DOI:
10.1039/D2JA00089J
(Technical Note)
J. Anal. At. Spectrom., 2022,
37, 1600-1610
A precise and accurate analytical method for determination of osmium isotope ratios at the 1–15 pg level by using a MC-ICP-MS equipped with sparging introduction and high-sensitivity discrete dynode-type ion-counting detectors†
Received
16th March 2022
, Accepted 17th May 2022
First published on 20th June 2022
Abstract
Application of the rhenium (Re) and osmium (Os) isotope system is a useful way to date geological materials, trace their circulation, and reconstruct the Earth's surface environment. However, measurements on less than 15 pg of Os have been challenging because of the large contribution of Os from external sources and insufficient ion beam intensity of instruments. Here, we present a measurement procedure we developed for Re and Os isotope analyses that minimizes contamination of Os from external sources and enables measurements on less than 15 pg of Os with high accuracy and precision by using a multiple collector-inductively coupled plasma-mass spectrometer equipped with compact discrete dynode (CDD) ion-counting detectors. The cleaning procedure we developed for laboratory equipment reduced the amount of Os from external sources to 0.002–0.015 pg, one order of magnitude lower than previously reported values. The measurements of Os isotope standard samples containing less than 30 pg of Os by the use of CDD detectors with in-run cross-calibration of differences in counting efficiencies of CDD detectors produced data with much better precision than those produced by using Faraday cup detectors. The measurement results of geological reference materials, JMS-2 and JCh-1, demonstrated that our method using CDD detectors yielded geochemical data with in-run precision that were equivalent to or better than those obtained by conventional procedures. The method enabled reduction of the sample volume and thus enabled high-spatial-resolution analysis with a small amount of the geological sample.
1. Introduction
The rhenium (Re) and osmium (Os) isotope system has been used as a unique and useful tool for diverse types of geochemical investigations. Radiometric dating is one of the most common applications of the Re–Os isotope system and uses the radioactive decay of 187Re to 187Os with a half-life of 4.23 × 1010 years. Because Re and Os are siderophile and chalcophile elements that are concentrated in sulfide minerals such as pyrite and chalcopyrite, the Re–Os isotope system has been used for precise dating of sulfide deposits. The initial value of the 187Os/188Os isotope ratio and the 187Os/188Os ratio in the minerals has been used to determine the sources of the minerals.1–5 The Re–Os isotope system has also been used for dating organic-rich geological materials such as black shale and crude oil, which concentrate Re at the ng g−1 level,6–9 and other Re–Os-rich geological materials such as iron meteorites.10,11 The 187Os/188Os ratio, which varies widely in the Earth's reservoirs, is also a versatile geochemical tracer. The 187Os/188Os ratio in seawater has fluctuated throughout the Earth's history and reflects changes in the balance between the influxes of terrigenous components with radiogenic Os and hydrothermal and extraterrestrial components with unradiogenic Os.12 The record of 187Os/188Os ratios preserved in deep-sea sediments has therefore been used for reconstructing global environmental changes on the Earth's surface. Deep-sea biogenic carbonate sediments could provide a Cenozoic record of 187Os/188Os fluctuations with high temporal resolution and highly reliable ages.13–15 Lithified, siliceous deep-sea sediment (chert) and metalliferous sediment (umber) in accretionary complexes have been used to obtain the marine 187Os/188Os record before the Cenozoic.16–20 The long-term fluctuations of the marine 187Os/188Os record may also be used to date deep-sea deposits that lack age-diagnostic proxies by fitting measured isotope ratios with a reconstructed marine secular 187Os/188Os curve.21–25 Moreover, stable 187Os/188Os ratios in extraterrestrial materials often mark apparent negative excursions of the 187Os/188Os record that allow detection of past meteorite impact events.26–29
Measurement techniques have been developed and improved to facilitate the use of a variety of geochemical applications of the Re–Os system. The improvements have focused on sample digestion methods that use mixtures of acids and procedures for introducing samples into instruments. A combination of sample digestion with a mixture of HNO3 and HCl (inverse aqua regia, HNO3
:
HCl = 3
:
1), a method for Os introduction into the instrument by argon (Ar)-gas bubbling in a sample solution (sparging introduction), and the use of a multiple collector-inductively coupled plasma-mass spectrometer (MC-ICP-MS) equipped with a multiple ion-counting (MIC) system and/or Faraday cup (FC) detectors has been used successfully to determine Os isotope ratios of samples containing small amounts of Os (∼15 pg) with low blanks (<1 pg) and high precision.30–34 In this technique, powdered samples are digested with inverse aqua regia in a sealed glass tube (a Carius tube) to prevent any leakage of volatile Os tetroxide (OsO4) during digestion at high temperatures (>200 °C).35 The sparging introduction makes it possible to achieve chemical separation (purification) while introducing Os into the ICP glass torch by using the highly volatile nature of the OsO4 molecule even at room temperature. The Os ionized by ICP is then detected by MIC and/or FC detectors. The signal is enhanced via a secondary electron multiplier (SEM) or high-resistivity amplifiers with 1011–1013 Ω resistors.33,34 Despite these improvements of Os isotope ratio measurements by MC-ICP-MS, precise determination of Os concentrations and isotope ratios from very small amount of Os (<15 pg) has still been challenging. Os is one of the rarest elements on the Earth's surface and less than 15 pg g−1 Os in geological materials is quite common. There have been two main problems associated with the measurements of very small amounts of Os: (1) contamination by high amounts of Os of external origins and (2) an ion beam intensity that is insufficient to obtain highly precise data with FC detectors. To correct for contamination by Os from the laboratory environment and reagents, several procedural blank samples have usually been analyzed together with actual samples, and the amount of Os in the blank samples has been subtracted from Os in the actual samples. However, the fact that a very small amount of Os in the actual sample necessarily increases the fraction of contaminant Os reduces the accuracy of the measurements. It has therefore been important to minimize the contribution of Os from the laboratory environment and reagents to improve the uncertainty of the measurement results.
The MIC system has often been used to overcome the problem associated with an insufficient Os ion beam intensity. Ion-counting detectors can enable highly sensitive measurements, but there have been difficulties associated with obtaining highly accurate and highly precise data because of the low stability of the ion-counting detectors. Corrections must be made for differences in instrumental mass bias effects and counting efficiencies between the several detectors that are used for calculating isotope ratios to obtain true isotope ratios with MIC systems.33 To make these corrections, a sample-calibrator bracketing method using standard samples has often been used, but this method cannot consider fluctuations of instrument conditions during a single run. Moreover, ion intensity measured by the MIC system with a conventional Channeltron-type SEM is much less stable than that measured with the FC detector, and a Channeltron-type SEM detector has a shorter lifetime than the FC detector. The large changes of the counting efficiency of the Channeltron-type SEM during even a single run cause a drift of the isotope ratio by ∼3%.33 The sample-calibrator bracketing method using Channeltron-type SEM detectors not only is time consuming but also increases the measurement uncertainty of the data obtained for the blank and for samples with a low amount of Os.
A discrete dynode-type SEM detector that was optimized to fit a multiple-collector array of a mass spectrometer has recently been equipped with a Thermo Fisher Scientific Neptune Plus mass spectrometer.36 This compact discrete dynode (CDD) SEM is a newly developed ion-counting device that achieves both high stability and high linearity over a large dynamic range.36 The much thinner width (6–7 mm) of the CDD SEM versus the classical discrete dynode SEM (∼20 mm) makes possible a collector array arrangement that collects ion beams with one mass difference. Here, we report the results of the development of an accurate, precise, and rapid analytical method for determination of Os isotope ratios using the sparging method and CDD detectors for analyses of samples with very small amounts of Os (<15 pg).
2. Experimental
Every experiment described below was conducted in a laboratory for analysis of platinum group elements at the Ocean Resources Research Center for Next Generation (ORCeNG) of the Chiba Institute of Technology (CIT), Japan.
2.1. Reagents
We used deionized water (electrical resistivity > 18.2 MΩ cm) produced by using a Millipore Milli-Q® system (Merck KGaA, Germany) throughout our experiments, including the cleaning of laboratory equipment and sample preparations. To clean laboratory equipment, we used electronic (EL)-grade HNO3 (61% m m−1, Kanto Chemical Co., Inc., Japan) and EL-grade HCl (36% m m−1 Kanto Chemical Co., Inc., Japan). HNO3 used for the sample digestion by inverse aqua regia was prepared from the EL-grade HNO3 by boiling at 190 °C in a clean-air chamber until the liquid volume had been reduced by 25% to remove dissolved OsO4 and minimize contamination by Os from external sources.37 We used concentrated HNO3 (∼60% m m−1) for chemical separation of Re (described below) and concentrated HCl (∼30% m m−1) throughout our experiments. These reagents were prepared by double distillation from the EL-grade reagents with a CleanAcids® (Analab, France) sub-boiling-type distilling purification apparatus. The concentrations of the distilled reagents were determined from their density, which we measured by weighing exactly 10 mL of reagents. The reagents were then diluted with deionized water to a concentration suitable for each experimental step. Notably, HNO3 prepared by distillation was not used for the acid digestion of powdered samples because we found that its use resulted in a relatively high Os blank due to contamination by external-origin Os during the distillation process.
2.2. Laboratory equipment
To clean the Carius tubes and PFA equipment, we prepared 50% m m−1 inverse aqua regia from EL-grade HNO3, EL-grade HCl, and deionized water (HNO3
:
HCl
:
water = 3
:
1
:
4). The borosilicate-glass Carius tubes used for sample digestion in this study were soaked in diluted inverse aqua regia at ∼120 °C for 24 hours and then in deionized water at ∼90 °C for another 24 hours. Finally, they were dried at 80 °C in a clean oven. We used 22- and 30 mL PFA vials as containers for the digested sample solutions. The PFA vial caps and PFA tubes used in the sparging introduction system were cleaned in several steps as follows: (i) ultrasonic cleaning in hot (∼80 °C) alkaline wash solution (TMSC: tetramethylammonium hydroxide solution, Tama Chemicals Co., Ltd.) that was diluted with deionized water to ∼5% m m−1 for 30 minutes and then soaked in diluted TMSC solution for 24 hours, (ii) soaking in diluted inverse aqua regia at ∼120 °C for 24 hours, and (iii) soaking in deionized water at ∼90 °C for 24 hours. The PFA vials were further cleaned by additional steps to minimize the amount of any external-origin Os that had been incorporated into the molecular structure of the PFA polymer as follows: (iv) adding ∼5 mL of EL-grade HNO3 to each vial and heating the vials at 190 °C in a clean-air chamber until the liquid volume was reduced to almost half, (v) removing the remaining HNO3 and washing the vials three times with deionized water, (vi) filling the vials with deionized water and heating them at ∼90 °C for 24 hours with the caps closed, and (vi) removing deionized water and drying the vials at 80 °C for 24 hours in a clean oven. Note that the vials were rinsed with deionized water between each cleaning step. These additional steps allowed Os incorporated into the PFA polymer to be completely oxidized and evaporated as volatile OsO4 molecules during the treatment with HNO3 at 190 °C.
2.3. Samples
The Os isotope standard solution used to make the Os standard samples was prepared and diluted to ∼10 ng g−1 from a chemical standard solution (Alfa Aesar 1000 μg g−1 Os ICP standard solution) produced from the material from the Johnson Matthey Company (London, United Kingdom). The Re standard solution was prepared from a chemical standard solution (Alfa Aesar 1000 μg g−1 Re ICP standard solution). Powdered samples of the geological reference materials of pelagic brown clay (JMS-2) collected from Penrhyn Basin, South Pacific,38 and chert (JCh-1) collected from the Ashio Belt, Tochigi Prefecture, Japan39 were provided by the Geological Survey of Japan (National Institute of Advanced Industrial Science and Technology, Japan) and were used for analysis of Re and Os concentrations, and isotope ratios. The powdered samples were dried at 110 °C in an oven for at least 24 hours before weighing.
2.4. Sample preparation
The sample preparation method used in this study followed the methods previously reported33,34 with some modifications. The whole procedures used in this study are described in the ESI.† In our procedure, ∼0.05 g of JMS-2 (∼15 pg Os) and ∼1.0 g of JCh-1 (∼5 pg Os) were weighed for isotopic measurements using CDD detectors, and ∼1.0 g of JMS-2 (∼300 pg Os) and ∼2.0 g of JCh-1 (∼10 pg Os) were weighed for isotopic measurements using FC detectors. We also oxidized the Os standard solution with inverse aqua regia to prepare the Os standard samples. After the Os isotope measurements, Re was purified in two-step column separation using an anion exchange resin (Muromac AG1-X8 100–200 mesh) as previously described.33,40 15 μL of a 100 ng g−1 iridium (Ir) standard solution traceable to a chemical standard solution (AccuStandard 1000 μg g−1 Ir ICP standard solution) was added into the final sample solution to adjust the Ir concentration to ∼1.5 ng g−1. The measured ratio of 193Ir/191Ir was used to make an external correction for mass bias effects during the measurement described in Section 2.5.2. In addition to the sample solutions, Re–Ir mixed standard solutions were prepared from the Re and Ir standard solutions.
2.5. Settings and configuration of the MC-ICP-MS
2.5.1. Os isotope ratio measurements.
The Os isotope ratios were measured with a MC-ICP-MS (Neptune Plus; Thermo Fisher Scientific, Waltham, MA, United States) combined with sparging introduction30–34 at the ORCeNG of the CIT. Table S1† summarizes the experimental settings and detector configurations. The caps on the 22- or 30 mL PFA vials containing samples or Os standard samples were replaced with PFA transfer caps. The vials were then inserted into the argon (Ar) sample gas line of the MC-ICP-MS instrument. The PFA transfer caps had two transfer ports: (1) one side was penetrated with a 1/8-inch PFA tube that was connected to the Ar sample gas outlet from the instrument with a Tygon tube; (2) the other side was free and connected to a quartz injector through an empty 120 mL PFA vessel that served as a buffer of fluctuations of the sample gas flux.33,41 The operating conditions were finely tuned at the beginning of every measurement to maximize the intensity of 192Os in sub-configuration 1 (CDD measurement) by using the Os standard samples containing 20 pg (CDD measurement) (192Os; ∼200
000 cps, peak intensity just after introducing Os with sample gas) or 100 pg (FC measurement) (192Os; ∼20 mV, peak intensity just after introducing Os with sample gas) of the total Os. By tuning every measurement, we maximized the Os ion intensity when the Ar sample gas flow rate was ∼1.1 L min−1. For measurement with FC detectors, we used a normal Ni sampling cone and Ni X skimmer cone to maximize the sensitivity, but the normal Ni sampling cone and Ni H skimmer cone were used for measurements with CDD detectors. Because the positions of the CDD detectors were fixed and could not be changed for each sub-configuration during the CDD measurements, the centering of ion-beams to the CDD detectors for each sub-configuration was achieved by adjusting the ion beam spacing with a dispersion quadrupole ion lens. However, in this case we found that a flat peak could not be achieved with the X skimmer cone, even if the ion beam focus was finely adjusted by using a focus quadrupole ion lens. We therefore used an H skimmer cone to both center the ion beam and achieve a flat peak.
During the measurements with CDD detectors, the Os ion intensities were measured with three CDD detectors (IC4, IC5, and IC6) (Table S1†). The configuration allowed us to measure ion intensities at m/z 187 (187Re+ and 187Os+), 188 (188Os+), 190 (190Os+), and 192 (192Os+). We also measured the ion intensity at m/z 185 (185Re+) to monitor isobaric interference of 187Os by 187Re. To obtain accurate isotope ratios, we used an in-run cross-calibration of the differences of the counting efficiencies between the CDD detectors. During a single cycle of run, each detector measured the 190Os+ ion intensity in the corresponding sub-configuration (Table S1†) in addition to the main sub-configuration measurement of 185Re+, 187Os+, and 188Os+ (sub-configuration 4). We used sub-configuration 1 to also measure 192Os+ for the ID calculation and to correct for mass bias effects. The differences of counting efficiencies were expressed as ratios of 190Os+ ion intensities measured by using each CDD detector:
|  | (1) |
|  | (2) |
|  | (3) |
where
Ri/j is the ratio of the counting efficiency of detector
i to detector
j, and

is the ion intensity of
190Os measured with detector
i. The raw isotope ratios measured in this study (
185Re/
188Os,
187Os/
188Os,
192Os/
188Os, and
190Os/
192Os) were corrected for the counting efficiency as follows:
| 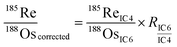 | (4) |
| 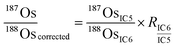 | (5) |
| 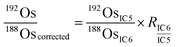 | (6) |
| 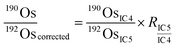 | (7) |
During the MC-ICP-MS measurements with sparging introduction, the beam intensity quickly increased when the Ar sample gas was introduced into the sample solution, and after reaching the peak it decreased exponentially with time due to the decrease of the amount of Os contained in the sample solution (Fig. S1†). The Os isotope data were recorded during the exponential decrease of the Os ion intensities in a single run. The decrease of intensity with time caused differences in 190Os intensities measured in sub-configurations 1, 2, and 3, which made it impossible to adequately correct differences of the counting efficiencies by these calculations. A quadratic drift correction (Thermo Fisher Scientific, NEPTUNE Plus Operating Manual) was applied in advance to these calculations by using the Neptune Plus software, so that the 190Os intensity difference between each sub-configuration due to the intensity decrease was adequately corrected. The effect of the instrumental mass bias on the 187Os/188Os and 190Os/192Os ratios was then corrected by normalizing with 192Os/188Os = 3.08271 (ref. 42) based on the exponential law. The use of these correction procedures enabled us to obtain Os isotope ratio data without any systematic drift during a single run of 50 cycles (Fig. S2†).
To measure geological reference materials (JMS-2 and JCh-1), on-peak backgrounds were measured before the actual sample measurements while only sample Ar gas was flowing to correct for the Os isotopes in the Ar gas and the memory effect. Five procedural blank samples were also measured, and the average amount of each Os isotope was subtracted from the corresponding Os amount in the geological reference materials to correct for the external-origin Os. For measurements of the Os standard samples, instead of subtracting Os isotopes in the Ar sample gas and the procedural blank samples, diluted inverse aqua regia without the Os standard solution that was used to dilute the Os standard samples was initially measured, and the average intensities of each isotope were subtracted from those of the Os standard samples. The 185Re/188Os ratio obtained from eqn (4) could be used for the isobaric interference correction of 187Re on 187Os, but it was not applied in this study because 185Re was not detected in our measurements (Fig. S1:† the average 185Re/188Os was usually almost zero within the uncertainty of the measurement).
During the measurements with FC detectors, ion intensities at m/z 183 (183W+), 185 (185Re+), 186 (186Os+), 187 (187Re+ and 187Os+), 188 (188Os+), 189 (189Os+), 190 (190Os+), and 192 (192Os+) were measured by using each FC detector (L4, L3, L2, L1, axial, H1, H2, and H3) (Table S1†). The on-peak backgrounds were measured before sample measurements for both geological reference materials and Os standard solutions. The instrumental mass bias effect on each Os isotope ratio (186Os/188Os, 187Os/188Os, 189Os/188Os, and 190Os/188Os) was corrected by normalizing with 192Os/188Os = 3.08271 (ref. 42) based on the exponential law.
2.5.2. Re measurements.
Re and Ir isotope ratios were also measured with the MC-ICP-MS (NEPTUNE Plus) at the ORCeNG of the CIT. Sample solutions were introduced by normal procedures using a self-aspirating PFA nebulizer (MicroFlow PFA-100, Elemental Scientific, USA) and a glass spray-chamber (SSI Quartz Dual Cyclonic Spray Chamber, Elemental Scientific, USA) to avoid any unexpected mass bias effect associated with the use of a desolvent nebulizer such as Aridus II.43 The operating conditions were finely tuned with a Re–Ir mixed standard solution containing 1.5 ng g−1 Re and Ir at the beginning of daily measurements to maximize the intensity of 187Re and 193Ir on the L3 and H2 FC detectors. After the fine tuning, the Ar sample gas flow rate was usually regulated to ∼1.1 L min−1. The intensities of the Re and Ir isotopes were measured with FC detectors (L4, L3, C, and H2) using the detector configuration summarized in Table S1,† and the intensities of three Os isotopes (189Os, 190Os, and 192Os) were also measured to monitor any isobaric interference of 187Os on 187Re. Because Re has only two naturally occurring isotopes (185Re and 187Re), a correction for the instrumental mass bias effect on the Re isotope ratio (187Re/185Re) was made with an external correction method based on the exponential law. The Ir standard solution was added to the sample solutions in advance. We assumed the natural 187Re/185Re and 193Ir/191Ir isotope ratios to be 1.6738 and 1.68097,44 respectively.
3. Results and discussion
3.1. Stability of the CDD detectors
We checked the stability of the CDD detectors by introducing a solution of uranium (U). The concentration of U in the solution was adjusted so that the intensity of 238U measured by each CDD detector was ∼300
000 cps. The plateau calibration (tuning of the operational voltage) for each CDD detector was finely tuned to make the ratios of the detection efficiencies of the CDD detectors ∼1. During nine runs of 50 cycles each (a total of 450 cycles) with 8 seconds of acquisition time, the ratios of 238U intensities obtained by using each CDD detector—the ratios of the counting efficiencies of pairs of CDD detectors (IC5/IC4, IC6/IC4, and IC6/IC5)—showed short-term (1 to several cycles) fluctuations (Fig. S3†) with a typical standard deviation (S.D.) in a single run (50 cycles) of 0.3–0.7%. However, the fact that the drifts during a single run (50 cycles) and a whole measurement (450 cycles) were much smaller than those obtained with an MIC system with a Channeltron-type SEM33 demonstrated the much higher stability of CDD detectors than MIC detectors with a Channeltron-type SEM. We therefore expected that the use of the 190Os intensity for in-run cross-calibration of the difference in counting efficiencies between pairs of CDD detectors would be an effective way to correct for the short-term fluctuations of the CDD detector efficiencies.
3.2. Os standard samples
The Os standard samples containing 1, 2, 5, 10, 20, and 30 pg of Os were measured with CDD detectors, and those containing 30, 50, 100, and 1000 pg of Os were measured with FC detectors. Table S2† and Fig. 1 summarize the results. All the 187Os/188Os ratios obtained with both the CDD and FC detectors were consistent with within 1 S.D. of the JMC Os standard value of 0.106838 ± 0.00015 (2S.D.) determined by negative thermal ionization mass spectrometry (N-TIMS)33 (Fig. 1). The 2R.S.D. (relative standard deviation) values in runs of JMC Os standard samples containing 1, 2, 5, 10, 20, and 30 pg of Os measured with CDD detectors were ∼7, ∼4, ∼3, ∼2, ∼1, and ∼1%, respectively. Those containing 30, 50, 100, and 1000 pg Os measured with FC detectors were ∼7, ∼4, ∼2, and 0.2%, respectively. Based on these data, the 2R.S.D. values obtained with the CDD and FC detectors could be approximated with eqn (8):34
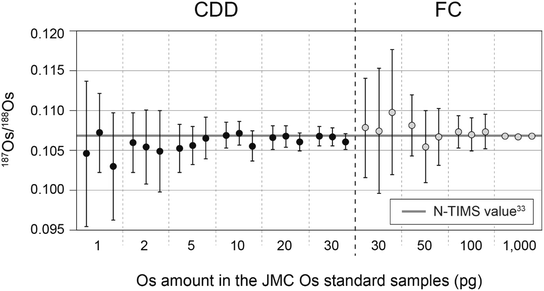 |
| Fig. 1 Results of the JMC Os standard samples with various amounts of Os measured with CDD and FC detectors. The error bars indicate 1 S.D. Error bars are smaller than the plot symbols when the samples contained 1000 pg of total Os and FC detectors were used. | |
In eqn (8), MOs is the amount of Os (pg) in a standard sample. In this study, A and B were estimated to be 6.18 and −0.546, respectively, for measurements with CDD detectors (Fig. 2), and 182 and −0.984 for FC detectors (Fig. 2). The predictions of eqn (11) indicated that measurements with CDD detectors could produce more precise data than measurements with FC detectors for all amounts of Os contained in the standard samples. However, the amount of Os in a standard sample is limited to no more than ∼80 pg, which is equivalent to an intensity of ∼800
000 cps for 192Os. The reason is that a higher intensity exceeds the certified linearity range of CDD detectors (<800
000 cps (ref. 36)) and may shorten the lifetime of the CDD SEM. Moreover, the achievable precision (2R.S.D.) for the 187Os/188Os ratio predicted that the precision expressed as 2R.S.D. for a standard sample containing 80 pg Os would be ∼0.6%, which is not much better than that of a standard sample containing 30 pg Os (∼1%). We therefore suggest that the amount of Os in a sample solution for a CDD measurement should be kept below ∼30 pg to ensure the linearity of the CDD detectors and to avoid potential damage to them. In contrast, FC detectors could accommodate a much wider range of intensities of an introduced ion—up to 50 V (1 mV = 62
500 cps)—and thus they could produce data with better precision than CDD detectors with amounts of Os as high as ng levels.34 In measurements using FC detectors with 1012 Ω amplifiers, ∼200 pg of Os would be associated with the same 2R.S.D. value as the measurement of 30 pg of Os with CDD detectors (Fig. 2). These results suggest that the following is the best way to choose detector types from the standpoint of precision: CDD detectors are preferred when the amount of Os is sure to be less than 30 pg. If a sample volume or its Os concentration is high enough to ensure that the sample contains 200 pg or more of Os, FC detectors with 1012 Ω amplifiers should be used because they will produce results with higher precision than CDD detectors. Another option is to use a CDD detector for the measurement but reduce the sample volume so that the equivalent amount of Os is less than 30 pg; this strategy can produce a high-precision measurement and reduce the amount of the sample consumed.
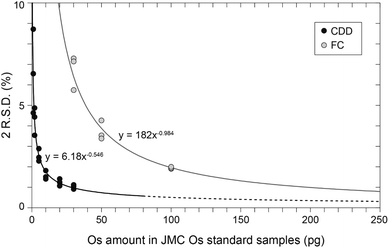 |
| Fig. 2 Achievable 2R.S.D values of 187Os/188Os at different amounts of Os in JMC Os standard samples measured by using CDD and FC detectors. The dotted line represents extrapolation for CDD measurements, but it cannot be achieved due to the limitation of the ion intensity for CDD detectors (∼800 000 cps) (see Section 3.2). | |
3.3. Procedural Os blank
The five procedural blank samples of Os analyzed together with the geological reference materials contained 0.002–0.015 pg (mean = 0.005, 2S.D. = 0.008, n = 5) (Table S3†). The mean value of these Os blanks was one order of magnitude lower than previously reported values (0.07–0.69 pg (ref. 20, 28 and 33)) that were measured via MC-ICP-MS combined with Carius tube digestion with inverse aqua regia and sparging introduction (Fig. 3). The analytical procedures used in this study were almost identical to the procedures used in these previous studies: we used the same amount of inverse aqua regia (4 mL) and the same heating temperature and time,20,28,33 and the specifications of the laboratory equipment (draft chamber, sample drying chamber, oven, etc.) at the ORCeNG of the CIT were similar to those used at the Japan Agency for Marine-Earth Science and Technology (JAMSTEC), where the previous analyses were conducted. One exception was the purification of HNO3 by heating at 190 °C in a clean-air chamber, which was prepared for the sample digestion. This procedure, which was modified from the previously reported procedure,45 was not employed at JAMSTEC about ten years ago,28,33 whose procedural Os blank values were ∼0.7 pg. The procedures used in the most recent study conducted at JAMSTEC20 included purification of HNO3; the procedural Os blanks reported in this study were reduced to ∼0.1 pg (Fig. 3). Another exception was the procedure used to clean the PFA vials, which was added as a further cleaning step and involved the use of HNO3 heated to 190 °C (see Section 2.2). To document the cleanliness of the experimental environment in the laboratory at the ORCeNG of the CIT, we recorded the results of measurements of procedural blank samples. Fig. S4† shows the secular record of analyses of procedural blank samples that were analyzed by using various operators for various lithological types of samples from May 2019 to December 2021. Notably, the amounts of Os in the first four blank samples, for which there was no further cleaning of the PFA vials, were 0.1–0.5 pg and therefore similar to the values reported in previous studies.27,33 The typical Os blank value at JAMSTEC by sparging introduction and MC-ICP-MS is now ∼0.2 pg. The amount of Os in the blank samples with the further cleaning step was less than 0.1 pg, with the exception of three outliers, even though the PFA vials were washed after each experiment and used repeatedly. The reason why the values of the blank samples sometimes exceeded 0.1 pg was probably the result of some technical errors (contamination by airborne particles, sample powders, etc.) made by using inadequately trained operators. This record suggested that further cleaning of the PFA vials was effective in removing Os that remained in the PFA polymer, thereby reducing Os contamination of the experimental environment. When the Os blank is the typical value (0.002–0.015 pg) obtained with our method, the blank contribution can be kept below ∼1.5%, even if the amount of Os in the sample is only ∼1 pg.
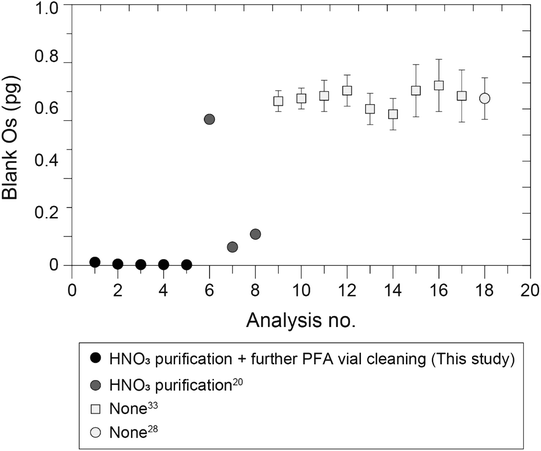 |
| Fig. 3 Amounts of Os in procedural blank samples of this study compared with previous studies20,28,33 analyzed by sparging introduction and MC-ICP-MS. This study employed both the HNO3 purification and the further PFA cleaning, and one of the previous studies employed only HNO3 purification20 and the other employed neither.28,33 The error bars indicate 2S.E. (this study) and 2S.D values.20,33 Data indicated as a light gray circle28 is the average of nine blank samples with an error bar equal to 2S.D.28 | |
3.4. Re standard solution
Twenty measurements were performed in the Re–Ir mixed standard solution containing 1.5 ng g−1 of Re and Ir with the assumed natural 193Ir/191Ir ratio of 1.68097.44 The mean of the 187Re/185Re ratios measured with mass bias correction performed by the external correction method was 1.6673 ± 0.0005 (mean ± 2S.D., n = 20), which was significantly lower than the assumed natural 187Re/185Re ratio of 1.6738 (ref. 44) (Fig. S5†). The mean 187Re/185Re ratio corrected by the external correction method was −3.9‰ difference in the δ187Re value46 relative to the assumed natural ratio, which was significantly larger fractionation than the reported isotopic fractionation of various isotope standard materials up to 0.3‰.46 This inferred that the external correction method was not appropriate. We therefore applied the correction method using empirical calibration of the mass bias coefficients for Re and Ir.46,47 The ratio of isotopes i and j (R) corrected for the mass bias effect is calculated with the mass bias coefficient β and eqn (9): | 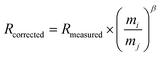 | (9) |
where mi and mj are the atomic weights of isotopes i and j, respectively. The external correction method for the mass bias effect on 187Re/185Re assumed that β values for 187Re/185Re and 193Ir/191Ir were identical. However, it has been pointed out that β values are not identical among different elements, such as Tl and Pb.48 It has been suggested that the relationship between β values of one element and another element should be expressed by a straight line such as eqn (10):47,48
In eqn (10), A and B are constant values, and βi and βj are the β values of elements i and j. It has been known that this relationship will not change, even if the tuning parameters such as the sample Ar gas flow rate, element concentration of sample solutions, and sampling and skimmer cones are changed.48 Moreover, it has been reported that the constant A is approximately 1.47 To confirm this relationship, we have recorded β values of 187Re/185Re and 193Ir/191Ir 273 times over three years with various instrument settings. A scattering diagram of recorded β values of 187Re/185Re and 193Ir/191Ir clearly showed a good linear relationship, a positive correlation, and a parallel shift towards higher β values of 187Re/185Re than 193Ir/191Ir (Fig. S6†). Assuming that this relationship between β values of 87Re/185Re and 193Ir/191Ir could be described by using eqn (10), a geometric mean regression yielded the constant A and intercept B as 0.995 and 0.348, respectively (Fig. S6†). Therefore, we assumed that the constant A was 1 and then the intercept B was calculated to be 0.355. Based on the above investigation, we corrected β values of 187Re/185Re with eqn (11).
| β187Re/185Re = β193Ir/191Ir + Bday | (11) |
In eqn (11), Bday was determined by measuring the Re–Ir mixed standard solution on the day of measurement from the natural values of 187Re/185Re and 193Ir/191Ir, and then the true β values of the sample 187Re/185Re ratios were calculated by using the determined Bday and eqn (11). To test the accuracy of this method, we corrected the 187Re/185Re ratios obtained from the 20 measurements of the Re–Ir mixed standard solution. The mean corrected 187Re/185Re value of these 20 measurements was 1.6738 ± 0.0005 (2S.D.), which was within 2S.D. of the assumed natural value (Fig. S5†). We therefore used this method to correct the isotope ratios of the actual samples.
3.5. Geological reference materials
We used both CDD and FC detectors to measure Re–Os isotope ratios and determined the concentrations of geological reference materials to compare the data as an example of the application of measurements with CDD detectors to those of the actual samples. For the measurements with FC detectors, we used ∼1 g of the JMS-2 sample of pelagic brown clay and ∼2 g of the JCh-1 sample of chert. These masses were equivalent to ∼300 pg and ∼10 pg of Os, respectively. For the measurements with CDD detectors, these masses were reduced to ∼0.05 g and ∼1 g, respectively. These weights were equivalent to ∼15 pg and ∼5 pg of Os, respectively.
Table S3† summarizes the Re and Os concentrations and the 187Os/188Os and 187Re/188Os isotope ratios determined in this study, and Fig. 4 compares these results to the Os concentrations and 187Os/188Os isotope ratios obtained in previous studies.28,33,34,49 The mean Os concentrations of JMS-2 determined by using CDD detectors and FC detectors were 305 ± 25 pg g−1 (n = 5, 2S.D.) with an in-run precision of 0.15–0.21% (2S.E.) and 338 ± 76 pg g−1 (n = 5, 2S.D.) with an in-run precision of 0.01–0.02% (2S.E.), respectively. The mean Os concentrations of JCh-1 determined by using CDD and FC detectors were 5.6 ± 1.7 pg g−1 (n = 5, 2S.D.) with an in-run precision of 0.17–0.30% (2S.E.) and 5.6 ± 0.4 pg g−1 (n = 5, 2S.D.) with an in-run precision of 0.27–0.41% (2S.E.), respectively. The mean 187Os/188Os isotope ratios of JMS-2 determined by using CDD and FC detectors were 0.843 ± 0.064 (n = 5, 2S.D.) with an in-run precision of 0.20–0.32% (2S.E.) and 0.790 ± 0.156 (n = 5, 2S.D.) with an in-run precision of 0.02–0.03% (2S.E.), respectively. The mean 187Os/188Os isotope ratios of JCh-1 determined by using CDD and FC detectors were 0.589 ± 0.115 (n = 5, 2S.D.) with an in-run precision of 0.24–0.49% (2 S.E.) and 0.585 ± 0.035 (n = 5, 2 S.D.) with an in-run precision of 0.38–0.54% (2 S.E.), respectively.
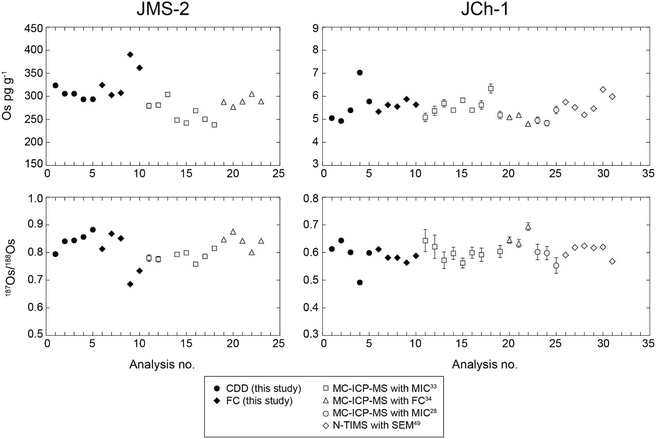 |
| Fig. 4 Results of Os concentrations and 187Os/188Os ratios for samples of geological reference materials (JMS-2 and JCh-1). The error bars indicate 2S.E. (this study and ref. 34 and 49) and 2S.D values.28,33 | |
The Os concentrations and 187Os/188Os isotope ratios of JMS-2 and JCh-1 determined by using CDD detectors and FC detectors generally were within the precision of each method. Even though the sizes of the JCh-1 samples for measurements with CDD detectors (∼1 g) were half of that for measurements with FC detectors (∼2 g), the in-run precision was better for the CDD detectors than that for the FC detectors (Table S3†). The in-run precision of JMS-2 data was one order of magnitude higher for measurements with FC detectors because the sample sizes were larger and contained ∼300 pg of Os. However, the use of the CDD detectors enabled a precise measurement result with 2 R.S.D. below 1% even for very small amounts of the JMS-2 samples (∼0.05 g). Our data agreed with those previously determined with an MC-ICP-MS equipped with a Channeltron-type SEM (MIC)28,33 and with FC detectors34 and via N-TIMS with pulse-counting, electron-multiplier mode and axial SEM49 (Fig. 4). Notably, the in-run precision of our data for JCh-1 (0.01–0.02 for Os concentrations and 0.001–0.003 for the 187Os/188Os ratio) obtained via CDD measurements was generally better than those obtained via N-TIMS with an axial SEM detector (0.02–0.04 for the Os concentration and 0.002–0.006 for the 187Os/188Os ratio).49 We also compared variations of our data with those from previous studies by calculating the precision (2R.S.D.) of data obtained with JMS-2 and JCh-1. For our data, the 2R.S.D. values of the Os concentrations of ten data of JMS-2 and JCh-1 were 19.7 and 20.5%, respectively, and those of the 187Os/188Os ratios of ten data of JMS-2 and JCh-1 were 15.4 and 13.7%, respectively. We calculated the precision (2R.S.D.) of the Os concentrations measured in previous studies of JMS-2 and JCh-1 to be 16.5% (n = 13)33,34 and 15.6% (n = 21),28,33,34,49 respectively. We calculated the 2R.S.D. values of the 187Os/188Os ratios measured using JMS-2 and JCh-1 to be 8.7% (n = 12)33,34 and 10.8% (n = 20),28,33,34,49 respectively. This comparison indicated that the variations of our data were slightly larger than those of the data reported in previous studies. The larger variation of the data in this study could be attributed to the heterogeneity of the Os distribution in the geological reference material itself (nugget effect), but they might also be attributed to the fact that sample heterogeneity would likely have a greater effect on the smaller samples used in this study (0.05–2 g) versus previous studies (1–3 g).
The data we obtained in this study indicated that measurement with CDD detectors could produce geochemical data with precision that were equal to or better than those obtained by conventional measurement procedures, even when the total amount of Os measured was much reduced (<30 pg Os). However, it should be noted that measurements with CDD detectors on only a small aliquot of the powdered sample from a much larger amount of the mother lithological sample are not always preferred because of the concern that sample heterogeneity may cause a small sample to not be representative of the much larger mother sample. In such cases, measurements with FC detectors on a large sample would still be preferred, and the use of FC detectors would also be preferred if the sample contained more than several hundred picograms of Os. The advantage of CDD detectors is that their use can improve the temporal and spatial resolutions of the Re–Os data of geological samples. For example, the use of a CDD detector makes it possible to obtain data with sufficient precision from a thin section of the sedimentary sequence or small region of the polycrystalline rock specimen that can yield only a few milligrams of the powdered sample.
4. Conclusions
We investigated a measurement procedure for measuring the concentrations and isotope ratios of Re and Os that could produce accurate and precise results for no more than 15 pg of Os by minimizing contamination from Os in the external environment and optimizing the measurement settings of CDD detectors. The amount of Os contamination from external sources could be kept at no more than 0.002–0.015 pg by addition of a step that involved cleaning PFA vials by heating them at 190 °C with HNO3 to remove Os incorporated into the PFA polymer. This step could reduce the percentage of external-origin Os to ∼1.5% when the amount of Os in the actual samples was ∼1 pg. We developed a highly sensitive analytical method for measuring Os isotope ratios that involved the use of CDD detectors and application of an in-line, cross-calibration method to correct for differences in the detection efficiencies of the CDD detectors. The use of CDD and FC detectors to analyze Os standard samples containing 1, 2, 5, 10, 20, 30, 50, 100, and 1000 pg of Os demonstrated that our cleaning step and the use of CDD detectors could produce data with much better precision than those obtained with FC detectors for amounts of Os less than 30 pg. The 2R.S.D. values of the results obtained with this method and CDD detectors indicated that the analytical precision achievable with CDD detectors was better than that obtained with FC detectors for any amount of Os contained in the samples, but the maximum amount of Os in samples for CDD detection should be kept below 30 pg to prevent exceeding the linearity range of the CDD SEM and potential damage thereto. Finally, measurement results of the geological reference materials, JMS-2 (pelagic brown clay) and JCh-1 (chert), confirmed that our method using CDD detectors produced Re–Os data with precision that were equivalent to or better than those obtained with an MC-ICP-MS equipped with FC detectors and conventionally used N-TIMS. Our results indicate that our method can reduce sample consumption, and thus achieve high spatial resolution via Re–Os analyses by limiting the quantity of the sample collected from geological samples to a small amount.
Author contributions
J. O., T. N., and Y. K. designed this study. J. O. and H. S. developed analytical methods for Re–Os isotope measurements. J. O. carried out Re–Os isotope analyses for the standard samples and the geological reference materials. K. A. developed the cleaning procedure for laboratory equipment. J. O. primarily wrote the manuscript with inputs from all co-authors.
Conflicts of interest
The authors declare no competing financial interests.
Acknowledgements
We thank H. Iida and N. Kanazawa of Thermo Fisher Scientific for helpful discussions and advice concerning the properties of MC-ICP-MS instruments. Discussion with Dr A. Ishikawa of Tokyo Institute of Technology was fruitful when building a chemical separation room for our laboratory. This study was financially supported by the Japan Society for the Promotion of Science through Grants-in-Aid for Scientific Research [grant numbers 19K14817 and 21K14011 to J. O. and 15H05771 and 20H05658 to Y. K.]
References
- Y. Kato, K. Suzuki, K. Nakamura, A. H. Hickman, M. Nedachi, M. Kusakabe, D. C. Bevacqua and H. Ohmoto, Earth Planet. Sci. Lett., 2009, 278, 40–49, DOI:10.1016/j.epsl.2008.11.021.
- T. Nozaki, Y. Kato and K. Suzuki, Geochim. Cosmochim. Acta, 2010, 74, 4322–4331, DOI:10.1016/j.gca.2010.04.055.
- T. Nozaki, Y. Kato and K. Suzuki, Sci. Rep., 2013, 3, 1889, DOI:10.1038/srep01889.
- T. Nozaki, Y. Kato and K. Suzuki, Econ. Geol., 2014, 109, 2023–2034, DOI:10.2113/econgeo.109.7.2023.
- N. J. Saintilan, D. Selby, R. A. Creaser and S. Dewaele, Sci. Rep., 2018, 8, 14946, DOI:10.1038/s41598-018-33399-7.
- D. Selby and R. A. Creaser, Geology, 2005, 33, 545–548, DOI:10.1130/G21324.1.
- D. Selby, R. A. Creaser and M. G. Fowler, Geochim. Cosmochim. Acta, 2007, 71, 378–386, DOI:10.1016/j.gca.2006.09.005.
- A. D. Rooney, D. Selby, J.-P. Houzay and P. R. Renne, Earth Planet. Sci. Lett., 2010, 289, 486–496, DOI:10.1016/j.epsl.2009.11.039.
- V. M. Cumming, D. Selby, P. G. Lillis and M. D. Lewan, Geochim. Cosmochim. Acta, 2014, 138, 32–56, DOI:10.1016/j.gca.2014.04.016.
- M. F. Horan, J. W. Morgan, R. J. Walker and J. N. Grossman, Science, 1992, 255, 1118–1121, DOI:10.1126/science.255.5048.1118.
- M. I. Smoliar, R. J. Walker and J. W. Morgan, Science, 1996, 271, 1099–1102, DOI:10.1126/science.271.5252.1099.
- B. Peucker-Ehrenbrink and G. Ravizza, Terra Nova, 2000, 12, 205–219, DOI:10.1046/j.1365-3121.2000.00295.x.
- G. Ravizza and B. Peucker-Ehrenbrink, Science, 2003, 302, 1392–1395, DOI:10.1126/science.1089209.
- R. van der Ploeg, D. Selby, M. J. Cramwinckel, Y. Li, S. M. Bohaty, J. J. Middelburg and A. Sluijs, Nat. Commun., 2018, 9, 2877, DOI:10.1038/s41467-018-05104-9.
- Y. Kuwahara, K. Yasukawa, K. Fujinaga, T. Nozaki, J. Ohta, H. Sato, J.-I. Kimura, K. Nakamura, Y. Yokoyama and Y. Kato, Sci. Rep., 2021, 11, 5695, DOI:10.1038/s41598-021-84448-7.
- Y. Kato, K. Fujinaga and K. Suzuki, Geochem., Geophys., Geosyst., 2005, 6, Q07004, DOI:10.1029/2005GC000920.
- Y. Kato, K. Fujinaga and K. Suzuki, Gondwana Res., 2011, 20, 594–607, DOI:10.1016/j.gr.2010.12.007.
- K. Fujinaga, K. Nakamura, J. Ohta, M. Yano, Y. Kuwahara, K. Yasukawa, Y. Takaya, K. Nakayama, T. Nozaki and Y. Kato, Ore Geol. Rev., 2022, 142, 104683, DOI:10.1016/j.oregeorev.2021.104683.
- T. Nozaki, T. Nikaido, T. Onoue, Y. Takaya, K. Sato, J.-I. Kimura, Q. Chang, D. Yamashita, H. Sato, K. Suzuki, Y. Kato and A. Matsuoka, J. Asian Earth Sci. X, 2019a, 1, 100004, DOI:10.1016/j.jaesx.2018.100004.
- Y. Tomimatsu, T. Nozaki, H. Sato, Y. Takaya, J.-I. Kimura, Q. Chang, H. Naraoka, M. Rigo and T. Onoue, Glob. Planet. Change, 2021, 197, 103387, DOI:10.1016/j.gloplacha.2020.103387.
- V. Klemm, S. Levasseur, M. Frank, J. R. Hein and A. N. Halliday, Earth Planet. Sci. Lett., 2005, 238, 42–48, DOI:10.1016/j.epsl.2005.07.016.
- V. Klemm, B. Reynolds, M. Frank, T. Pettke and A. N. Halliday, Earth Planet. Sci. Lett., 2007, 253, 57–66, DOI:10.1016/j.epsl.2006.10.018.
- S. G. Nielsen, S. Mar-Gerrison, A. Gannoun, D. LaRowe, V. Klemm, A. N. Halliday, K. W. Burton and J. R. Hein, Earth Planet. Sci. Lett., 2009, 278, 297–307, DOI:10.1016/j.epsl.2008.12.010.
- K. T. Goto, T. Nozaki, T. Toyofuku, A. H. Augustin, G. Shimoda, Q. Chang, J.-I. Kimura, K. Kameo, H. Kitazato and K. Suzuki, Deep Sea Res., Part II, 2017, 146, 82–92, DOI:10.1016/j.dsr2.2016.10.010.
- J. Ohta, K. Yasukawa, T. Nozaki, Y. Takaya, K. Mimura, K. Fujinaga, K. Nakamura, Y. Usui, J.-I. Kimura, Q. Chang and Y. Kato, Sci. Rep., 2020, 10, 9896, DOI:10.1038/s41598-020-66835-8.
- B. Peucker-Ehrenrink, G. Ravizza and A. W. Hofmann, Earth Planet. Sci. Lett., 1995, 130, 155–167, DOI:10.1016/0012-821X(95)00003-U.
- F. S. Paquay, G. E. Ravizza, T. K. Dalai and B. Peucker-Ehrenbrink, Science, 2008, 320, 214–218, DOI:10.1126/science.1152860.
- H. Sato, T. Onoue, T. Nozaki and K. Suzuki, Nat. Commun., 2013, 4, 2455, DOI:10.1038/ncomms3455.
- T. Nozaki, J. Ohta, T. Noguchi, H. Sato, A. Ishikawa, Y. Takaya, J.-I. Kimura, Q. Chang, K. Shimada, J.-i. Ishibashi, K. Yasukawa, K. Kimoto, K. Fujinaga and Y. Kato, Sci. Rep., 2019b, 9, 16111, DOI:10.1038/s41598-019-52709-1.
- D. R. Hassler, B. Peucker-Ehrenbrink and G. E. Ravizza, Chem. Geol., 2000, 166, 1–14, DOI:10.1016/s0009-2541(99)00180-1.
- R. Schoenberg, T. F. Nägler and J. D. Kramers, Int. J. Mass Spectrom., 2000, 197, 85–94, DOI:10.1016/S1387-3806(99)00215-8.
- M. Norman, V. Bennett, M. McCulloch and L. Kinsley, J. Anal. At. Spectrom., 2002, 17, 1394–1397, 10.1039/b204518d.
- T. Nozaki, K. Suzuki, G. E. Ravizza, J.-I. Kimura and Q. Chang, Geostand. Geoanal. Res., 2012, 36, 131–148, DOI:10.1111/j.1751-908X.2011.00125.x.
- J.-I. Kimura, T. Nozaki, R. Senda and K. Suzuki, J. Anal. At. Spectrom., 2014, 29, 1483–1490, 10.1039/c4ja00092g.
- S. B. Shirey and R. J. Walker, Anal. Chem., 1995, 67, 2136–2141, DOI:10.1021/ac00109a036.
-
D. Tuttas, J. B. Schwieters, C. Bouman and M. Deerberg, Thermo Fisher Scientific Application Note: 30192, 2009 Search PubMed.
- B. Gao, W. Li, X. Jin and L. Zhang, Microchem. J., 2019, 150, 104165, DOI:10.1016/j.microc.2019.104165.
- S. Terashima, N. Imai, M. Taniguchi, T. Okai and A. Nishimura, Geostand. Newsl., 2007, 26, 85–94, DOI:10.1111/j.1751-908X.2002.tb00626.x.
- N. Imai, S. Terashima, S. Itoh and A. Ando, Geostand. Newsl., 1996, 20, 165–216, DOI:10.1111/j.1751-908X.1996.tb00184.x.
- J. W. Morgan, D. W. Golightly and A. F. Dorrzapf Jr, Talanta, 1991, 38, 259–265, DOI:10.1016/0039-9140(91)80045-2.
- A. Tokumaru, T. Nozaki, K. Suzuki, K. T. Goto, Q. Chang, J.-I. Kimura, Y. Takaya, Y. Kato, A. Usui and T. Urabe, Geochem. J., 2015, 49, 233–241, DOI:10.2343/geochemj.2.0352.
- J.-M. Luck and C. J. Allègre, Nature, 1983, 302, 130–132, DOI:10.1038/302130a0.
- M. Ishida, T. Nozaki, Y. Takaya, J. Ohta, Q. Chang, J.-I. Kimura, K. Nakamura and Y. Kato, Deep Sea Res., Part I, 2022, 180, 103687, DOI:10.1016/j.dsr.2021.103687.
- J. R. De Laeter, J. K. Böhlke, P. De Bièvre, H. Hidaka, H. S. Peiser, K. J. R. Rosman and P. D. P. Taylor, Pure Appl. Chem., 2003, 75, 683–800, DOI:10.1351/pac200375060683.
- G. Yang, A. Zimmerman, H. Stein and J. Hannah, Anal. Chem., 2015, 87, 7017–7021, DOI:10.1021/acs.analchem.5b01751.
- C. A. Miller, B. Peucker-Ehrenbrink and L. Ball, J. Anal. At. Spectrom., 2009, 24, 1069–1078, 10.1039/B818631F.
- A. Poirier and R. Doucelance, Geostand. Geoanal. Res., 2009, 33, 195–204, DOI:10.1111/j.1751-908X.2009.00017.x.
- T. Hirata, Analyst, 1996, 121, 1407–1411, 10.1039/AN9962101407.
- J. Kuroda, R. S. Hori, K. Suzuki, D. R. Gröcke and N. Ohkouchi, Geology, 2010, 38, 1095–1098, DOI:10.1130/G31223.1.
|
This journal is © The Royal Society of Chemistry 2022 |