DOI:
10.1039/D1MA01055G
(Paper)
Mater. Adv., 2022,
3, 2447-2455
Air-grown hybrid copper(I) halide single crystals: structural transformations and ultraviolet-pumped photoluminescence applications†
Received
11th November 2021
, Accepted 24th January 2022
First published on 25th January 2022
Abstract
Lead-free copper-based halide hybrid materials have emerged as a class of potential candidates for next-generation optoelectronic devices. In this work, we demonstrated that Gua4Cu4Br8 and Gua3Cu2I5 (Gua = CH6N3+) single crystals have been successfully grown by slow evaporation methods in an ambient atmosphere. Interestingly, non-centrosymmetric Gua6Cu4I10 single crystals were formed on the basis of Gua3Cu2I5 induced by chiral (R)- and (S)-methylbenzylamine (MBA) cations. In particular, Gua3Cu2I5 exhibited a large Stokes shift of about 200 nm with a long PL lifetime of about 3835 ns and an absolute PLQY of about 72.04% at room temperature. More importantly, ultraviolet (UV)-pumped light-emitting diodes based on Gua3Cu2I5 single crystals exhibited strong photoluminescence characteristics at an emission peaking position of about 575 nm with high luminance. The above characteristics should provide guidance for further applications of lead-free hybrid single-crystalline optoelectronic devices.
Introduction
Hybrid lead-free copper halide materials have attracted attention in optoelectronic devices such as solar cells,1–3 field-effect transistors (FETs),4,5 light emitting diodes (LEDs),6,7 resistive switching memory devices,8,9 circularly polarized luminescence (CPL) based devices,10 photodetectors,11,12etc. Most of the perovskite studies mainly focused on Pb-based halide hybrids, which suffered from the important issues of stability13,14 and intrinsic toxicity.15,16 Therefore, the above studies urged materials scientists to investigate new lead-free halide hybrids with excellent optoelectronic properties and stability. To search for environmentally friendly lead-free halide hybrids with outstanding optoelectronic properties, there is an effective strategy to replace Pb with low-toxic tin (Sn),17–19 manganese (Mn),20,21 and copper (Cu)22–25 in lead halide perovskites, which can not only eliminate the toxicity of Pb but also obtain excellent physical characteristics such as high mobility, strong photoluminescence and good stability. Sn-based halide perovskite materials exhibited excellent semiconducting properties, and were widely used in FETs.26 Mn-based halide perovskites possessed good photoluminescence characteristics, which were applied to light-emitting and scintillating devices.27,28 Moreover, nontoxic transition metal Cu is an ideal candidate for Pb to form Cu-based halide hybrid compounds. Previous studies on Cu-based halide perovskite materials focused on divalent Cu(II) compounds, most of which are powders and thin films.29,30 The structural and magnetic properties of (4,4′-bipyridinium)Cu2Cl6−xBrx are investigated.31 Reversible and irreversible thermochromism behaviors of copper(II)-based halide perovskites were demonstrated.32 The thermochromic behaviors of two perovskites (2-chloroethylammonium)2CuCl4 and (2-bromoethylammonium)2CuCl4 were discussed.32 The above studies mainly focused on divalent Cu(II)-based halide perovskites exhibiting poor photoluminescence characteristics, which hinders their application in the optoelectronic field. Recently, Cu(I)-based halide compounds with excellent photoluminescence properties urged us to investigate these issues of Cu(I)-based halide analogues. The all-inorganic Cu(I)-based halide perovskite Cs3Cu2I5 with a bright blue emission and a high photoluminescence quantum yield (PLQY) of ∼91.2% was first reported by Jun et al.33 Then, high-performance and stable yellow LEDs based on solution-processed CsCu2I3 thin films were fabricated.34 Stable UV-pumped white light-emitting diodes based on anthracene-coated CsCu2I3 were demonstrated by Liu et al.6 All-inorganic blue emitters K2CuX3 (X = Cl, Br) and Cs3Cu2Br5−xIx (0 ≤ x ≤ 5) with near-unity PLQY were investigated by the Saparov research group.35,36 Nontoxic and ultra-stable LEDs based on 1D-Cu4I6(bttmp)2 thin films were prepared by Zhu et al., which exhibited a high PLQY and long-term air stability.7 Recently, highly efficient cool-white photoluminescence characteristics of Gua3Cu2I5 (Gua = guanidine) single crystals in DMF in an inert atmosphere were investigated by Peng et al.37 Opaque Gua3Cu2I5 single crystals exhibit highly efficient cool-white emission peaking at 481 nm. At higher temperatures, Gua3Cu2I5 single crystals exhibited yellow emission, while at room temperature, they exhibited blue emission. Therefore, it is necessary to introduce different organic molecules into the lattice of Gua3Cu2I5 and form new photoluminescent materials by solution synthesis and growth methods in an ambient atmosphere.
In this work, bulk colourless Gua4Cu4Br8 and Gua3Cu2I5 (Gua = CH6N3+) single crystals were successfully grown by a slow evaporation method in an ambient atmosphere. Interestingly, non-centrosymmetric Gua6Cu4I10 single crystals were formed on the basis of Gua3Cu2I5 induced by chiral (R)- and (S)-methylbenzylamine (MBA) cations. The band gap, theoretical calculations, X-ray photoelectron spectra (XPS), thermal stability and optical properties of Gua4Cu4Br8 and Gua3Cu2I5 were studied, which exhibited a large Stokes shift with a long PL decay lifetime. Both Gua4Cu4Br8 and Gua3Cu2I5 are direct band gap semiconductor materials. As we all know, Gua3Cu2I5 crystals exhibited good luminescence properties.37 From the viewpoint of crystal structures, Gua4Cu4Br8 single crystals were introduced to analyse the crystal structure and PL differences induced by the Br and I atoms. Similarly, we tried to compare Gua3Cu2I5 and Gua6Cu4I10 induced by chiral MBA cations. The PL decay lifetime and PLQY exhibited obvious difference due to the different crystal structures. Based on these results, it is concluded that Gua3Cu2I5 exhibited the strongest luminescence properties among the three single crystals. Therefore, ultraviolet (UV)-pumped yellow light-emitting diodes based on Gua3Cu2I5 single crystals were fabricated, which exhibited strong photoluminescence characteristics at an emission peaking position of about 575 nm with a luminance of about 3600 cd m−2. The above behaviours should pave the way for further optoelectronic applications of these single-crystalline materials.
Results and discussion
A previous report37 has shown that opaque single crystals of blue emissive Gua3Cu2I5 were formed in organic solvents in an inert atmosphere. Due to the ease of oxidation of copper(I), it is challenging to obtain copper(I) halide perovskite single crystals in an ambient atmosphere. According to eqn (1)–(3) of redox potentials, H3PO2 was used as a reducing reagent to prevent the oxidation of I− and Cu+. If the amount of H3PO2 is too little, the oxidation of Cu+ and I− can easily occur. In contrast, an excessive amount of H3PO2 is too viscous to be beneficial for crystal growth. Therefore, all the growth conditions were maintained in an ambient atmosphere. Bulk colourless Gua4Cu4Br8 and Gua3Cu2I5 single crystals have been grown at room temperature by slow evaporation methods for one week (Fig. 1). When exposed to UV-254 nm and 365 nm irradiation conditions, bulk colourless Gua4Cu4Br8 and Gua3Cu2I5 single crystals exhibited different photoluminescence characteristics as shown in Fig. 1(B, C) and (E, F). In particular, Gua3Cu2I5 single crystals exhibited strong yellow emission under irradiation of UV-254 nm wavelength as shown in Fig. 1E. In the Gua–Cu–Br formation, Gua2CO3 reacted with CuBr in a molar ratio of 1
:
2 in HBr and H3PO2 mixed solution at 60 °C in an ambient atmosphere. In the formation processes of Gua–Cu–I systems, Gua2CO3 reacted with CuI in a molar ratio of 3
:
4 in HI and H3PO2 mixed solution at 70 °C in an ambient atmosphere. Previously, Tang's research group reported chiral tetranuclear copper(I) iodide clusters based on the (R)- and (S)-MBA.38 Cu+ is easily connected with (R)- and (S)-MBA so as to form a tetrahedral coordination environment. But in HI and H3PO2 mixed solution, the Cu-(R)/(S)-MBA clusters were completely replaced by I−. Interestingly, Gua3Cu2I5 reacted with chiral R-or S-MBA in a molar ratio of 2
:
1 in HI and H3PO2 mixed solution to promote the formation of non-centrosymmetric Gua6Cu4I10 single crystals as shown in Fig. S1 (ESI†). | H3PO3(aq) + 2H+ +2e− = H3PO2(aq) + H2O E = −0.499 eV | (1) |
| Cu2+ + e− = Cu+E = +0.159 eV | (2) |
| I2 + 2e− = 2I−E = +0.54 eV | (3) |
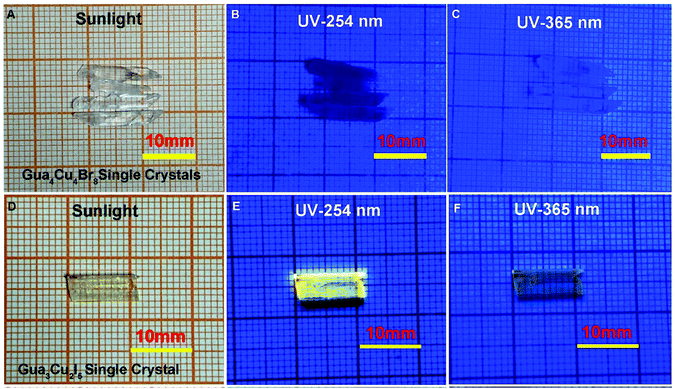 |
| Fig. 1 Photographs of Gua4Cu4Br8 single crystals under sunlight (A), UV-254 nm (B) and UV-365 nm (C) conditions. Photographs of Gua3Cu2I5 single crystals under sunlight (D), UV-254 nm (E) and UV-365 nm (F) conditions. | |
Differently, a previous report revealed that Gua3Cu2I5 exhibited an orthorhombic system with the space group Fdd2 (no. 43).37 Gua4Cu4Br8 and Gua3Cu2I5 single crystals crystallized in monoclinic systems of P21/n (no. 14) and C2/c (no. 15) space groups by single-crystal X-ray diffraction measurements at room temperature for the first time. All the crystallographic data are listed in Table S1 (ESI†). In the crystal structure of Gua4Cu4Br8, the adjacent four Cu atoms were coordinated with ten bromine atoms (Fig. 2A), forming a special {Cu4Br10} face-sharing tetrahedral unit. In addition, the {Cu4Br10} polyhedra are connected from the same layer and surrounded by Gua cations. Moreover, although the atomic radius of I is larger than that of Br, the adjacent four Cu atoms are coordinated with seven iodine atoms forming a polyhedral unit as shown in Fig. 2C. This structure is connected from the same layer and surrounded by Gua cations to form the layered structures, which is different from the isolated {Cu2I5} face-sharing tetrahedral dimer.37 The powder X-ray diffraction (PXRD) patterns of all the materials are in good agreement with the simulated XRD diffraction patterns of these single crystals, as shown in Fig. 2(B, D) and Fig. S1C (ESI†). Next, X-ray photoelectron spectroscopy (XPS) measurements were performed to investigate the valence state of elements in Gua4Cu4Br8 and Gua3Cu2I5 single crystals. All the XPS results are demarcated using C 1s (284.8 eV) as the reference. These results confirm the existence of Br, I and Cu elements. The high-resolution core-level XPS spectra of C 1s, N 1s, Cu 2p, Br 3d and I 3d are extracted, as shown in Fig. S2 (ESI†). Cu 2p XPS spectra are the prominent characteristics for verifying the Cu oxidation state, which means that the Cu(I) state plays a dominant role in Gua4Cu4Br8 and Gua3Cu2I5 single crystals. The HRXPS spectrum peak at a binding energy of about 933 eV can hardly be observed,39 which further eliminates the existence of the Cu(II) state. The binding energies are located at about 932 and 952 eV, corresponding to Cu 2p3/2 and Cu 2p1/2, respectively. Moreover, no shake-up satellite appears between the Cu 2p3/2 and Cu 2p1/2 peaks, indicating that only Cu(I) ions exist in the lattice,40–42 which confirms that all the samples are both pure phase copper(I) based halide hybrids. From the above description, the presence of I−, Br−, CH6N3+ and Cu+ verifies the chemical composition and valence state of Gua4Cu4Br8 and Gua3Cu2I5 single crystals.
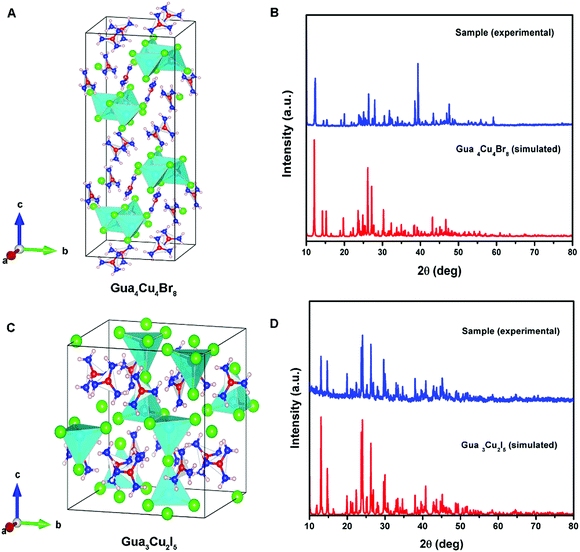 |
| Fig. 2 (A) Crystal structure of Gua4Cu4Br8. (B) Powder and single-crystal X-ray diffraction (PXRD) patterns of Gua4Cu4Br8. (C) Crystal structure of Gua3Cu2I5. (D) PXRD patterns of Gua3Cu2I5. | |
The structural transformation usually involves bond rearrangements and composition changes of atoms in a crystal caused by the changes in the coordination number of metals, the addition of different ligands, temperature changes or solvent exchanges.43–45 Interestingly, single crystal-to-single crystal phase transformations from Gua3Cu2I5 to Gua6Cu4I10 occurred induced by the effect on the chiral R-or S-MBA. Gua6Cu4I10 single crystals under sunlight and UV-254 nm wavelength conditions are shown in Fig. S1A and B (ESI†). Gua6Cu4I10 single crystals exhibited an orthorhombic system with Cmc21 (no. 36) with the lattice parameters of a = 12.072(3) Å, b = 13.215(3) Å, and c = 13.581(3) Å. Gua3Cu2I5 exhibited the lattice parameters of a = 12.0317(9) Å, b = 13.2491(10) Å, and c = 13.5695(10) Å; β = 90.985(7)° in Table S1 (ESI†). The lattice parameters of Gua6Cu4I10 are larger than those of Gua3Cu2I5. In the crystal structure of Gua6Cu4I10, the strong distortions of the {Cu3I6} polyhedral unit can be caused by the Gua parts, which results in an acentric structure as shown in Fig. 3. As stated above, Gua6Cu4I10 single crystals were easily formed and crystallized when Gua3Cu2I5 single crystals were exposed to R- and S-MBA cations.
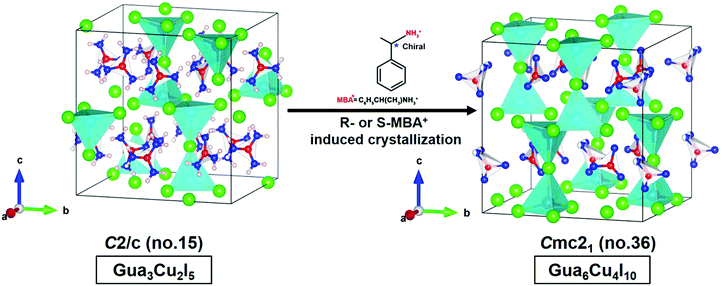 |
| Fig. 3 Crystal structural transformations from Gua3Cu2I5 to Gua6Cu4I10 induced by chiral MBA cations. | |
Subsequently, the optical band gap of Gua4Cu4Br8 and Gua3Cu2I5 was investigated. The optical band gap is determined using the Kubelka–Munk function.46 A previous study37 has demonstrated the optical band gap of blue emissive Gua3Cu2I5 powders in an air atmosphere. A distinct feature peak at 366 nm can be observed, which corresponds to the calculated band gap of 2.98 eV. In our results, the optical band gaps of Gua4Cu4Br8 and Gua3Cu2I5 in Fig. 4A and B were 3.6 eV and 2.72 eV, respectively. The electronic properties of Gua4Cu4Br8 and Gua3Cu2I5 were determined by the density functional theory (DFT) calculation method, as shown in Fig. 4C and D, both of which exhibited the direct band gap semiconductors, according to the calculation method based on a previous report.47 PBE functional calculations48 generally underestimate the band gap. Differently, blue emissive Cua3Cu2I5 exhibited a direct band gap of 2.75 eV at the G point.37 However, from Fig. 4D, yellow emissive Cua3Cu2I5 showed a band gap of 2.44 eV at the Γ point. The detailed projected density of states (PDOS) in Fig. 4E and F indicates that the valence band maximum (VBM) of yellow emissive Cua3Cu2I5 is composed of inorganic parts, Cu 3d and I 5p atomic orbitals. However, for the conduction band minimum (CBM), the contribution of specific atomic orbitals cannot be found obviously. To study the composition of the CBM, we calculated more states of PDOS as shown in Fig. 4F. Although the 4S orbital of Cu accounts for the highest proportion, its height is very low in comparison with the height of total DOS. Other orbitals of I 5s, I 5p, and partly Gua molecules make second contribution with a similar proportion. To further explain the PDOS diagrams of the VBM and CBM, the spatial distribution of charge density is plotted. It can be seen from Fig. S3A (ESI†) that the VBM is mainly composed of Cu3d and I5p atomic orbitals. In Fig. S3B (ESI†), the distribution of the CBM is more interesting. The charge density distribution is wide. Both C and N atoms on Gua molecules show little charge density distribution. The main distribution is concentrated on the Cu atoms sharing two I atoms. The distance between the two Cu atoms is relatively close, about 2.55 Å. The 4s orbitals of Cu atoms overlap partially, which is similar to the distribution of π orbitals. Moreover, it should be noted that the 5s and 5p orbitals of I atoms are also partially involved in the CBM. This also explains the distribution of the CBM. The CBM is no longer composed of pure Cu 4s orbitals. The main compositions for the CBM are the overlapping 4s orbitals of Cu atoms that share two I atoms.
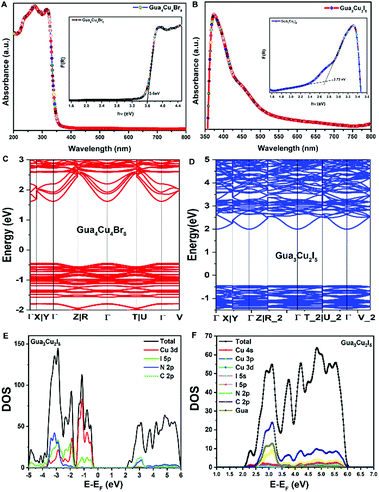 |
| Fig. 4 Optical band gap of Gua4Cu4Br8 (A) and Gua3Cu2I5 (B). DFT band structures of Gua4Cu4Br8 (C) and Gua3Cu2I5 (D). Projected density of states (PDOS) plots of the Gua3Cu2I5via DFT calculations (E). Detailed projected density of states (PDOS) plots of the Gua3Cu2I5via DFT calculations (F). | |
Previously, the emissions of Gua3Cu2I5 single crystals exhibited a reversible phase transition from blue to yellow color in a heating–cooling cycle.37 The thermogravimetry analysis (TGA) measurements of Gua4Cu4Br8 and Gua3Cu2I5 were performed from room temperature to 600 °C as shown in Fig. S4 (ESI†). It can be seen that Gua4Cu4Br8 and Gua3Cu2I5 are stable up to about 300 °C. In particular, the emission colour changes of Gua3Cu2I5 single crystals when exposed to heating and cooling processes under the irradiation of UV-254 nm wavelength cannot be observed in Fig. S5 (ESI†). They both showed relatively good thermal stability. Furthermore, the PL spectra and the PL decay lifetime measurements of Gua4Cu4Br8 and Gua3Cu2I5 powders and single crystals were performed as shown in Fig. 5 Gua4Cu4Br8 and Gua3Cu2I5 powders and single crystals exhibited broad PL peaks, which was similar to the previous reports on perovskite materials.49 From Fig. 5A and B, the excitonic absorption bands of Gua4Cu4Br8 and Gua3Cu2I5 powders and single crystals were positioned at 330 nm and 325 nm, respectively. The PL excitation (PLE) and PL spectra of Gua4Cu4Br8 and Gua3Cu2I5 powders and single crystals also exhibited only one PL peak at about 600 nm and 575 nm, respectively. However, the excitonic absorption band of Gua6Cu4I10 single crystals exhibited was positioned at 340 nm. The PL spectra of Gua6Cu4I10 single crystals also exhibited only one PL peak at about 560 nm in Fig. S1D (ESI†). Such broad luminescence and a large stoke shift were attributed to self-trapped excitons.50–52 The time-resolved photoluminescence (TRPL) spectra of Gua4Cu4Br8 and Gua3Cu2I5 powders and single crystals were well fitted by the first-order exponential decay function, as shown in Fig. 5B, C, E and F. The PL decay lifetime (τ) of Gua4Cu4Br8 powders and single crystals was 538 ns and 505 ns with an absolute photoluminescence quantum yield (PLQY) of less than 1% when exposed to excitation at 290 nm and emission at 600 nm. However, Gua3Cu2I5 powders and single crystals exhibit yellow luminescence with PL decay lifetimes of 3835 ns (3.835 μs) and 3830 ns (3.830 μs) under the conditions of excitation at 290 nm and emission at 550 nm. And the absolute PLQY of Gua3Cu2I5 is about 72.04% as shown in Fig. S6 (ESI†). However, the TRPL spectrum of Gua6Cu4I10 powders agreed well with the biexponential decay function. The average PL decay lifetime (τ) of Gua6Cu4I10 powders was 6.29 μs with an absolute PLQY of 12.07% when exposed to excitation at 330 nm and emission at 550 nm in Fig. S7 and S8 (ESI†).
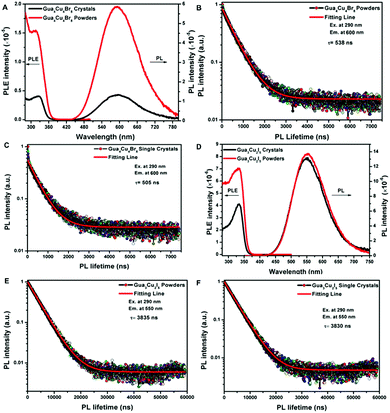 |
| Fig. 5 PLE and PL spectra of Gua4Cu4Br8 (A). PL decay lifetime of Gua4Cu4Br8 powders (B) and single crystals (C). PLE and PL spectra of Gua3Cu2I5 (D). PL decay lifetime of Gua3Cu2I5 powders (E) and single crystals (F). | |
As descripted above, a high PL intensity and yellow emission of Gua3Cu2I5 perovskite materials urged us to employ the obtained perovskite single crystals for LED applications. There has been a previous report on the utilization of Gua3Cu2I5 perovskite powders for UV-pumped LEDs exhibiting bright cool-white emissions.37 Differently, we introduced Gua3Cu2I5 single crystals into UV-pumped LEDs. The commercial UV-LED (255 nm) chip was used to optically pump different phosphor mixtures. Gua3Cu2I5 single crystals were assembled with a 255 nm UV-LED chip to form LEDs, as illustrated in Fig. 6(A, B) and Movie S1 (ESI†). The broad emission spectra of UV-pumped LEDs based on Gua3Cu2I5 single crystals are shown in Fig. 6B. The PL intensity of the LEDs based on Gua3Cu2I5 single crystals increases with an increase in the working voltage from 5.5 to 7.5 V, indicating the high photoluminescence characteristics. The PL spectra presented an emission peak of UV-pumped LEDs at around 575 nm, which correspond to the emissions of Gua3Cu2I5 single crystals. From Fig. 6C, the measurement results at room temperature demonstrated that the CIE color coordinate is (0.44, 0.51), which agreed well with the yellow emission region. With the increase of working voltage from 5.5 to 7.5 V, the luminance of UV-pumped LEDs also increased and reached up to about 3600 cd m2 as shown in Fig. 6D. These results show that the yellow light emitted from the LEDs based on Gua3Cu2I5 single crystals exhibited high luminance, which can further promote the applications of high-brightness LEDs and other optoelectronic devices. Moreover, the operational stability measurements of Gua3Cu2I5 single crystal-based LEDs were performed under UV light (255 nm) excitation for 35 minutes in air, as shown in Fig. 7. And the PL intensity was recorded every 5 minutes. The PL intensity gradually decreased with the increase of time. The possible reason is that the oxidation and decompositions of Gua3Cu2I5 single crystals happen when exposed to light and air.53 If encapsulations of these devices are performed, Gua3Cu2I5 single crystal-based LEDs will exhibit relatively good photoluminescence.
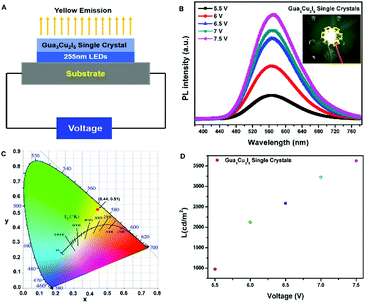 |
| Fig. 6 (A) Schematic illustration of fabricated UV-pumped LEDs based on Gua3Cu2I5 single crystals. (B) PL intensity of Gua3Cu2I5 single-crystalline UV-pumped LED devices under the excitation of UV-255 nm LED chips at different working voltages. Inset: The photograph of Gua3Cu2I5 single-crystalline UV-pumped LEDs at a work voltage of 7.5 V. (C) CIE chromaticity diagram of Gua3Cu2I5 at room temperature. (D) Luminance–voltage diagram of Gua3Cu2I5 single crystal-based UV-pumped LEDs. | |
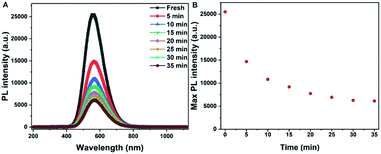 |
| Fig. 7 (A) PL intensity and (B) the maximum intensity of Gua3Cu2I5 single-crystalline UV-pumped LED devices under the excitation of UV-255 nm LED chips after different running times. | |
Conclusions
In summary, we have demonstrated that Gua4Cu4Br8 and Gua3Cu2I5 single crystals with photoluminescence characteristics were grown by slow evaporation methods in air. Acentric Gua6Cu4I10 single crystals originated from Gua3Cu2I5 single crystals induced by (R)- and (S)-MBA cations. The obtained copper(I) halide perovskites exhibited strong PL emissions and large Stoke shifts (about 200 nm) between their absorption and emission spectra. Color coordinates of (0.44, 0.51) and a high luminance of up to 3600 cd m−2 were obtained based on Gua3Cu2I5 single-crystalline UV pumped LEDs, which can provide broad prospects for potential applications in solid-state lighting and displays.
Experimental
Materials
CuI (AR, J&K), CuBr (AR, J&K), Gua2CO3 (AR, J&K), H3PO2 (AR, J&K), HI (57%, J&K), HBr (48%, J&K), R-MBA, and S-MBA were purchased from Sino-pharm and utilized without further purification.
Synthesis and crystal growth
Gua4Cu4Br8 was synthesized with a reaction of Gua2CO3 and CuBr with a molar ratio of 1
:
2 in mixed acid (HBr/H3PO2 = 3
:
1) solution. Gua2CO3 (0.3650 g, 2 mmol) and CuBr (0.5710 g, 4 mmol) were dissolved in HBr (6 mL) and H3PO2 (2 mL) solution at 60 °C temperature to form a colourless solution. And then the solution was cooled to room temperature. Bulk Gua4Cu4Br8 single crystals were formed by a slow evaporation method in an ambient atmosphere as shown in Fig. 1A.
Gua3Cu2I5 was synthesized with a reaction of Gua2CO3 and CuI with a molar ratio of 3
:
4 in mixed acid (HI/H3PO2 = 2
:
1) solution. Gua2CO3 (1.0910 g, 6 mmol) and CuI (1.5250 g, 8 mmol) were dissolved in HI (8 mL) and H3PO2 (4 mL) solution at 70 °C temperature to form a light-yellow solution. And then the solution was cooled to room temperature. Bulk Gua3Cu2I5 single crystals were formed by a slow evaporation method in an ambient atmosphere as shown in Fig. 1D.
Gua6Cu4I10 was synthesized with a reaction of Gua3Cu2I5 and R/S-MBA with a molar ratio of 2
:
1 in mixed acid (HI/H3PO2 = 2
:
1) solution. Gua3Cu2I5 (3.7560 g, 4 mmol) and R/S-MBA (0.2435 g, 2 mmol) were dissolved in HI (12 mL) and H3PO2 (6 mL) solution at 70 °C temperature to form a light-yellow solution. And then the solution was cooled to room temperature. Bulk Gua6Cu4I10 single crystals were formed by a slow evaporation method in an ambient atmosphere as shown in Fig. S3 (ESI†).
Slow evaporation was carried out under the following conditions: oil bath (35 °C), humidity (20%) and atmospheric pressure.
Powder and single crystal X-ray diffraction measurements
X-Ray powder diffraction patterns were determined on a PANalytical XPert Pro MPD equipment with Cu-Ka radiation in the 2θ range of 100°–90° using reasonable step size and step time settings. Single-crystal X-ray diffraction data were collected on a Bruker D8 advance diffractometer equipped with a CCD detector (graphite-monochromated Mo-Ka radiation, λ = 0.71073 Å) at 293(2) K. APEX3 software was used for data integration and cell refinements. The crystal was kept at 293(2) K during data collection. Using Olex2,54 the structure was solved with the Olex2.solve55 structure solution program using Charge Flipping and refined with the XL56 refinement package using Least Squares minimization.
X-Ray photoelectron spectroscopy (XPS) measurements
Polycrystalline samples were extracted using a sample transporter that protects against air exposure. The sample transporter is connected to an ultra-high vacuum chamber and then transferred to the XPS systems for characterization. Pass energy values are 160 eV for XPS wide scan, and 10 eV for high resolution scan. All the data analysis about XPS was performed using the XPS Peaks Fit software. All the elements were fitted with the 80% Gaussian and 20% Lorentzian peak shapes after applying background subtractions with Shirley function.57
UV-Vis and steady-state, time-resolved photoluminescence (PL) spectrum measurements
The optical absorption spectra were recorded using a UV-Vis absorption spectrophotometer (PerkinElmer, Lambda 1050). Steady-state, time-resolved photoluminescence (PL) spectra were recorded with a photoluminescence system (HORIBA, FluoroLog-3). The powder and single crystalline samples were placed in a holder applied to the photoluminescence system. The absolute PLQY of all the samples was measured, which was coated on the BaSO4 standard sample.
Simulation details
Structural optimization was performed via the Vienna ab initio simulation package (VASP)58,59 with the conjugate gradient method. The Perdew, Burke, and Ernzerhof exchange–correlation functional within GGA was used.60 The convergence of energy between two electronic steps in the self-consistent process was set to 10–5 eV. Ionic relaxation was stopped when the maximum force was smaller than 0.01 eV Å−1. All atoms in the models and the cell shape and volume were fully relaxed. A Monkhorst–Pack grid of 3 × 2 × 2 is used for the reciprocal space sampling.61 An energy cut-off of 450 eV was adopted for plane-wave expansion of electronic wave functions.
Thermogravimetric analysis measurements
Thermogravimetric analysis (TGA) was carried out using a TGA/DSC1/1600HT analyzer (METTLER TOLEDO Instruments). All the powder samples were placed in an Al2O3 crucible, and heated at a rate of 10 °C min−1 from room temperature to 600 °C under flowing N2 gas, respectively.
UV-Pumped LEDs fabrication and measurements
The PL, CIE chromaticity and luminance characteristics of UV-pumped LEDs were investigated using a PR-788 spectrophotometer (Photo Research) and a Keithley-2400 instrument. For fabricating UV-pumped LEDs, regular Gua3Cu2I5 single crystals were placed on commercial UV LED chips with 255 nm emission wavelength as the pumping source for LEDs as shown in Fig. 6(A, B) and Movie S1 (ESI†). The fabricated LED devices were placed in the measurement equipment.
Author contributions
Y. D. designed the experiment; Y. D. and Z. S. performed the crystal growth and the crystallographic experiments. L. M. and B. Y. performed and analysed the property characterization including steady-state PL, TRPL, UV-vis diffuse reflectance spectroscopy and UV-pumped LED fabrications. G. L. contributed to theoretical calculations. Y. D. and Z. S. wrote the paper. L. M. and G. L. revised the paper and provided valuable suggestions. All authors revised and approved the final version of the manuscript for submission.
Conflicts of interest
There are no conflicts to declare.
Acknowledgements
This work is supported by the National Natural Science Foundation of China (Grant No. 51802215). All the authors thank Dr Jing Wei, Beijing Institute of Technology, and Dr Chunlong Li, Qilu University of Technology for their help in XPS and TGA measurements.
Notes and references
- Z. Shi, J. Guo, Y. Chen, Q. Li, Y. Pan, H. Zhang, Y. Xia and W. Huang, Adv. Mater., 2017, 29, 1605005 CrossRef PubMed.
- D. Cortecchia, H. Dewi, A. Yin, J. Bruno, A. Chen, S. Baikie, T. Boix, P. P. Grätzel, M. Mhaisalkar, S. Soci and C. N. Mathews, Inorg. Chem., 2016, 55, 1044 CrossRef CAS PubMed.
- X. Li, B. Li, J. Chang, B. Ding, S. Zheng, Y. Wu, J. Yang, G. Yang, X. Zhong and J. Wang, ACS Appl. Energy Mater., 2018, 1, 2709 CrossRef CAS.
- C.-H. Choi, J. Y. Gorecki, Z. Fang, M. Allen, S. Li, L.-Y. Lin, C.-C. Cheng and C.-H. Chang, J. Mater. Chem. C, 2016, 4, 10309 RSC.
- A. Liu, H. Zhu, W. T. Park, S. J. Kang, Y. Xu, M.-G. Kim and Y.-Y. Noh, Adv. Mater., 2018, 30, 1802379 CrossRef PubMed.
- W. Liu, K. W. Ng, H. Lin, Z. Dai, J. Xu, S. Su, Z. Tang and S. Wang, J. Phys. Chem. C, 2021, 125, 13076 CrossRef CAS.
- K. Zhu, Z. Cheng, S. Rangan, M. Cotlet, J. Du, L. Kasaei, S. J. Teat, W. Liu, Y. Chen, L. C. Feldman, D. M. O’Carroll and J. Li, ACS Energy Lett., 2021, 6, 2565 CrossRef CAS.
- S.-Y. Kim, J.-M. Yang, E.-S. Choi and N.-G. Park, Adv. Funct. Mater., 2020, 30, 2002653 CrossRef CAS.
- F. Zeng, Y. Guo, W. Hu, Y. Tan, X. Zhang, J. Feng and X. Tang, ACS Appl. Mater. Interfaces, 2020, 12, 23094 CrossRef CAS PubMed.
- Y. Dang, X. Liu, B. Cao and X. Tao, Matter, 2021, 4, 794 CrossRef CAS.
- Y. Li, Z. Shi, L. Wang, Y. Chen, W. Liang, D. Wu, X. Li, Y. Zhang, C. Shan and X. Fan, Mater. Horiz., 2020, 7, 1613 RSC.
- J. Ma, X. Xia, S. Yan, Y. Li, W. Liang, J. Yan, X. Chen, D. Wu, X. Li and Z. Shi, ACS Appl. Mater. Interfaces, 2021, 13, 15409 CrossRef CAS PubMed.
- W. Xiang, S. F. Liu and W. Tress, Energy Environ. Sci., 2021, 14, 2090 RSC.
- B. Ehrler and E. M. Hutter, Matter, 2020, 2, 794 CrossRef.
- A. Babayigit, A. Ethirajan, M. Muller and B. Conings, Nat. Mater., 2016, 15, 247 CrossRef CAS PubMed.
- A. L. Wani, A. Ara and J. A. Usmani, Interdiscip. Toxicol., 2015, 8, 55 CrossRef CAS PubMed.
- Y. Dang, Y. Zhou, X. Liu, D. Ju, S. Xia, H. Xia and X. Tao, Angew. Chem., Int. Ed., 2016, 55, 3447 CrossRef CAS PubMed.
- L. He, H. Gu, X. Liu, P. Li, Y. Dang, C. Liang, L. K. Ono, Y. Qi and X. Tao, Matter, 2020, 2, 167 CrossRef.
- Y. Dang, C. Zhong, G. Zhang, D. Ju, L. Wang, S. Xia, H. Xia and X. Tao, Chem. Mater., 2016, 28, 6968 CrossRef CAS.
- J. Zhao, T. Zhang, X.-Y. Dong, M.-E. Sun, C. Zhang, X. Li, Y. Zhao and S.-Q. Zang, J. Am. Chem. Soc., 2019, 141, 15755 CrossRef CAS PubMed.
- G. Hu, B. Xu, A. Wang, Y. Guo, J. Wu, F. Muhammad, W. Meng, C. Wang, S. Sui, Y. Liu, Y. Li, Y. Zhang, Y. Zhou and Z. Deng, Adv. Funct. Mater., 2021, 31, 2011191 CrossRef CAS.
- L. Xie, B. Chen, F. Zhang, Z. Zhao, X. Wang, L. Shi, Y. Liu, L. Huang, R. Liu, B. Zou and Y. Wang, Photonics Res., 2020, 8, 768 CrossRef CAS.
- Z. Xiao, K.-Z. Du, W. Meng, D. B. Mitzi and Y. Yan, Angew. Chem., Int. Ed., 2017, 56, 12107 CrossRef CAS PubMed.
- Z. Xiao, Z. Song and Y. Yan, Adv. Mater., 2019, 31, 1803792 CrossRef CAS PubMed.
- J. Lin, H. Chen, J. Kang, L. N. Quan, Z. Lin, Q. Kong, M. Lai, S. Yu, L. Wang, L. Wang, M. F. Toney and P. Yang, Matter, 2019, 1, 180 CrossRef.
- C. Chen, X. Zhang, G. Wu, H. Li and H.-Z. Chen, Adv. Opt. Mater., 2017, 5, 1600539 CrossRef.
- L. Hou, Y. Zhu, J. Zhu, Y. Gong and C. Li, J. Mater. Chem. C, 2020, 8, 8502 RSC.
- J.-H. Wei, J.-F. Liao, X.-D. Wang, L. Zhou, Y. Jiang and D.-B. Kuang, Matter, 2020, 3, 892 CrossRef.
- B. A. Connor, R. W. Smaha, J. Li, A. Gold-Parker, A. J. Heyer, M. F. Toney, Y. S. Lee and H. I. Karunadasa, Chem. Sci., 2021, 12, 8689 RSC.
- M. Wang, W. Wang, B. Ma, W. Shen, L. Liu, K. Cao, S. Chen and W. Huang, Nano-Micro Lett., 2021, 13, 62 CrossRef PubMed.
- R. D. Willett, R. E. Butcher, C. P. Landee and B. Twamley, Polyhedron, 2006, 25, 2093 CrossRef CAS.
- C. Pareja-Rivera and D. Solis-Ibarra, Adv. Opt. Mater., 2021, 9, 2100633 CrossRef CAS.
- T. Jun, K. Sim, S. Iimura, M. Sasase, H. Kamioka, J. Kim and H. Hosono, Adv. Mater., 2018, 30, 1804547 CrossRef PubMed.
- X. Mo, T. Li, F. Huang, Z. Li, Y. Zhou, T. Lin, Y. Ouyang, X. Tao and C. Pan, Nano Energy, 2021, 81, 105570 CrossRef CAS.
- T. D. Creason, T. M. McWhorter, Z. Bell, M. Du and B. Saparov, Chem. Mater., 2020, 32, 6197–6205 CrossRef CAS.
- R. Roccanova, A. Yangui, H. Nhalil, H. Shi, M.-H. Du and B. Saparov, ACS Appl. Electron. Mater., 2019, 1, 269 CrossRef CAS.
- H. Peng, X. Wang, Y. Tian, B. Zou, F. Yang, T. Huang, C. Peng and S. Yao, ACS Appl. Mater. Interfaces, 2021, 13, 13443 CrossRef CAS PubMed.
- L. Yao, G. Niu, J. Li, L. Gao, X. Luo, B. Xia and Y. Liu, J. Phys. Chem. Lett., 2020, 11, 1255 CrossRef CAS PubMed.
- M. C. Biesinger, Surf. Interface Anal., 2017, 49, 1325 CrossRef CAS.
- P. Cheng, L. Sun, L. Feng, S. Yang, Y. Yang, D. Zheng, Y. Zhao, Y. Sang, R. Zhang, D. Wei, W. Deng and K. Han, Angew. Chem., Int. Ed., 2019, 58, 16087 CrossRef CAS PubMed.
- L. Xie, B. Chen, F. Zhang, Z. Zhao, X. Wang, L. Shi, Y. Liu, L. Huang, R. Liu, B. Zou and Y. Wang, Photonics Res., 2020, 8, 768 CrossRef CAS.
- T. Li, X. Mo, C. Peng, Q. Lu, C. Qi, X. Tao, Y. Ouyang and Y. Zhou, Chem. Commun., 2019, 55, 4554 RSC.
- C. G. Bischak, M. Lai, Z. Fan, D. Lu, P. David, D. Dong, H. Chen, A. S. Etman, T. Lei, J. Sun, M. Grünwald, D. T. Limmer, P. Yang and N. S. Ginsberg, Matter, 2020, 3, 534 CrossRef.
- Z. Li, Y. Sun, H. Yao, J. Zhao, Q. Wang, L. Ding and Z. Jin, J. Energy Chem., 2021, 52, 102 CrossRef.
- S. Liu, H. Liu, G. Zhou, X. Li and S. Wang, Chem. Eng. J., 2022, 427, 131430 CrossRef CAS.
-
W. M. Wendlandt and H. G. Hecht, Reflectance spectroscopy, Wiley Interscience, New York, 1966, p. 62 Search PubMed.
- P. Fu, S. Hu, J. Tang and Z. Xiao, Front. Optoelectron., 2021, 14, 252 CrossRef.
- J. P. Perdew, K. Burke and M. Ernzerhof, Phys. Rev. Lett., 1996, 77, 3865 CrossRef CAS PubMed.
- G. Zhou, B. Su, J. Huang, Q. Zhang and Z. Xia, Mater. Sci. Eng., R, 2020, 141, 100548 CrossRef.
- L. Lian, M. Zheng, P. Zhang, Z. Zheng, K. Du, W. Lei, J. Gao, G. Niu, D. Zhang, T. Zhai, S. Jin, J. Tang, X. Zhang and J. Zhang, Chem. Mater., 2020, 32, 3462 CrossRef CAS.
- H. Chen, J. M. Pina, F. Yuan, A. Johnston, D. Ma, B. Chen, Z. Li, A. Dumont, X. Li, Y. Liu, S. Hoogland, Z. Zajacz, Z. Lu and E. H. Sargent, J. Phys. Chem. Lett., 2020, 11, 4326 CrossRef CAS PubMed.
- B. Zhang, X. Wu, S. Zhou, G. Liang and Q. Hu, Front. Optoelectron., 2021, 14, 459 CrossRef.
- H. Shankar, A. Jha and P. Kar, Mater. Adv., 2022, 3, 658 RSC.
- O. V. Dolomanov, L. J. Bourhis, R. J. Gildea, J. A. K. Howard and H. Puschmann, J. Appl. Crystallogr., 2009, 42, 339 CrossRef CAS.
- L. J. Bourhis, O. V. Dolomanov, R. J. Gildea, J. A. K. Howard and H. Puschmann, Acta Crystallogr., Sect. A: Found. Adv., 2015, 71, 59 CrossRef CAS PubMed.
- G. M. Sheldrick, Acta Crystallogr., Sect. A: Found. Crystallogr., 2008, 64, 112 CrossRef CAS PubMed.
- W. Song, K. Leung, Q. Shao, K. J. Gaskell and J. E. Reutt-Robey, J. Phys. Chem. C, 2016, 120, 22979 CrossRef CAS.
- G. Kresse and J. Furthmüller, Comput. Mater. Sci., 1996, 6, 15 CrossRef CAS.
- G. Kresse and J. Furthmüller, Phys. Rev. B: Condens. Matter Mater. Phys., 1996, 54, 11169–11186 CrossRef CAS PubMed.
- J. P. Perdew, K. Burke and M. Ernzerhof, Phys. Rev. Lett., 1996, 77, 3865 CrossRef CAS PubMed.
- H. J. Monkhorst and J. D. Pack, Phys. Rev. B: Condens. Matter Mater. Phys., 1976, 13, 5188 CrossRef.
Footnote |
† Electronic supplementary information (ESI) available: XPS spectra, crystal data, spatial distributions calculation, TGA/DSC diagrams, PL spectra, PL lifetime and PLQY. CCDC 2113134–2113136. For ESI and crystallographic data in CIF or other electronic format see DOI: 10.1039/d1ma01055g |
|
This journal is © The Royal Society of Chemistry 2022 |
Click here to see how this site uses Cookies. View our privacy policy here.