DOI:
10.1039/D1MA00785H
(Paper)
Mater. Adv., 2022,
3, 1240-1247
Tuning the switching pressure in square lattice coordination networks by metal cation substitution†
Received
31st August 2021
, Accepted 8th December 2021
First published on 10th December 2021
Abstract
Coordination networks that undergo guest-induced switching between “closed” nonporous and “open” porous phases are of increasing interest as the resulting stepped sorption isotherms can offer exceptional working capacities for gas storage applications. For practical utility, the gate ad/desorption pressures (Pga/Pgd) must lie between the storage (Pst) and delivery (Pde) pressures and there must be fast switching kinetics. Herein we study the effect of metal cation substitution on the switching pressure of a family of square lattice coordination networks [M(4,4′-bipyridine)2(NCS)]n (sql-1-M-NCS, M = Fe, Co and Ni) with respect to CO2 sorption. The Clausius–Clapeyron equation was used to correlate Pga/Pgd and temperature. At 298 K, Pga/Pgd values were found to vary from 31.6/26.7 bar (M = Fe) to 26.7/20.9 bar (M = Co) and 18.5/14.6 bar (M = Ni). The switching event occurs within 10 minutes as verified by dynamic CO2 sorption tests. In addition, in situ synchrotron PXRD and molecular simulations provided structural insight into the observed switching event, which we attribute to layer expansion of sql-1-M-NCSvia intercalation and inclusion of CO2 molecules. This study could pave the way for rational control over Pga/Pgd in switching adsorbent layered materials and enhance their potential utility in gas storage applications.
Introduction
New approaches to gas storage/delivery are needed in the “age of gas” to address the large energy footprint associated with existing technologies such as compressed or liquefied gas storage.1 Whereas physisorption holds promise for greatly improving the energy efficiency of industrial gas storage, it does not yet meet the prerequisites for practical deployment such as adsorbed natural gas (ANG) storage.2 This is at least partly because rigid physisorbents such as zeolites tend to suffer from inappropriate sorption isotherms (Langmuir or type I3), resulting in lower working capacity than the saturation uptake (Scheme 1a).4
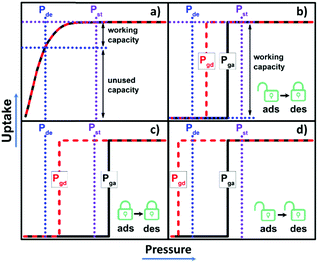 |
| Scheme 1 Comparison of different types of gas sorption isotherms. (a) type I; (b) type F-IV (Pde < Pgd < Pga < Pst); (c) type F-IV (Pde < Pgd < Pst < Pga) and (d) type F-IV (Pgd < Pde < Pga < Pst). Black solid line: adsorption; red dash line: desorption; Pga: gate adsorption pressure; Pgd: gate desorption pressure; Pde: delivery pressure; Pst: storage pressure; ads: adsorption; des: desorption. | |
Metal–organic materials (MOMs),5,6 such as metal–organic frameworks (MOFs),7,8 or porous coordination polymers/networks (PCPs/PCNs),9–12 have attracted attention from academic and industrial researchers thanks to their potential utility in the areas as broad as storage, separation and catalysis.13,14 A prominent feature of MOMs is their inherent modularity which enables fine tuning of structures through crystal engineering or reticular chemistry.15,16 However, although ca. 1
00
000 MOMs have been deposited in the MOF subset of the Cambridge Structural Database (CSD),17 most are rigid physisorbents that exhibit the aforementioned type I sorption isotherms. In contrast, flexible MOMs (FMOMs) or soft porous crystals (SPCs) can undergo guest-induced switching between nonporous and porous phases and exhibit perhaps the most desirable isotherm type, a stepped or type F-IV isotherm.18–20 The optimal type F-IV isotherm would offer gate adsorption (Pga) and desorption (Pgd) pressures that lie between the storage (Pst) and delivery (Pde) pressures to enable high working capacity (Scheme 1b). There is little margin for error, however, as FMOMs that remain closed during adsorption (Pga > Pst, Scheme 1c) or open during desorption (Pgd < Pde, Scheme 1d) will offer negligible working capacity. Furthermore, gas storage criteria for Pst and Pde could vary significantly in different circumstances. The ability to fine-tune the switching pressures in FMOMs is thus important to enable the development of bespoke adsorbent materials for gas storage/delivery. Thus far, to our knowledge, only a relatively small subset of FMOMs feature type F-IV isotherms and even fewer have been subjected to systematic studies that address how to control their switching pressures.21
Crystal-engineering approaches can be exploited to tailor structures, compositions and, therefore, properties of MOMs.22 In the context of FMOMs, ligand “linker” or metal “node” substitution can be used to modulate flexibility.23–26 Ligand functionalization was used to adjust the switching of parent sorbents such as MIL-53(Fe) and [Co(bdp)].27–29 Regarding ligand substitution, we recently reported a family of two-fold interpenetrated FMOMs, X-pcu-n-Zn (n = 5–8), which exhibited pronounced switching behavior with comparable uptakes but different switching pressures.30,31 Considerable attention has been paid to exploiting metal cation substitution to modulate FMOM platforms, however, it can be a challenge to retain the type F-IV sorption profile.32–37 For example, MIL-53(Cr or Al) were found to exhibit type F-I isotherms for CO2 sorption,32 while MIL-53(Fe or Sc) featured type F-IV isotherms.33,34 In addition, the Co and Fe variants of [M(bdp)] exhibited type F-IV isotherms,4 but their Zn and Ni analogues showed type I isotherms.35 The pillared-layer coordination networks, DUT-8(M), exhibited type F-IV (DUT-8(Ni)), type F-I (DUT-8(Co)) or type I (DUT-8(Cu)) isotherms upon CO2 sorption.36 Even though the zeolitic imidazolate frameworks [M(bim)2] (M = Co, Zn and Cd) retained their switching nature, there a significant pre-step was observed in the Co and Zn variants.37 Herein we address the use of metal cation substitution to tune the switching pressure in a family of three square lattice (sql) coordination networks [M(bpy)2(NCS)2]n, sql-1-M-NCS (1 = bpy = 4,4′-bipyridine, M = Fe, Co and Ni).
Square lattice (sql) coordination networks account for nearly half of reported 2D coordination networks (CNs).38sql CNs with general formula [M(L)2(A)2] can be readily formed by self-assembly of octahedral metal ions (M), axial counter anions (A) and linear linker ligands (L).39 The prototypal non-interpenetrated sql CN, [Cd(bpy)2(NO3)2]n, was reported by Fujita's group in 1994.40 This seminal work was followed by a series of studies that focused more upon the structural features of such networks rather than sorption properties until [Cu(bpy)2(BF4)2] (ELM-11) was investigated.41,42 ELM-11 was found to exhibit switching when exposed to gases such as CO2, C2H2 and n-butane.43–47 Its metal cation substituted analogue, [Ni(bpy)2(BF4)2] (ELM-31), also exhibits a type F-IV isotherm upon CO2 sorption but with different uptake and switching pressure.48 Nevertheless, the metal substitution strategy has not yet been fully exploited in the ELM-11 family.
Recently, our group reported the sorption properties of a previously known sql CN [Co(bpy)2(NCS)2],49sql-1-Co-NCS,50,51 which is closely related to the ELM family.43,44 The 195 K CO2 sorption isotherm of sql-1-Co-NCS was observed to be type F-IV.50 High-pressure CO2 sorption at different temperatures exhibited type F-IV sorption profiles and the temperature vs switching pressure obeyed the Clausius–Clapeyron equation. Crystallographic studies revealed that the interlayer distance in the closed phase (4.5 Å) increased in the CO2 loaded phase (5.4 Å), although the location of CO2 molecules was not determined.50 In this contribution, we report the Fe and Ni analogues of sql-1-Co-NCS and their CO2 sorption profiles and switching kinetics.
Results and discussion
sql-1-M-NCS (M = Fe and Ni) were synthesized in powder form (rod shape, 0.5–2 μm, Fig. S14, ESI†) by heating their precursors {[M(bpy)(NCS)2(H2O)2]·bpy}n at 50 °C in vacuo. These precursors were prepared by a water slurry method. The crystal structures of sql-1-Co-NCS and sql-1-Ni-NCS were previously determined by single-crystal X-ray diffraction50,52 and we determined the crystal structure of sql-1-Fe-NCS herein by refinement of synchrotron PXRD data (Fig. S1 and S3a, ESI†). The three sql-1-M-NCS CNs are comprised of M(II) cations coordinated to four equatorial bpy ligands and two axial NCS− ligands and they are isostructural. Their synchrotron PXRD patterns match well (Fig. S1, ESI†). The effective cavity sizes (7.5 Å × 7.5 Å) and interlayer distances (4.5 Å) are also equivalent, although there are minor differences in the torsion angle of the bpy linker ligands (54.3°–54.7°) and the M–M–M (75.3°–76.4°) and M–N–CS (169.5°–170.9°) angles (Fig. 1). Terminal NCS ligands interdigitate adjacent layers (Fig. 1d–f) and block the cavities.
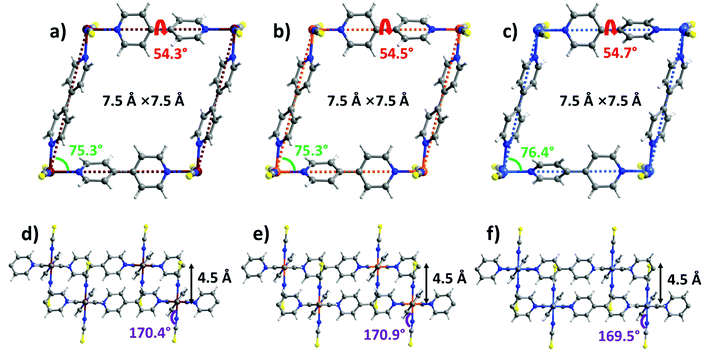 |
| Fig. 1 Comparison of the crystal structures of sql-1-M-NCS. (a and d) M = Fe; (b and e) M = Co; (c and f) M = Ni. | |
Although the cavity size and interlayer distance of sql-1-M-NCS are comparable to those of ELM-11, the hydrophilicity of sql-1-M-NCS towards humidity is mitigated thanks to utilisation of the relatively hydrophobic NCS− anion (Fig. S4, ESI†). Thermogravimetric analysis (TGA) revealed that sql-1-Ni-NCS exhibits better thermal stability (ca. 180 °C) than its Fe and Co analogues (ca. 150 °C) (Fig. S5, ESI†), which follows the empirical Irving–Williams series.53 195 K CO2 sorption studies revealed that sql-1-M-NCS CNs exhibit the same saturation uptake (ca. 138 cm−3 g−1), equivalent to three CO2 molecules per formula unit (denoted as sql-1-M-NCS·3CO2), but with distinct Pga/Pgd values: Fe (15/12 kPa) > Co (10/8 kPa) > Ni (4/3.5 kPa) (Fig. 2). Unlike ELM-11 which exhibits multi-step CO2 sorption isotherms at 195 K,54,55sql-1-M-NCS registered single-step sorption isotherms although sql-1-Fe-NCS had a small sub-step with 17 cm−3 g−1 uptake between 11 and 15 kPa.
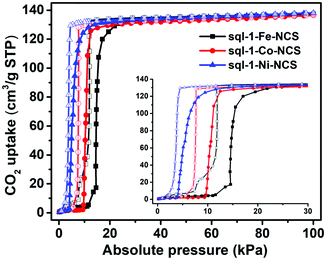 |
| Fig. 2 CO2 sorption isotherms for sql-1-M-NCS (M = Fe, Co, and Ni) at 195 K. The solid and open symbols represent adsorption and desorption branches, respectively. | |
We next collected high-pressure (up to 38 bar) CO2 sorption isotherms on sql-1-M-NCS (M = Fe and Ni) between 273 and 298 K (5 K intervals) as previously conducted for sql-1-Co-NCS (Fig. 3a, b and Fig. S6, ESI†). The Pga/Pgd values for sql-1-Fe-NCS were 12.5/10.5, 15.2/12.8, 18.5/15.5, 22.3/18.7, 26.6/22.6 and 31.6/26.7 bar from 273 to 298 K, respectively. In contrast, sql-1-Ni-NCS was found to offer lower Pga/Pgd values: 6.5/5.1, 8.1/6.4, 10.1/7.9, 12.5/9.8, 15.2/11.9 and 18.5/14.5 bar from 273 to 298 K, respectively. The CO2 sorption isotherms of sql-1-M-NCS (M = Fe and Ni) revealed a relationship between the Pga/Pgd and temperature which obeys the Clausius–Clapeyron equation d
ln
P/(d(1/T)) = (±)ΔH/R (Fig. 3c, d and Fig. S7, ESI†). The phase transition enthalpies ΔH (the signs of ΔH are negative/positive for adsorption/desorption, respectively, absolute values are used hereafter) were calculated to be ca. 25.2 and 28.4 kJ mol−1 for sql-1-Fe-NCS and sql-1-Ni-NCS, respectively, 1.0 kJ mol−1 lower and 2.2 kJ mol−1 higher than that of sql-1-Co-NCS (26.2 kJ mol−1).50
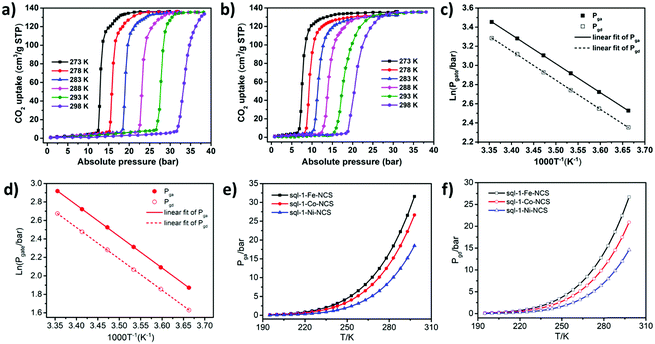 |
| Fig. 3 High-pressure CO2 sorption isotherms of (a) sql-1-Fe-NCS and (b) sql-1-Ni-NCS. The linear fit of gate sorption pressure (LnP) and temperature (1000 T−1) using the Clausius–Clapeyron equation for (c) sql-1-Fe-NCS and (d) sql-1-Ni-NCS. (e) Calculated Pga and (f) Pgd for sql-1-M-NCS (M = Fe, Co, and Ni). | |
One advantage of switching CNs is the switching pressure can be calculated at a given temperature once ΔH in the Clausius–Clapeyron equation has been determined.47,50 We thus plotted calculated switching pressure vs temperature from 195 to 298 K for sql-1-M-NCS (M = Fe, Co and Ni) (Fig. 3e, f and Fig. S8, Table S1, ESI†). The Pga and Pgd were thereby tuned by the metal centers: Pga/Pgd (Fe) > Pga/Pgd (Co) > Pga/Pgd (Ni). Since the switching pressure is exponentially correlated to the temperature, it increases substantially at elevated temperature. For example, at 298 K, the Pga/Pgd were calculated to be 31.6/26.7, 26.7/20.9, and 18.5/14.6 bar when M = Fe, Co and Ni, respectively, two orders of magnitude higher than at 195 K. The magnitude of the hysteresis between Pga and Pgd was also affected by temperature (Fig. S8, ESI†). The hysteresis gaps were found to be 4.9 (Fe), 5.8 (Co) and 3.9 (Ni) bar at 298 K, 163, 290, and 780 times their values (3.0, 2.0, and 0.5 kPa) at 195 K. The hysteresis in sql-1-M-NCS suggests that CO2 can be stored at lower pressure (between Pgd and Pga) than the charging pressure (Pga or above).
When compared to other switching CNs which exhibit wide ranges of CO2 uptakes (40–590 cm−3 g−1) and switching pressures (1.3–75 kPa) at 195 K (Table S2 and Fig. S9a, ESI†),21sql-1-M-NCS exhibit moderate uptakes and relatively low switching pressures. At elevated temperature (e.g. 298 K), sql-1-M-NCS retained almost the same uptake, whereas some benchmark switching CNs like DUT-8(Ni) and X-pcu-n-Zn tend to exhibit lower or even negligible CO2 uptakes (Table S2 and Fig. S9b, ESI†),31,36 due to Pga values (e.g. >35 bar) that are beyond the maximum testing pressures. The prototypical switching sql CN, ELM-11, was observed to exhibit 80 and 173 cm−3 g−1 of CO2 uptake at the first (Pga1 = 0.65 bar) and second steps (Pga2 = 19 bar) at 298 K.54 The distinct differences between ELM-11 and sql-1-M-NCS demonstrate the feasibility of tuning the switching pressure and even the uptake in families of sql-1 CNs.
The sorption kinetics of sorbents is also relevant to their storage and separation performance,56–58 especially for FMOMs featuring structural transitions that might control the kinetics.51,59–61 We conducted dynamic CO2 sorption studies on sql-1-M-NCS gravimetrically at 288 K and 1–36 bar. The CO2 pressure was elevated/reduced constantly with a maximum rate of 2.5 bar min−1 for adsorption/desorption process. It was observed that sql-1-M-NCS started adsorbing/desorbing CO2 at 11.5/7.8, 10.0/9.5 and 7.5/10.8 min (equivalent to 23.9/18.0, 20.0/13.9 and 13.5/10.7 bar) for M = Fe, Co and Ni, respectively (Fig. 4). It is consistent with the Pga/Pgd values (22.2/18.7, 18.5/14.5 and 12.4/9.8 bar) calculated from the Clausius–Clapeyron equation at 288 K. We determined that it took 7.5/5, 6/6 and 5/7.5 min to reach full loading/unloading for sql-1-M-NCS (M = Fe, Co, and Ni, respectively), indicating ca. 12.5 min for a sorption cycle and 22 cm−3 g−1 min−1 for the average sorption rate. It was reported that ELM-11 reaches full loading of CH4 (ca. 80 cm−3 g−1) in 10 min at 303 K.43 Such sorption kinetics of sql CNs is comparable or even superior to some rigid sorbents.56,62
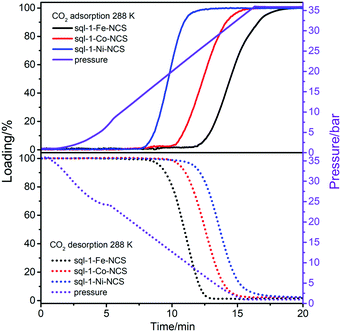 |
| Fig. 4 Kinetics of CO2 adsorption (top) and desorption (bottom) for sql-1-M-NCS (M = Fe, Co and Ni) at 288 K. | |
To better understand the switching mechanism of sql-1-M-NCS, in situ CO2 loaded synchrotron PXRD experiments (Fig. S2, ESI†) and molecular simulations were conducted. As revealed by Fig. 5, the cavity size of sql-1-M-NCS·3CO2 remains unchanged compared to that of sql-1-M-NCS, however, the cavity geometry deforms from a rhombus in the closed phase to a square in the open phase accompanied by an interlayer distance increase from 4.5 to 5.4 Å. Such intra-network and inter-network deformations induced by guest molecules are quite common amongst switching and flexible CNs.21,63,64 In addition, the bpy ligand twists by 10.3° (Fe), 9.6° (Co), and 8.9° (Ni) to accommodate the CO2 molecules which occupy the interlayer and cavity voids as visualised by molecular simulations. It also reveals that [CO2]3 cluster units are formed (C⋯O distance: 2.755–3.265 Å) in the cavities of sql-1-M-NCS·3CO2 and propagate along a axis (Fig. S10–S12, ESI†). Each strand of {[CO2]3}n is constrained in half of the cavity thanks to the ABAB stack mode of layers. Notably, the M–N–CS angles are compressed to 144.9° (Fe), 156.1° (Co) and 162.1° (Ni) in the open phases, corresponding to a change of 25.5°, 14.8°, and 7.4° from those in the closed phases, respectively. Overall, transformations between sql-1-M-NCS and sql-1-M-NCS·3CO2 followed the trend Pga (Fe) > Pga (Co) > Pga (Ni), corresponding enthalpy changes being 25.2 (Fe) < 26.2 (Ni) < 28.4 (Ni) kJ mol−1 (exothermic or endothermic for adsorption or desorption, respectively). Such trends could be attributable ligand twisting, metal coordination sphere distortion, layered net expansion and/or sorbate/sorbent interactions. The isostructural nature within each set of three phases suggests that, as for many trends in d-block metals, electronic configurations (d6, d7 and d8), which influence metal–ligand bonding and electrostatics, are behind the observed trends. A similar situation was reported for metal substituted variants of ZIF-7.37
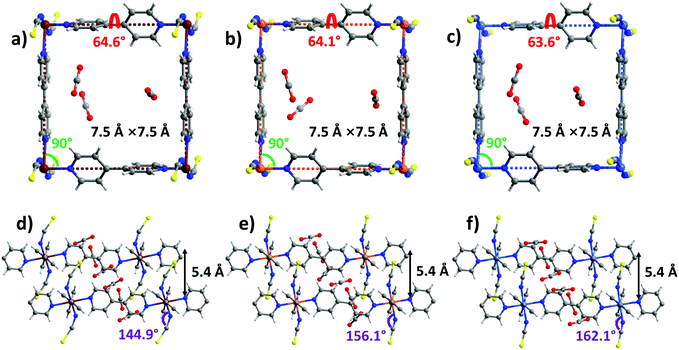 |
| Fig. 5 Comparison of the crystal structures of sql-1-M-NCS·3CO2. (a and d) M = Fe; (b and e) M = Co; (c and f) M = Ni. | |
Conclusions
In conclusion, we report herein the switching behavior of three sql-1-M-NCS CNs through CO2 sorption under equilibrium and dynamic conditions. Although metal cation substitution is a simple crystal engineering strategy for modulating the properties of MOMs, to our knowledge this is the first example of switching CNs that retain stepped sorption isotherms across three metal nodes. Crystallographic and modelling studies reveal that the metal centers affect the conformations in sql-1-M-NCS·3CO2, which in turn reflect the switching pressure required for the structural transition. While several metrics such as kinetics, hydrophobicity, and strong stability to regeneration, as exemplified by sql-1-Co-NCS,50 are favorable in sql-1-M-NCS, their working capacity, a key metric for gas storage, could be improved. This is particularly the case for ANG, for which the latest DOE target is 700 cm−3 g−1 or 263 cm−3 cm−3.65 Unfortunately, even the “softest” sql-1-M-NCS (i.e.sql-1-Ni-NCS) exhibited negligible CH4 uptake below 60 bar at 298 K (Fig. S13, ESI†). This is most likely because CH4 interacts weakly with host frameworks compared to CO2. In contrast, stronger binding aromatics such as xylenes adsorb ca. 87 wt% in sql-1-Co-NCS and we recently reported that ELM-11 (sql-1-Cu-BF4) adsorbs up to 245 cm−3 g−1 of C2H2via four sorption steps.47,51 The findings herein suggest that adsorbate-dependent uptake and switching pressure in families of sql CNs are likely to be a common feature of such switching CNs and the study of other hydrocarbons will be conducted.
Experimental section
Synthesis of square lattice (sql) coordination networks
[Fe(bpy)2(NCS)2]n (sql-1-Fe-NCS) was prepared by a water slurry method. FeSO4·7H2O (10 mmol, 2.78 g), NaSCN (20 mmol, 1.62 g) and 4,4′-bipyridine (20 mmol, 3.12 g) were added to 50 mL water in a 100 mL bottle. The slurry was stirred continuously for 3 h at room temperature to form the precursor {[Fe(bpy)(NCS)2(H2O)2] bpy}n which was then filtered, washed with water and air-dried (yield ∼95%). The precursor powder was activated at 50 °C in vacuo for 5 h to transform to the sql coordination network: sql-1-Fe-NCS.
[Ni(bpy)2(NCS)2]n (sql-1-Ni-NCS) was prepared by the same method as described above except that the metal salt FeSO4·7H2O was replaced by NiSO4·6H2O (10 mmol, 2.63 g).
Synchrotron powder X-ray diffraction
Synchrotron PXRD data was obtained from beamline I11 at the Diamond Light Source (λ = 0.82455(2) Å and zero point = −0.01826(1)°). Powder sample sealed in a Φ = 0.5 mm capillary tube was measured at 195 K under vacuum using positional scanning detector (PSD). After that, CO2 (195 K, 1 bar) was filled into the capillary tube and powder X-ray data was collected until sample stabilized. The data was used for structure solution and refinement of sql-1-Fe-NCS and sql-1-M-NCS 3CO2 (M = Fe and Ni). Analysis of the powder data was carried out in TOPAS Academic and FullProf. The previously reported crystal structures of sql-1-Co-NCS and sql-1-Co-NCS 3CO2 were used as starting templates.50 Lattice parameters were determined using the Pawley method. The CO2 location was not determined by synchrotron PXRD refinement while it was modelled by molecular simulation (see simulation section below). Crystallographic data (CCDC number: 2106581–2106583) is summarized in Table S3 (ESI†) and comparative patterns for the observed and calculated intensities including their differences are presented in Fig. S3 (ESI†).
Density functional theory (DFT) calculations
All periodic DFT calculations were carried out using the Castep as implemented in the Materials Studio package. Vanderbilt-type ultrasoft pseudopotentials and the generalized gradient approximation (GGA) with the Perdew–Burke–Ernzerhof (PBE) exchange correlation were used for all structure calculations. A semi-empirical dispersion correction was included in the calculation to take the van der Waals interactions into account. A cutoff energy of 544 eV and a 2 × 3 × 2 k-point mesh (generated using the Monkhorst–Pack scheme) were found to be sufficient for total energy to converge within 0.01 meV atom−1. The starting structures (sql-1-M-NCS·3CO2) were obtained from the synchrotron PXRD refinement as described above. Three CO2 molecules per formula unit were added into each structure based on CO2 sorption studies and were set flexible to allow the optimization of their binding sites. All the simulations on the structures were conducted in P1 space group.
Thermogravimetric analysis (TGA)
TGA for all the compounds were carried out under N2 atmosphere in a TA instruments Q50 thermal analyzer between room temperature and 300 °C with a constant heating rate of 10 °C min−1.
Scanning electron microscopy (SEM)
SEM analysis was performed using an SU 70 Hitachi instrument. The samples were sputter-coated with gold (20 mA, 90 s) prior to imaging.
Low pressure CO2 adsorption
Low pressure CO2 adsorption experiments (up to 1 bar) of sql-1-M-NCS (M = Fe and Ni) were conducted on the Micromeritics TriStar II PLUS 3030 instrument at 195 K which was maintained by a 4 L Dewar flask filled with the mixture of acetone and dry ice. High-purity CO2 was used as received from BOC Gases Ireland, CP grade (99.995%).
High pressure CO2 adsorption
High pressure CO2 isotherms of sql-1-M-NCS (M = Fe and Ni) were collected on a Micromeritics HPVA II-100 instrument at different temperatures (273–298 K). The activated sample (ca. 300 mg) was further degassed in situ for 1 hour before the measurements. Free spaces were determined at 0.7 bar Helium (He) and 25 °C. A background correction was performed by subtracting the adsorption of the empty sample cell from the obtained isotherms.
Water vapor sorption
Water vapor sorption measurements were conducted using a Surface Measurement Systems DVS Vacuum at 298 K. Samples of ELM-11 (purchased from TCI) and sql-1-M-NCS (M = Fe and Ni) were further degassed under vacuum (1 × 10−4 Torr) in situ and stepwise increase in relative pressure from 0 to 90% were controlled by equilibrated weight changes of the sample (dM/dT = 0.01% min−1). Vacuum pressure transducers were used with ability to measure from 1 × 10−6 to 760 Torr with a resolution of 0.01%. Approximately 10 mg of sample was used for each experiment. The mass of the sample was determined by comparison to an empty reference pan and recorded by a high-resolution microbalance with a precision of 0.1 μg.
Dynamic CO2 sorption study
Dynamic CO2 sorption experiments were carried out using a Hiden Isochema XEMIS microbalance at 288 K. In each experiment, around 20 mg of sample (sql-1-M-NCS) was used and further evacuated in situ at 323 K for 5 h. Pressure was then maintained at 1 bar without pumping out until mass equilibrium was reached. The pressure was first elevated from 1 to 36 bar, held until mass equilibrium was reached, and then reduced from 36 to 1 bar with a maximum constant rate of 2.5 bar per min. Background calibration was applied by repeating the same procedure except that the sql-1-M-NCS sample was replaced by a 20 mg counterweight in the sample cell.
Author contributions
Shi-Qiang Wang: conceptualization, methodology, investigation, formal analysis, writing – original draft; Shaza Darwish: investigation, writing – review & editing; Debobroto Sensharma: investigation, writing – review & editing; Michael J. Zaworotko: supervision, funding acquisition, writing – review & editing.
Conflicts of interest
There are no conflicts to declare.
Acknowledgements
M. J. Z. would like to acknowledge the support of Irish Research Council (IRCLA/2019/167) and Science Foundation Ireland (13/RP/B2549 and 16/IA/4624). We thank Dr Claire Murray and Dr Chiu C. Tang at the Diamond Light Source, UK for the access to the synchrotron X-ray diffraction beamline i11 (EE20500). S.-Q. W. would also like to thank his colleagues, Mr Daniel O’Hearn, Dr Andrey Bezrukov and Dr Soumya Mukherjee, for their assistance at Diamond Light Source.
Notes and references
- S. Kitagawa, Angew. Chem., Int. Ed., 2015, 54, 10686–10687 CrossRef CAS PubMed.
- C. M. Simon, J. Kim, D. A. Gomez-Gualdron, J. S. Camp, Y. G. Chung, R. L. Martin, R. Mercado, M. W. Deem, D. Gunter, M. Haranczyk, D. S. Sholl, R. Q. Snurr and B. Smit, Energy Environ. Sci., 2015, 8, 1190–1199 RSC.
- M. Thommes, K. Kaneko, A. V. Neimark, J. P. Olivier, F. Rodriguez-Reinoso, J. Rouquerol and K. S. Sing, Pure Appl. Chem., 2015, 87, 1051–1069 CAS.
- J. A. Mason, J. Oktawiec, M. K. Taylor, M. R. Hudson, J. Rodriguez, J. E. Bachman, M. I. Gonzalez, A. Cervellino, A. Guagliardi, C. M. Brown, P. L. Llewellyn, N. Masciocchi and J. R. Long, Nature, 2015, 527, 357–361 CrossRef CAS PubMed.
- J. J. Perry IV, J. A. Perman and M. J. Zaworotko, Chem. Soc. Rev., 2009, 38, 1400–1417 RSC.
- T. R. Cook, Y.-R. Zheng and P. J. Stang, Chem. Rev., 2012, 113, 734–777 CrossRef PubMed.
-
L. R. MacGillivray, Metal-organic frameworks: design and application, John Wiley & Sons, 2010 Search PubMed.
-
D. Farrusseng, Metal-organic frameworks: applications from catalysis to gas storage, John Wiley & Sons, 2011 Search PubMed.
- S. Kitagawa, R. Kitaura and S. I. Noro, Angew. Chem., Int. Ed., 2004, 43, 2334–2375 CrossRef CAS PubMed.
-
S. R. Batten, S. M. Neville and D. R. Turner, Coordination polymers: design, analysis and application, Royal Society of Chemistry, 2009 Search PubMed.
- C. Janiak and J. K. Vieth, New J. Chem., 2010, 34, 2366–2388 RSC.
- D. J. O'Hearn, A. Bajpai and M. J. Zaworotko, Small, 2021, 2006351 CrossRef PubMed.
- J.-R. Li, R. J. Kuppler and H.-C. Zhou, Chem. Soc. Rev., 2009, 38, 1477–1504 RSC.
- B. Li, H.-M. Wen, Y. Cui, W. Zhou, G. Qian and B. Chen, Adv. Mater., 2016, 28, 8819–8860 CrossRef CAS PubMed.
- B. Moulton and M. J. Zaworotko, Chem. Rev., 2001, 101, 1629–1658 CrossRef CAS PubMed.
- N. W. Ockwig, O. Delgado-Friedrichs, M. O’Keeffe and O. M. Yaghi, Acc. Chem. Res., 2005, 38, 176–182 CrossRef CAS PubMed.
- P. Z. Moghadam, A. Li, X.-W. Liu, R. Bueno-Perez, S.-D. Wang, S. B. Wiggin, P. A. Wood and D. Fairen-Jimenez, Chem. Sci., 2020, 11, 8373–8387 RSC.
- A. Schneemann, V. Bon, I. Schwedler, I. Senkovska, S. Kaskel and R. A. Fischer, Chem. Soc. Rev., 2014, 43, 6062–6096 RSC.
- S. Horike, S. Shimomura and S. Kitagawa, Nat. Chem., 2009, 1, 695–704 CrossRef CAS PubMed.
- Q.-Y. Yang, P. Lama, S. Sen, M. Lusi, K. J. Chen, W. Y. Gao, M. Shivanna, T. Pham, N. Hosono, S. Kusaka, J. J. IV. Perry, S. Ma, B. Space, L. J. Barbour, S. Kitagawa and M. J. Zaworotko, Angew. Chem., Int. Ed., 2018, 57, 5684–5689 CrossRef CAS PubMed.
- S.-Q. Wang, S. Mukherjee and M. J. Zaworotko, Faraday Discuss., 2021, 231, 9–50 RSC.
- S. Mukherjee and M. J. Zaworotko, Trends Chem., 2020, 2, 506–518 CrossRef CAS.
- J.-P. Zhang, H.-L. Zhou, D.-D. Zhou, P.-Q. Liao and X.-M. Chen, Natl. Sci. Rev., 2018, 5, 907–919 CrossRef CAS.
- S. K. Elsaidi, M. H. Mohamed, D. Banerjee and P. K. Thallapally, Coord. Chem. Rev., 2018, 358, 125–152 CrossRef CAS.
- S. Henke, A. Schneemann, A. Wuetscher and R. A. Fischer, J. Am. Chem. Soc., 2012, 134, 9464–9474 CrossRef CAS PubMed.
- A. Schneemann, P. Vervoorts, I. Hante, M. Tu, S. Wannapaiboon, C. Sternemann, M. Paulus, D. C. F. Wieland, S. Henke and R. A. Fischer, Chem. Mater., 2018, 30, 1667–1676 CrossRef CAS.
- N. A. Ramsahye, T.-K. Trung, S. Bourrelly, Q.-Y. Yang, T. Devic, G. Maurin, P. Horcajada, P. L. Llewellyn, P. Yot, C. Serre, Y. Filinchuk, F. Fajula, G. Ferey and P. Trens, J. Phys. Chem. C, 2011, 115, 18683–18695 CrossRef CAS.
- T. Devic, F. Salles, S. Bourrelly, B. Moulin, G. Maurin, P. Horcajada, C. Serre, A. Vimont, J.-C. Lavalley, H. Leclerc, G. Clet, M. Daturi, P. L. Llewellyn, Y. Filinchuk and G. Ferey, J. Mater. Chem., 2012, 22, 10266–10273 RSC.
- M. K. Taylor, T. Runčevski, J. Oktawiec, M. I. Gonzalez, R. L. Siegelman, J. A. Mason, J. Ye, C. M. Brown and J. R. Long, J. Am. Chem. Soc., 2016, 138, 15019–15026 CrossRef CAS PubMed.
- A.-X. Zhu, Q.-Y. Yang, A. Kumar, C. Crowley, S. Mukherjee, K.-J. Chen, S.-Q. Wang, D. O’Nolan, M. Shivanna and M. J. Zaworotko, J. Am. Chem. Soc., 2018, 140, 15572–15576 CrossRef CAS PubMed.
- A.-X. Zhu, Q.-Y. Yang, S. Mukherjee, A. Kumar, C.-H. Deng, A. A. Bezrukov, M. Shivanna and M. J. Zaworotko, Angew. Chem., Int. Ed., 2019, 58, 18212–18217 CrossRef CAS PubMed.
- S. Bourrelly, P. L. Llewellyn, C. Serre, F. Millange, T. Loiseau and G. Férey, J. Am. Chem. Soc., 2005, 127, 13519–13521 CrossRef CAS PubMed.
- P. L. Llewellyn, P. Horcajada, G. Maurin, T. Devic, N. Rosenbach, S. Bourrelly, C. Serre, D. Vincent, S. Loera-Serna, Y. Filinchuk and G. Ferey, J. Am. Chem. Soc., 2009, 131, 13002–13008 CrossRef CAS PubMed.
- J. P. Mowat, V. R. Seymour, J. M. Griffin, S. P. Thompson, A. M. Slawin, D. Fairen-Jimenez, T. Düren, S. E. Ashbrook and P. A. Wright, Dalton Trans., 2012, 41, 3937–3941 RSC.
- S. Galli, N. Masciocchi, V. Colombo, A. Maspero, G. Palmisano, F. J. López-Garzón, M. Domingo-García, I. Fernández-Morales, E. Barea and J. A. R. Navarro, Chem. Mater., 2010, 22, 1664–1672 CrossRef CAS.
- N. Klein, H. C. Hoffmann, A. Cadiau, J. Getzschmann, M. R. Lohe, S. Paasch, T. Heydenreich, K. Adil, I. Senkovska, E. Brunner and K. Stefan, J. Mater. Chem., 2012, 22, 10303–10312 RSC.
- C. M. McGuirk, T. Runčevski, J. Oktawiec, A. Turkiewicz, M. K. Taylor and J. R. Long, J. Am. Chem. Soc., 2018, 140, 15924–15933 CrossRef CAS PubMed.
- T. G. Mitina and V. A. Blatov, Cryst. Growth Des., 2013, 13, 1655–1664 CrossRef CAS.
- M. J. Zaworotko, Chem. Commun., 2001, 1–9 RSC.
- M. Fujita, Y. J. Kwon, S. Washizu and K. Ogura, J. Am. Chem. Soc., 1994, 116, 1151–1152 CrossRef CAS.
- D. Li and K. Kaneko, Chem. Phys. Lett., 2001, 335, 50–56 CrossRef CAS.
- A. Kondo, H. Noguchi, S. Ohnishi, H. Kajiro, A. Tohdoh, Y. Hattori, W.-C. Xu, H. Tanaka, H. Kanoh and K. Kaneko, Nano Lett., 2006, 6, 2581–2584 CrossRef CAS PubMed.
- H. Kanoh, A. Kondo, H. Noguchi, H. Kajiro, A. Tohdoh, Y. Hattori, W.-C. Xu, M. Inoue, T. Sugiura, K. Morita, H. Tanaka, T. Ohba and K. Kaneko, J. Colloid Interface Sci., 2009, 334, 1–7 CrossRef CAS PubMed.
- H. Kajiro, A. Kondo, K. Kaneko and H. Kanoh, Int. J. Mol. Sci., 2010, 11, 3803–3845 CrossRef CAS PubMed.
- V. Bon, I. Senkovska, D. Wallacher, A. Heerwig, N. Klein, I. Zizak, R. Feyerherm, E. Dudzik and S. Kaskel, Microporous Mesoporous Mater., 2014, 188, 190–195 CrossRef CAS.
- L. Li, R. Krishna, Y. Wang, X. Wang, J. Yang and J. Li, Eur. J. Inorg. Chem., 2016, 4457–4462 CrossRef CAS.
- S.-Q. Wang, X.-Q. Meng, M. Vandichel, S. Darwish, Z. Chang, X.-H. Bu and M. J. Zaworotko, ACS Appl. Mater. Interfaces, 2021, 13, 23877–23883 CrossRef CAS PubMed.
- A. Kondo, A. Chinen, H. Kajiro, T. Nakagawa, K. Kato, M. Takata, Y. Hattori, F. Okino, T. Ohba, K. Kaneko and H. Kanoh, Chem. – Eur. J., 2009, 15, 7549–7553 CrossRef CAS PubMed.
- J. Lu, T. Paliwala, S. C. Lim, C. Yu, T. Y. Niu and A. J. Jacobson, Inorg. Chem., 1997, 36, 923–929 CrossRef CAS.
- S.-Q. Wang, Q.-Y. Yang, S. Mukherjee, D. O’Nolan, E. Patyk-Kaźmierczak, K.-J. Chen, M. Shivanna, C. Murray, C. C. Tang and M. J. Zaworotko, Chem. Commun., 2018, 54, 7042–7045 RSC.
- S.-Q. Wang, S. Mukherjee, E. Patyk-Kaźmierczak, S. Darwish, A. Bajpai, Q.-Y. Yang and M. Zaworotko, Angew. Chem., Int. Ed., 2019, 58, 6630–6634 CrossRef CAS PubMed.
- Z. Yugen, J. Li, W. Deng, N. Masayoshi and I. Tsuneo, Chem. Lett., 1999, 195–196 Search PubMed.
- H. Irving and R. J. P. Williams, J. Chem. Soc., 1953, 3192–3210 RSC.
- M. Ichikawa, A. Kondo, H. Noguchi, N. Kojima, T. Ohba, H. Kajiro, Y. Hattori and H. Kanoh, Langmuir, 2016, 32, 9722–9726 CrossRef CAS PubMed.
- S. Hiraide, H. Tanaka, N. Ishikawa and M. T. Miyahara, ACS Appl. Mater. Interfaces, 2017, 9, 41066–41077 CrossRef CAS PubMed.
- Y. Wang and D. Zhao, Cryst. Growth Des., 2017, 17, 2291–2308 CrossRef CAS.
- P. Nugent, Y. Belmabkhout, S. D. Burd, A. J. Cairns, R. Luebke, K. Forrest, T. Pham, S. Q. Ma, B. Space, L. Wojtas, M. Eddaoudi and M. J. Zaworotko, Nature, 2013, 495, 80–84 CrossRef CAS PubMed.
- K. Li, D. H. Olson, J. Seidel, T. J. Emge, H. Gong, H. Zeng and J. Li, J. Am. Chem. Soc., 2009, 131, 10368–10369 CrossRef CAS PubMed.
- D. Tanaka, K. Nakagawa, M. Higuchi, S. Horike, Y. Kubota, T. C. Kobayashi, M. Takata and S. Kitagawa, Angew. Chem., Int. Ed., 2008, 47, 3914–3918 CrossRef CAS PubMed.
- A. M. Kałuża, S. Mukherjee, S.-Q. Wang, D. J. O’Hearn and M. J. Zaworotko, Chem. Commun., 2020, 56, 1940–1943 RSC.
- F. Millange, C. Serre, N. Guillou, G. Ferey and R. I. Walton, Angew. Chem., Int. Ed., 2008, 47, 4100–4105 CrossRef CAS PubMed.
- S. Choi, J. H. Drese and C. W. Jones, ChemSusChem, 2009, 2, 796–854 CrossRef CAS PubMed.
- P. Wang, T. Kajiwara, K.-I. Otake, M.-S. Yao, H. Ashitani, Y. Kubota and S. Kitagawa, ACS Appl. Mater. Interfaces, 2021, 13, 52144–52151 CrossRef CAS PubMed.
- M.-H. Yu, B. Space, D. Franz, W. Zhou, C. He, L. Li, R. Krishna, Z. Chang, W. Li, T.-L. Hu and X.-H. Bu, J. Am. Chem. Soc., 2019, 141, 17703–17712 CrossRef CAS PubMed.
-
Y. He, W. Zhou and B. Chen. Current Status of Porous Metal-Organic Frameworks for Methane Storage, Metal–Organic Frameworks: Applications in Separations and Catalysis Wiley-VCH, 2018, pp. 163–198 Search PubMed.
Footnote |
† Electronic supplementary information (ESI) available: Synchrotron PXRD patterns, water vapour sorption isotherms, TGA curves, Clausius–Clapeyron calculations, etc. CCDC 2106581–2106583. For ESI and crystallographic data in CIF or other electronic format see DOI: 10.1039/d1ma00785h |
|
This journal is © The Royal Society of Chemistry 2022 |