, 597 CrossRef CAS
.
- S. L. C. Ferreira, M. A. Bezerra, A. S. Santos, W. N. L. dos Santos, C. G. Novaes, O. M. C. de Oliveira, M. L. Oliveira and R. L. Garcia, TrAC, Trends Anal. Chem., 2018, 100, 1 CrossRef CAS
.
- L. Ferńandez-López, B. Gómez-Nieto, M. J. Gismera, M. T. Sevilla and J. R. Procopio, Spectrochim. Acta, Part B, 2018, 147, 21 CrossRef
.
- I. D. I. Calle, P. Pérez-Rodríguez, D. Soto-Gómez and J. E. López-Periago, Microchem. J., 2017, 133, 293 CrossRef
.
- A. P. S. Gonzales, M. A. Firmino, C. S. Nomura, F. R. P. Rocha, P. V. Oliveira and I. Gaubeur, Anal. Chim. Acta, 2009, 636, 198 CrossRef CAS PubMed
.
- T. Sun, Y. Li, Q. Niu, T. Li and Y. Liu, Spectrochim. Acta, Part A, 2018, 195, 142 CrossRef CAS PubMed
.
- L. Tian, J. Qi, K. Qian, O. Oderinde, Q. Liu, C. Yao, W. Song and Y. Wang, J. Electroanal. Chem., 2018, 812, 1 CrossRef CAS
.
- A. Kawde, A. Ismail, A. R. Al-Betar and O. Muraza, Microporous Mesoporous Mater., 2017, 243, 1 CrossRef CAS
.
- W. Zhu, X. Huang, Z. Guo, X. Wu, H. Yu and H. Tian, Chem. Commun., 2012, 48, 1784 RSC
.
- Y. H. Lee, N. Park, Y. B. Park, Y. J. Hwang and J. S. Kim, Chem. Commun., 2014, 50, 3197 RSC
.
- R. Sheng, P. Wang, Y. Gao, Y. Wu, W. Liu, J. Ma, H. Li and S. Wu, Org. Lett., 2008, 10, 5015 CrossRef CAS PubMed
.
- I. Oehme and O. S. Wolfeis, Microchim. Acta, 1997, 126, 177 CrossRef CAS
.
- M. Benounis, N. Jafrezic-Renault, H. Halouani, R. Lamartine and I. Dumazet-Bonnamour, Mater. Sci. Eng., C, 2006, 26, 364–368 CrossRef CAS
.
- R. F. M. Elshaarawy, R. Ali, S. M. Saleh and C. Janiak, J. Mol. Liq., 2017, 241, 308 CrossRef CAS
.
- S. M. Saleh, R. Ali and R. F. Elshaarawy, RSC Adv., 2016, 6, 68709 RSC
.
- G. Sivaraman, M. Iniya, T. Anand, N. G. Kotla, O. Sunnapu, S. Singaravadivel, A. Gulyani and D. Chellappa, Coord. Chem. Rev., 2018, 357, 50 CrossRef CAS
.
- J. Ponniah S, S. K. Barik, A. Thakur, R. Ganesamoorthi and S. Ghosh, Organometallics, 2014, 33, 3096 CrossRef CAS
.
- G. Sivaraman, T. Anand and D. Chellappa, RSC Adv., 2013, 3, 17029 RSC
.
- S. J. Ranee, G. Sivaraman, A. M. Pushpalatha and S. Muthusubramanian, Sens. Actuators, B, 2018, 255, 630 CrossRef CAS
.
- V. K. G. Gangatharan, K. M. Palsamy, S. Gandhi, A. Jamespandi, A. Kandasamy, T. Arunachalam, A. Shenmuganarayanan, S. Balasubramaniyam and R. Jegathalaprathaban, Sens. Actuators, B, 2018, 255, 3235 CrossRef
.
- L. N. Li, S. S. Shen, R. Y. Lin, Y. Bai and H. W. Liu, Chem. Commun., 2017, 53, 9986 RSC
.
- J. Wang, H. Chen, F. Ru, Z. Zhang, X. Mao, D. Shan, J. Chen and X. Lu, Chem. – Eur. J., 2018, 24, 3499 CrossRef CAS PubMed
.
- Y. Ping, Z. Chen, Q. Ding, Q. Zheng, Y. Lin and Y. Peng, Tetrahedron, 2017, 73, 594 CrossRef CAS
.
- F.-U. Rahman, S.-B. Yu, S. K. Khalil, Y. P. Wu, S. Koppireddi, Z.-T. Li, H. Wang and D.-W. Zhang, Sens. Actuators, B, 2018, 263, 594 CrossRef CAS
.
- B. Kaur, N. Kaur and S. Kuma, Coord. Chem. Rev., 2018, 358, 13 CrossRef CAS
.
- A. K. Manna, M. Sahu, K. Rout, U. K. Das and G. K. Patra, Microchem. J., 2020, 157, 104860 CrossRef CAS
.
- K. Rout, A. K. Manna, M. Sahu, J. Mondal, S. K. Singh and G. K. Patra, RSC Adv., 2019, 9, 25919 RSC
.
- M. Sahu, A. K. Manna and G. K. Patra, Inorg. Chim. Acta, 2021, 517, 120199 CrossRef CAS
.
- M. Sahu, A. K. Manna, S. Chowdhury and G. K. Patra, RSC Adv., 2020, 10, 44860 RSC
.
- M. Sahu, A. K. Manna, K. Rout, J. Mondal and G. K. Patra, Inorg. Chim. Acta, 2020, 508, 119633 CrossRef CAS
.
- A. K. Manna, J. Mondal, K. Rout and G. K. Patra, Sens. Actuators, B, 2018, 275, 350 CrossRef CAS
.
- A. K. Manna, K. Rout, S. Chowdhury and G. K. Patra, Photochem. Photobiol. Sci., 2019, 18, 1512 CrossRef CAS PubMed
.
-
SMART & SAINT Software Reference manuals, version 5.0, Bruker AXS Inc., Madison,WI, 1998 Search PubMed
.
- T. Gruene, H. W. Hahn, A. V. Luebben, F. Meilleur and G. M. Sheldrick, J. Appl. Crystallogr., 2014, 47, 462 CrossRef CAS PubMed
.
-
L. J. Farrugia, WinGX: An Integrated System of Windows Programs for the Solution, Refinement and Analysis for Single Crystal X-ray Diffraction Data, version 1.80.01, Department of Chemistry: University of Glasgow, 2003 Search PubMed
.
- D. R. Gayakwad, S. R. Sarda, S. U. Tekale, R. B. Nawale, D. Rajani, J. B. Bharad and R. P. Pawar, Int. J. Med. Med. Sci., 2020, 11, 14 Search PubMed
.
-
M. J. Frisch, G. W. Trucks, H. B. Schlegel, G. E. Scuseria, M. A. Robb, J. R. Cheeseman, G. Scalmani, V. Barone, B. Mennucci, G. A. Petersson, H. Nakatsuji, M. Caricato, X. Li, H. P. Hratchian, A. F. Izmaylov, J. Bloino, G. Zheng, J. L. Sonnenberg, M. Hada, M. Ehara, K. Toyota, R. Fukuda, J. Hasegawa, M. Ishida, T. Nakajima, Y. Honda, O. Kitao, H. Nakai, T. Vreven, J. A. Montgomery, Jr., J. E. Peralta, F. Ogliaro, M. Bearpark, J. J. Heyd, E. Brothers, K. N. Kudin, V. N. Staroverov, R. Kobayashi, J. Normand, K. Raghavachari, A. Rendell, J. C. Burant, S. S. Iyengar, J. Tomasi, M. Cossi, N. Rega, J. M. Millam, M. Klene, J. E. Knox, J. B. Cross, V. Bakken, C. Adamo, J. Jaramillo, R. Gomperts, R. E. Stratmann, O. Yazyev, A. J. Austin, R. Cammi, C. Pomelli, J. W. Ochterski, R. L. Martin, K. Morokuma, V. G. Zakrzewski, G. A. Voth, P. Salvador, J. J. Dannenberg, S. Dapprich, A. D. Daniels, Ö. Farkas, J. B. Foresman, J. V. Ortiz, J. Cioslowski and D. J. Fox, Gaussian 09, Revision C.01, Gaussian Inc., Wallingford, CT, 2009 Search PubMed
.
- A. D. Becke, J. Chem. Phys., 1993, 98, 5648 CrossRef CAS
.
- A. W. Addison, T. N. Rao, J. Reedijk, J. van Rijn and G. C. Verschoor, J. Chem. Soc., Dalton Trans., 1984, 1349 RSC
.
- N. Narayanaswamy and T. Govindaraju, Sens. Actuators, B, 2012, 161, 304 CrossRef CAS
.
- G. K. Patra, R. Chandra, A. Ghorai and K. K. Shrivas, Inorg. Chim. Acta, 2017, 462, 315 CrossRef CAS
.
- A. Mohammadi, B. Khalili and A. S. Haghayegh, Spectrochim. Acta, Part A, 2019, 222, 117193 CrossRef CAS PubMed
.
- C. Wu, J. Wang, J. Shen, C. Zhang, Z. Wu and H. Zhou, Tetrahedron, 2017, 73, 5715 CrossRef CAS
.
- B. Zhang, H. Liu, F. Wu, G. Hao, Y. Chen, C. Tan, Y. Tan and Y. Jiang, Sens. Actuators, B, 2017, 243, 765 CrossRef CAS
.
- N. H. Ly, C. Seo and S.-W. Joo, Sensors, 2016, 16, 1785 CrossRef PubMed
.
- S. Qiu, Y. Wei, T. Tu, J. Xiang, D. Zhang, Q. Chen, L. Luo and Z. Lin, Food Chem., 2020, 317, 126434 CrossRef CAS PubMed
.
- S. A. Lee, J. J. Lee, J. W. Shin, K. S. Min and C. Kim, Dyes Pigm., 2015, 116, 131 CrossRef CAS
.
Footnote |
† Electronic supplementary information (ESI) available: Fig. S1–S10. CCDC 2087278 and 2087279. For ESI and crystallographic data in CIF or other electronic format see DOI: 10.1039/d1ma01227d |
|
This journal is © The Royal Society of Chemistry 2022 |