DOI:
10.1039/D2FO03308A
(Communication)
Food Funct., 2023,
14, 74-86
Platycodin D ameliorates hyperglycaemia and liver metabolic disturbance in HFD/STZ-induced type 2 diabetic mice†
Received
31st October 2022
, Accepted 30th November 2022
First published on 1st December 2022
Abstract
In this work, we investigated the ameliorative effects of platycodin D (PD), a major active chemical ingredient isolated from the roots of Platycodon grandiflorum (PG), on high-fat diet (HFD)/streptozotocin (STZ)-induced type 2 diabetes (T2D) mice. PD treatment (2.5 and 5.0 mg kg−1) improved HFD-induced body weight gain. PD administration also decreased the fasting blood glucose (FBG) level and improved glucose and insulin tolerance levels. These data collectively showed that PD could maintain glucose homeostasis. In addition, the diabetic mice with PD treatment also showed fewer pathological changes in liver tissues and improved hepatic functional indexes with respect to the levels of aspartate aminotransferase (AST), alanine aminotransferase (ALT), and recovery of abnormal liver function caused by T2D. Except for these, PD decreased the decomposition of hepatic glycogen. The results from western blot analysis showed that PD treatment might regulate the hepatic gluconeogenesis pathway with the increased phosphorylation/expression of AMPK and decreased expressions of PCK1 and G6Pase. In the aspect of lipid metabolism, PD decreased the whole-body lipid levels, including total cholesterol (TC), triglycerides (TG), and high-density lipoprotein (HDL), and reduced the hepatic fat accumulation induced by T2D through the AMPK/ACC/CPT-1 fatty acid anabolism pathway. In addition, the results of molecular docking showed that PD may have a potential direct effect on AMPK and other key glycolipid metabolism proteins. To summarize, PD modulation of hepatic glycolipid metabolism abnormalities is promising for T2D therapy in the future.
1. Introduction
Type 2 diabetes (T2D) is a disorder of glucose and lipid metabolism caused by insulin resistance and insufficient insulin secretion, characterized by hyperglycemia and lipid metabolism disorder.1 The liver is the main organ of glucose and lipid metabolism in the body and is sensitive to insulin,2 the development of non-alcoholic fatty liver disease (NAFLD) is closely associated with T2D and over 90% of people with T2D are diagnosed with NAFLD.3 Persistent disorders of glycolipid metabolism in the liver can cause hyperglycemia and hyperlipidemia, diabetic nephropathy, and NAFLD.4 It is well known that abnormalities in glucose and lipid metabolism often occur together, and numerous studies have shown that simultaneous control of glucose and lipid metabolism in the liver plays a very important role in the treatment of diabetes.5
In modern times, synthetic drugs are often used to treat diabetes including T2D. Taking metformin as an example, clinical studies have shown that in a short period, metformin not only has the effect of lowering blood sugar but may also have the effect of reducing body weight and hyperinsulinemia.6,7 However, clinical research about metformin pointed out that administrating synthetic drugs for a long time presented some side effects, such as nausea and lactic acidosis, weight loss, and hypoglycemia might occur.8,9 In addition, other synthetic anti-diabetic drugs also have some side effects, such as headache, edema, anemia, and cough.10 According to recent reports, some traditional Chinese medicine (TCM) extracts and natural monomer compounds with fewer side effects have good effects on fatty liver, hyperlipidemia, and hyperglycemia.11,12 In particular, various natural products containing saponin have also become substitutes for many drugs and nutritional supplements.13 Therefore, it is a hot research idea to develop natural products with good bioaccessibility as therapeutic drugs for metabolic diseases.14,15
Platycodon grandiflorum (PG) is a perennial herb widely distributed in Northeast Asia and is often processed into food.16,17 In addition, PG is officially listed as a traditional herbal medicine in China, South Korea, Japan, and other countries and is widely used in respiratory diseases such as cough, asthma, tuberculosis, and other diseases.18 In recent years, more and more studies have shown that PG has been proven to have a variety of pharmacological activities, including anti-inflammatory,19 anti-obesity, lowering cholesterol, lowering blood sugar, and anti-oxidative stress effects.20–22
Platycodin D (PD) is a triterpene saponin isolated from the roots of PG with strong pharmacological activities23 and is the symbolic component of PG.24 Recently, PD regulates high-fat diet-induced hepatic lipogenesis through the AMPK signaling pathway, while reducing serum triglyceride levels.25 Nevertheless, whether PD has an effect on T2D as well as its molecular mechanism has not been confirmed. Anti-hyperglycemia, anti-hyperlipidemic, and hepatic steatosis regulation are the basic principles for choosing anti-diabetic drugs and are further confirmed through molecular studies.26,27 Thus, we constructed a T2D murine model28 to study the regulatory effects of PD on glucose and lipid metabolism and to preliminarily investigate the mechanisms based on the AMPK-mediated glycolipid metabolism pathways. Metformin will be used as the standard drug for the treatment of T2D.
2. Materials and methods
2.1 Preparation and analysis of PD
The medicinal materials were purchased in Chifeng city, Inner Mongolia. The samples were identified by Professor Li Wei and deposited in the College of Chinese Medicinal Material, Jilin Agricultural University (Changchun, China). The medicinal materials were sun-dried, crushed, then 70% methanol was added at a 20
:
1 liquid to material ratio and the extraction was sonicated twice at room temperature. The extract obtained after centrifugal concentration was separated with AB-8 macroporous resin and then purified with silica gel. The extract obtained after centrifugal concentration was adsorbed with AB-8 macroporous resin for 12 hours, distilled water, 30% ethanol and 50% ethanol were used for elution, the part eluted with 50% ethanol was concentrated by centrifugation and then purified by silica gel to obtain the crude product. The crude product was purified by middle-pressure preparative liquid chromatography to more than 98% purity with a C18 column (SW-5223-120-SP, Santai Technologies, Inc.). The obtained substance was concentrated by centrifugation and freeze-dried to prepare powdered PD.
PD was analyzed on a Waters e2695 HPLC system (Waters Corporation, Milford, USA) equipped with a UV detector. Analysis was performed on a Waters C18 column (4.6 cm × 25 cm, 5 μm) at 30 °C and the detection wavelength was set at 210 nm. The mobile phase consisted of a mixture of acetonitrile (A) and water (B) and was set in a gradient elution program: 0–30 min, 18.0–25.0% A; 30–60 min, 25.0–27.0% A; 60–70 min, 27.0–27.0% A; 70–71 min, 27.0–18% A with a flow rate of 1.0 mL min−1. The injection volume was 20 μL.
2.2 Reagents and kits
High-fat diet (HFD) consists of 20% carbohydrate, 35% protein, and 45% fat (a percentage of total kcal 60%, in the light of D12492; Research Diets Inc.). STZ was purchased from Sigma (St. Louis, MO, USA). Low-density lipoprotein cholesterol (LDL-C), triacylglycerol (TG), total cholesterol (TC), alanine aminotransferase (ALT), aspartate aminotransferase (AST), and hematoxylin–eosin (H&E) kits were obtained from Nanjing Jiancheng Bioengineering Research Institute (Nanjing, China). Periodic acid-Schiff (PAS) and Oil Red O were obtained from Beijing Coolaber Technology Co., Ltd (Beijing, China). Immunofluorescence staining kits were purchased from BOSTER Biological Technology (Wuhan, China). The insulin (INS) ELISA kit was bought from R&D Systems (Minneapolis, MN, USA).
2.3 Experimental animals
Eight-week-old male C57BL/6 mice were obtained from Beijing Huafukang Experimental Animal Co, Ltd, with Certificate No. of SCXK (Jing) 2019–0023. The living environment of mice is maintained at a temperature of (22 ± 2 °C), relative humidity of (60% ± 10%), a 12 h light/dark cycle (6
:
00 am to 6:00 pm light), and unrestricted access to food and drinking water. All experiments were conducted by the “Regulations on the Management of Experimental Animals” of Jilin Agricultural University and approved by the Experimental Animal Ethics Committee of Jilin Agricultural University (No. 2019 07 05 001).
2.4 Experimental design
The mice were randomly divided into a control group (n = 10), and a high-fat diet group (n = 40), the control group was fed with normal feed, and the high-fat diet group was continuously fed with high-fat feed. After feeding for 11 weeks, a single intraperitoneal injection of streptozotocin (100 mg kg−1) induced diabetes. After 5 weeks, when the blood glucose stabilized, it was measured using a blood glucose tester. If the FBG level reaches or exceeds 7.8 mmol L−1, the animal is considered to have diabetes. The mice in the HFD group were divided into four groups, including the T2D model group by gavage with normal distilled water (n = 10), the positive control group by gavage with metformin 200 mg kg−1 (ref. 29 and 30) (n = 10), the low-dose group by gavage with PD 2.5 mg kg−1 (n = 10), and the high-dose group by gavage with PD 5.0 mg kg−1 (n = 10). The mice were sacrificed 8 weeks after PD treatment (Fig. 1). The blood samples and liver tissues were collected, weighed at the same time, and the liver index was calculated using the following formula: organ index (mg g−1) = organ weight/body weight. Part of the liver was placed in 10% neutral buffered formalin, embedded in paraffin, and part of the liver was frozen with OCT. And histopathological observation and immunofluorescence analysis were performed. The remaining liver tissues were freshly frozen in liquid nitrogen and stored at −80 °C.
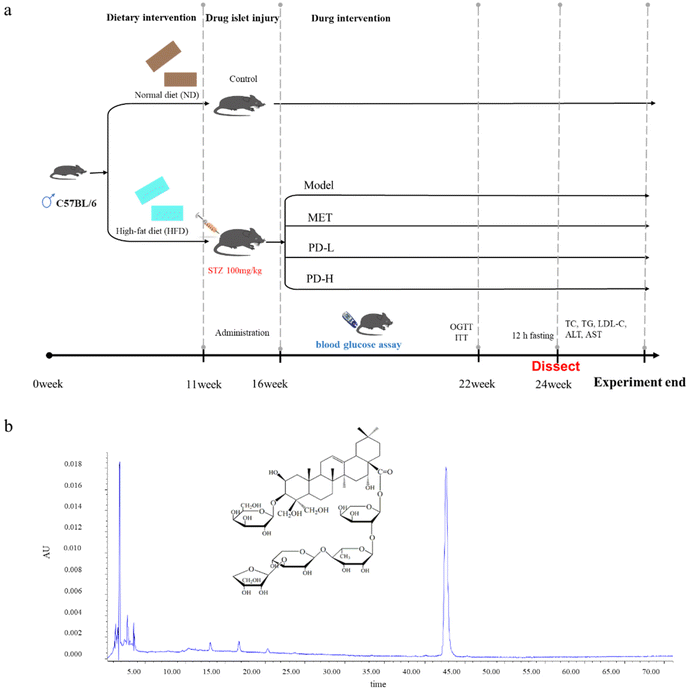 |
| Fig. 1 Schematic representation of the experimental procedure (a); HPLC chromatograms of PD (b). | |
2.5 Homeostasis model assessment of insulin resistance
Homeostasis model assessment of insulin resistance (HOMA-IR) is one of the most common methods used to evaluate insulin resistance. The HOMA-IR scores were calculated from glucose and insulin concentrations obtained from mice after 12 h fasting. The mouse serum insulin concentration was examined using a mouse insulin ELISA kit according to the manufacturer's protocol. The absorbance value was examined at 450 nm using a microplate reader. HOMA-IR was determined according to the following equation: HOMA-IR = FBG (mmol L−1) × fasting insulin (mU L−1)/22.5.
2.6 Oral glucose tolerance test and insulin tolerance test
An oral glucose tolerance test (OGTT) was performed on fasting mice with oral glucose (2.0 g per kg body weight) after 12 h fasting. We measured blood glucose levels immediately after the glucose challenge at 0, 30, 60, and 120 min. The area under the curve during the OGTT was calculated using the trapezoidal method.
Following overnight fasting of 12 h, the mice received regular insulin (0.15 U kg−1) body weight intraperitoneally. Blood glucose levels were measured at 0, 30, 60, and 120 min after insulin was injected. The area under the curve during the insulin tolerance test (ITT) was calculated using the trapezoidal method.
2.7 Measurement of biochemical parameters
According to the manufacturer's instructions, the serum lipid profiles, which included TC, LDL-C, and TG were analyzed using an Epoch Microplate Spectrophotometer (BioTek Instruments). Two basic liver function biomarkers, ALT and AST were determined at the serum level using commercially available kits according to the manufacturer's instructions. All the examinations were performed in microplates, then read at OD. Hemolyzed samples were excluded from the analysis.
2.8 Histopathological examination of liver tissues
H&E staining.
Microscopic examinations were performed to observe histopathological changes. Histopathological sections were selected randomly and analyzed under a microscope (Leica, DM 2500, Germany) at magnifications of 200 × for pathological changes including hepatocyte vacuolation/degeneration inflammatory infiltration. Liver tissues were fixed with 10% formaldehyde at room temperature for 24 h and embedded in paraffin for 24 h before the subsequent processing. The paraffin-embedded tissues were prepared at a thickness of 5 μm and stained with H&E according to the manufacturer's instructions.
PAS staining.
Liver tissues were fixed, embedded, and sectioned, then the sections were stained with 1% periodic acid for 15 min, 1% Chevron dye for 30 min, and 0.5% hematoxylin for 3–5 min at 37 °C. Images were captured under a light microscope (Leica, DM 2500, Germany). Glycogen was stained purple-red and the cell nuclei were stained light blue.
Oil red O staining.
Liver tissues were directly fixed in OCT, frozen at −80 °C, and cut into 5 μm thickness using a Leica freezing slicer (Leica, CM 3050, Germany). The sections were washed with PBS 3 times and fixed with 4% paraformaldehyde solution for 10 min. Followed by another 3 times washing with PBS, 60% isopropyl alcohol was used to rinse for 10 s, and 0.3% oil red O staining solution was added for 1 min. After rinsing with 60% isopropyl alcohol and PBS 3 times, the sections were observed and photographed under a microscope.
2.9 Molecular docking studies
The molecular structures of PD were drawn using ChemBioDraw. The 2D structure was processed and transformed into a three-dimensional (3D) structure using ChemBio3D Ultra 14.0.0.117, and the MM2 algorithm was used for energy minimization. PyMOL was used to remove the water molecules and organic matter of the target protein receptor. The Edit module in AutoDock Tools 1.5.6 was used to hydrogenate the protein, calculate the charge and add the atomic type, and the Torsion Tree module was used to determine the root of the ligand and select the ligand's twistable bond.
After completion, the PDBQ format was used to export the receptor proteins and small molecules. AutoDock Vina was run for virtual docking. It is generally accepted that the lower the energy is, the more likely the binding is to occur. Finally, the conformation with the best affinity was selected as the final docking conformation and visualized using PyMOL.
2.10 Western blot analysis
The RIPA lysis buffer was used to extract liver tissue protein and the BCA protein assay kit was used to determine the concentrations of protein (Beyotime, Shanghai, China). Western blot analysis was performed as reported previously.31 Then membranes were blocked with 5% BSA for 2 h. Subsequently, the membranes were incubated overnight with primary antibodies against G6Pase (1
:
1000), PCK1 (1
:
1000), AMPK (1
:
1000), p-AMPK (1
:
1000), PGC-1α (1
:
1000), CPT-1 (1
:
1000), ACC (1
:
1000), p-ACC (1
:
1000), and CD36 (1
:
1000) at 4 °C, the level of GAPDH (1: 1000) was assessed as a loading control, then the secondary antibody (1
:
2000) was allowed to bind to the primary antibody for 2 h at room temperature. Signals were captured using the Emitter Coupled Logic substrate (Pierce Chemical Co., Rockford, IL, USA). The intensities of each band were quantified and analyzed by using Image J software (NIH, Bethesda, MD, USA).
2.11 Statistical analysis
All analyses were performed at least three times and all data were expressed as mean ± standard deviation (mean ± S.D.) established in different experiments. The differences between the experimental groups were analyzed by one-way variance (ANOVA) with Dunnetts multiple comparison post hoc test. Results data plots were created using GraphPad Prism 6.0.4 software (GraphPad Software, Inc, San Diego, USA). A difference of p < 0.05 or p < 0.01 was statistically significant.
3.Results
3.1 Effects of PD on body weights, blood lipid, and FBG levels in T2D mice
During the experiment, there was a significant difference in body weights between the control group mice and T2D mice due to different dietary treatments, and the body weight of mice in the control group was significantly lower than the model group. Besides, as the duration of administration was increased, the body weight of the PD administration groups was lower than that of the T2D model group, as was that of the positive control group (Fig. 2a).
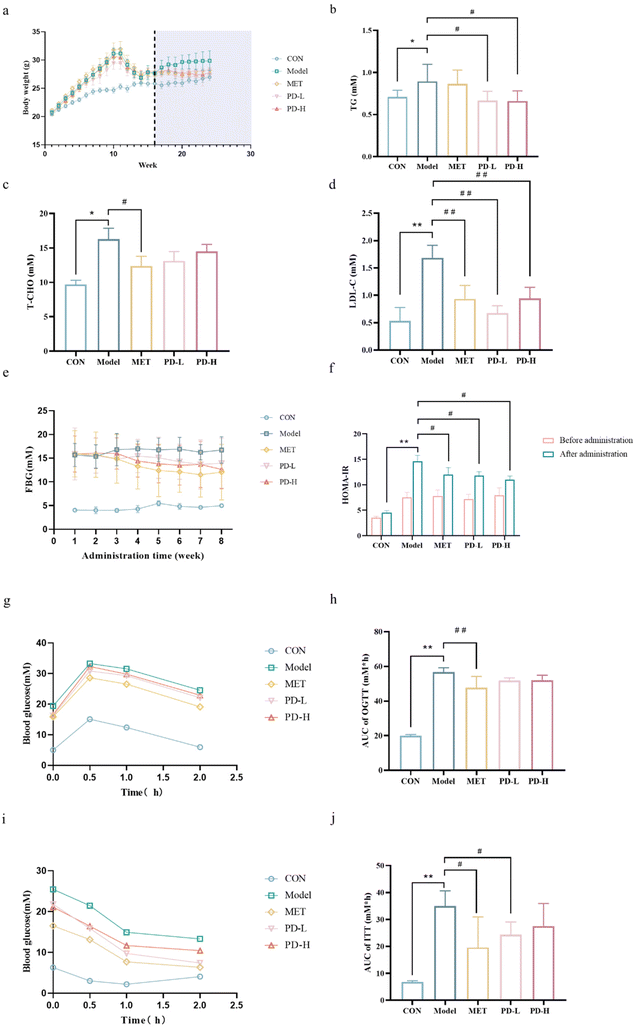 |
| Fig. 2 Effects of PD on T2D-related parameters. (a) Changes in body weights; (b) TG level; (c) T-CHO level; (d) LDL-C level. (e) Blood glucose level; (f) HOMA-IR; (g) OGGT; (h) area under the curve of OGTT; (i) ITT; (j) area under the curve of ITT. Data are expressed as mean ± SD (n = 10 in each group). **p < 0.01 vs. control group; #p < 0.05, ##p < 0.01 vs. T2D model group. | |
Diabetic individuals often have dyslipidemia, causing increased levels of serum TG, TC, and LDL-C, we found that the T2D model group mice exhibited higher levels of these three indicators than the control group mice. However, T2D mice treated with PD showed a significant decrease (p < 0.05) in the levels of TG and LDL-C, the PD (2.5 mg kg−1) group showed a better treatment trend than the PD(5 mg kg−1) group (Fig. 2b–d). Taken together, these data showed a potential mitigation effect of PD on T2D.
The FBG of the T2D model group was higher than that of the control group. When the mice's state stabilized, the FBG in the PD low-dose group was stable at 13.9 mM, and the FBG in the PD high-dose group was stable at 12.6 mM, which was not as high as the 12.0 mM in the positive control group, but much lower than the FBG level of 16.7 mM in the model group (Fig. 2e). To detect the effect of PD on the level of insulin resistance, we used HOMA-IR to quantify insulin resistance.32 PD groups had a lower average HOMA-IR score than the model group, although they are still above the control group (Fig. 2f).
3.2 Effect of PD on glucose intolerance and insulin resistance in T2D mice
The OGTT results showed that the blood glucose level increased rapidly in the model group mice after the gavage of glucose, and decreased more slowly than the other groups after reaching the highest level (Fig. 2g). Compared with the model group, mice in the PD groups and the positive control group had lower blood glucose levels within 0.5 h after glucose infusion and decreased gradually and more rapidly over the next 1 h (Fig. 2g). As a result of the area under the curve (AUC) measurement, the PD administration groups showed lower trend than the T2D model group (Fig. 2h). The results of OGTT indicate that T2D mice have insufficient tolerance to glucose. PD had a slight regulatory effect on the glucose tolerance change of T2D, proving its beneficial effect on regulating glucose homeostasis (p < 0.05).
ITT was another method to evaluate insulin resistance. During ITT progression, the initial blood glucose concentration was higher in the model group than in the other groups, and rapid reduction in blood glucose levels after insulin injection, decreased more slowly than the other groups after1 h (Fig. 2i). In the AUC measurements (Fig. 2j), the PD administration groups and the positive control group were lower than the T2D model group. ftreatment improved the insulin resistance of T2D mice, proving the improvement of their pancreatic islets.
3.3 Effect of PD on hepatic damage and hepatic steatosis in T2D mice
The livers of mice were weighed for index analysis. Due to the different dietary treatments of the control group and the T2D model group, the liver index of the model group was much higher than that of the control group. The liver index in the PD (2.5 and 5 mg kg−1) groups was lower than that in the model group, The liver index in the PD (2.5 mg kg−1) group was more close to the normal group (Fig. 3a).
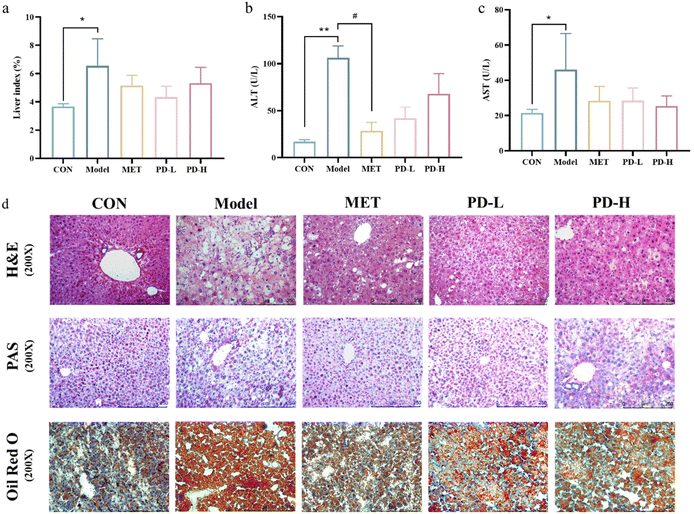 |
| Fig. 3 Effects of PD on the serum index and liver function. (a) Liver index; (b) serum ALT level; (c) serum AST level; (d) representative figures of histological abnormalities in diabetic liver tissues (400×). H&E staining was used for the analysis of histological abnormalities; PAS staining was used for the detection of glycogen (purple) in the liver tissues; Oil red O staining was used to detect liver fat deposition. Data are expressed as mean ± SD (n = 10 in each group). **p < 0.01 vs. control group; #p < 0.05, ##p < 0.01 vs. T2D model group. | |
As shown in Fig. 3b and c, compared with the control group, serum ALT and AST levels were significantly increased after HFD/STZ challenge (p < 0.01), indicating liver cell damage, and successfully established a T2D-induced a liver damage murine model. However, the serum levels of ALT and AST in the PD administration groups and the positive control group were lower than those in the model group (p < 0.01). Suggesting that PD could improve T2D-induced liver injury.
The results from H&E staining also showed that in the control group, the liver tissue cells were arranged in an orderly manner, with normal cell morphology, abundant cytoplasm, and clear boundary. However, the T2D model group showed obvious hepatic pathological changes which were accompanied by lipid vacuoles, steatosis, and cellular inflammatory infiltration. These pathological features could be alleviated by intragastric administration of PD (Fig. 3d).
To assess the distribution of glycogen in the liver, PAS staining was used. The results showed that compared with the control group, the hepatic glycogen decomposition of mice in the T2D model group was severe, and this situation could be alleviated by 8 weeks of PD administration (Fig. 3d). The results from Oil red O staining showed that fat accumulation in HFD/STZ-induced diabetic mice was more serious than that in the control group. Compared with untreated diabetic mice, PD administration reduced fat accumulation (Fig. 3d).
3.4 Molecular docking analysis of PD and related proteins
Molecular docking was used to verify whether PD plays a significant role in the regulation of proteins related to the liver gluconeogenesis pathway and fatty acid anabolism pathway. The results showed the potential binding capacity of PD to core target proteins. With the screening criteria of binding energy less than −5.0 kJ mol−1, the results all ranged from −9 to −5.3 kJ mol−1 which indicated potential binding between PD and the protein. The obtained stable complexes (Fig. 4a–c) provided a basis for AMPK, G6Pase, and ACC as key proteins for PD in the liver gluconeogenesis pathway and the fatty acid anabolism pathway. It also shows that the H-bonding interactions and hydrophobic interactions of PD with proteins are related. For example, PD forms water-mediated hydrogen bonds with amino acid residues such as GLN189, GLY159, GLU429, ARG441, and ASP427, and has a hydrophobic interaction with PHE27, ILE61, LEU160, VAL57, and LYS60.
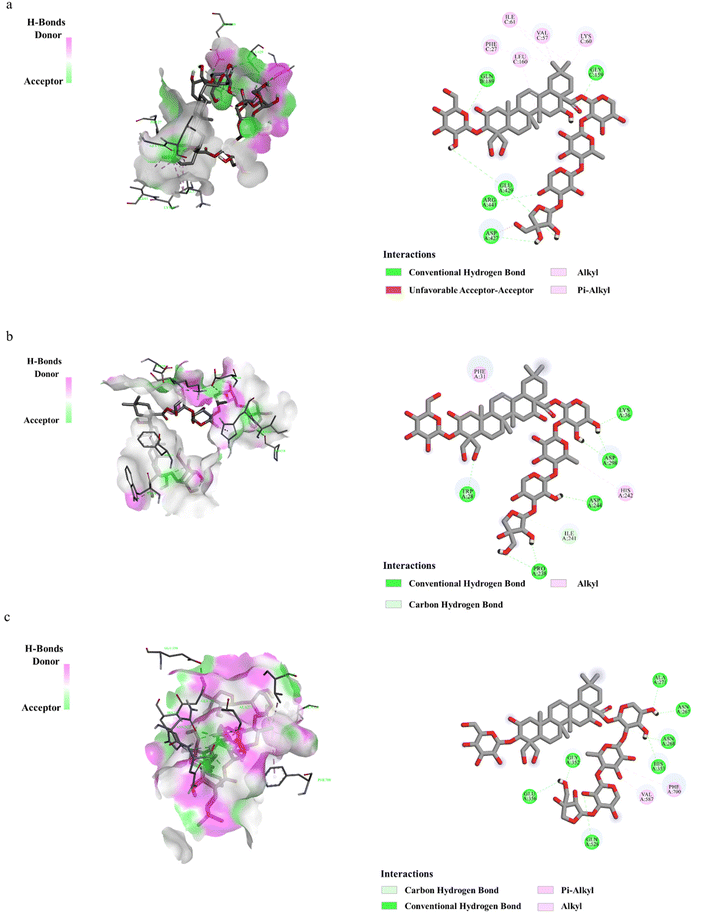 |
| Fig. 4 Binding of PD interacting with target proteins. The binding mode of PD for the proteins of AMPK (a), G6Pase (b), and ACC (c). Green represents the compounds, blue represents the associated protein, and the yellow dotted line represents the hydrophobic interactions between the compound and the associated protein. | |
3.5 Effect of PD on liver gluconeogenesis pathway in T2D mice
As shown in Fig. 5a, the expression of each protein in the AMPK/PCK1/G6Pase pathway was characterized by western blot. Excessive gluconeogenesis occurs in the diabetic state, PGC-1α was overexpressed, and the phosphorylation level of AMPK decreased. The expression of PCK1 and G6Pase promotes the occurrence and development of gluconeogenesis. Combining the band results and the optical density integral, it could be seen that PD and metformin administration could increase AMPK phosphorylation and reduce the expression of PGC-1α, PCK1, and G6Pase.
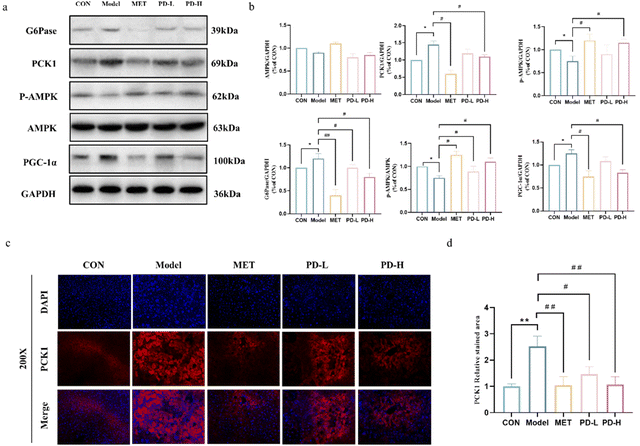 |
| Fig. 5 Effects of PD on the AMPK/PCK1/G6Pase gluconeogenesis pathway in the liver of T2D mice. (a) Western blot analysis of the AMPK/PCK1/G6Pase gluconeogenesis pathway. (b) Quantification of the AMPK/PCK1/G6Pase gluconeogenesis pathway relative to protein expression was performed by densitometric analysis. (c and d) Expression of PCK1 was displayed by immunofluorescence staining (200×). **p < 0.01 vs. control group; #p < 0.05, ##p < 0.01 vs. T2D model group. | |
To further evaluate the effect of PD on liver gluconeogenesis, we examined the effect of PD on PCK1 expression in liver tissues by immunofluorescence staining. As shown in Fig. 5c and d, the control group liver tissues showed a low expression of PCK1, while the overexpression of PCK1 was evidenced in the T2D model group and was remarkably extenuated by PD and metformin treatment (p < 0.05, p < 0.01).
3.6 Effect of PD on the fatty acid anabolism pathway in the liver of T2D mice
Abnormal hepatic lipid accumulation, one of the T2D characteristic manifestations, may be attributed to the imbalance of lipid metabolism in the liver. As shown in Fig. 6a and b, the expression of each protein in the AMPK/ACC/CPT-1 fatty acid anabolic pathway was characterized by western blot. Under the T2D state, the decrease in the phosphorylation level of AMPK could reduce the expression of ACC phosphorylation. Combined with the results of optical density integration, it could be seen that the administration of PD could promote ACC phosphorylation, and promote the expression of CPT-1, namely PD administration could reduce fatty acid synthesis and uptake as well as promote fatty acid oxidation.
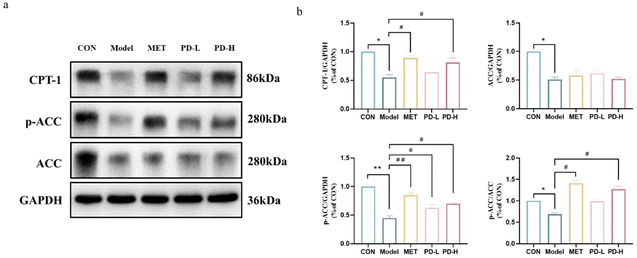 |
| Fig. 6 Effects of PD on the AMPK/ACC/CPT-1 fatty acid metabolic pathway in the liver of T2D mice. (a) Western blot analysis of the AMPK/ACC/CPT-1 fatty acid metabolic pathway. (b) Quantification of the AMPK/ACC/CPT-1 fatty acid metabolic pathway relative protein expression was performed by densitometric analysis. **p < 0.01 vs. control group; #p < 0.05, ##p < 0.01 vs. T2D model group. | |
4. Discussion
T2D is a metabolic disease caused by disturbances in glucose and fatty acid metabolism, which can lead to a variety of complications.33 The liver is one of the major organs in the regulation of glucose and fatty acid metabolism, and T2D can often lead to abnormal liver lipid metabolism, resulting in excessive accumulation of liver fat and ultimately NAFLD.34 Controlling disorders of glycolipid metabolism in the liver has become an important strategy for the prevention and control of T2D and related complications. This study was designed to evaluate the effects and mechanisms of PD on the amelioration of hyperglycemia and liver metabolic disturbance in HFD/STZ-induced T2D mice.
Abnormally high blood glucose levels are the main characteristic of T2D.35,36 Modern scholarly research has found that reasonable control of blood glucose could reduce glucose-induced toxicity and improve islet cell function, preventing and delaying the progression of diabetic complications. Notably, our study investigated the effect of PD administration in T2D mice and found that PD was effective at controlling blood glucose and regulating glucose homeostasis. In the OGTT and ITT experiments, glucose tolerance and insulin sensitivity were improved in mice administered with PD.
The liver is the central platform for the metabolism of blood glucose and fatty acids in the body and plays an important role in the metabolism of carbohydrates,37 regulating gluconeogenesis in the organism and being responsible for the balance of blood glucose. Gluconeogenesis is an important pathway for producing glucose from lactate, amino acid, and glycerol in the liver or kidneys, to ensure the homeostasis of blood glucose levels,38 its occurrence is regulated by insulin and glucagon39 and also by a series of transcription factors that feed signals to two key rate-limiting enzymes of gluconeogenesis: G6Pase and PCK1.40 Natural products have shown significant potential in modulating and activating the AMPK pathway.41,42 Our research results showed that PD administration in terms of glucose metabolism could promote the phosphorylation of AMPK, and PAS staining showed that PD decreased the decomposition of hepatic glycogen in T2D mice. This also verified that activation of AMPK could contribute to the maintenance of cellular energy homeostasis,43 enhance glucose entry into the cell and inhibit intracellular glucose production.44 PD activated AMPK, suppressed the overexpression level of G6Pase and PGC-1α, PD administration also limited the expression level of PCK1, and finally ameliorated and reversed the situations of excessive gluconeogenesis and lipid metabolism disorder caused by the HFD/STZ.
T2D induces disturbances in hepatic glucose metabolism and is often accompanied by severe lipid accumulation.45 In this study, H&E and Oil Red O staining revealed considerable hepatic steatosis, and PD treatment successfully prevented fat formation in T2D mice. This also verified the idea that PD can regulate lipid metabolism by inhibiting adipogenesis.25 Dyslipidemia is one of the main features of T2D, with elevated serum levels of TC, LDL-C, and TG and usually decreased HDL-C.46 The results showed that TC, TG, and LDL-C levels in T2D mice decreased after 8 weeks of PD administration. The liver is generally known to be the central regulator of lipid homeostasis.47 The continued accumulation of fatty acids could lead to liver injury,48 increase cell membrane permeability and release ALT and AST into the circulation, and is often used as an important marker to determine the extent of liver injury.49,50 The results of this study clearly showed the high levels of ALT and AST in the sera of HFD/STZ-induced T2D mice. In contrast, PD caused a decrease in the levels of ALT and AST. Ultimately, PD controls the development of NAFLD caused by T2D. In the study of fatty acid anabolism pathways, the administration of PD could promote ACC phosphorylation, and promote the expression of CPT-1. Based on these data, we suggest that PD administration regulates fatty acid metabolism disorder. Mechanistically, the results are in agreement with those reported in earlier studies which found that hyperglycemia activated ACC, hence increasing malonyl-CoA and reducing CPT-1 activity.51,52 Metformin is widely used in the treatment of T2D, so it is often used in T2D research as a positive drug.53 Modern studies have shown that metformin inhibits glucose production and G6Pase expression in a manner that activates AMPK, thereby inhibiting hepatic gluconeogenesis,54 activating AMPK phosphorylation levels while also inhibiting ACC and increasing CPT-1 expression to reduce hepatic lipid accumulation.55 Our study found that PD may also regulate gluconeogenesis and fatty acid metabolism in T2D by the same mechanism.
The biochemical indicators we tested did not show statistically significant differences, making clear that PD ameliorates hyperglycaemia and liver metabolic disturbance, this may indicate that the dose range we have chosen did not lead to a significant difference in efficacy, and PD may play a role below the dose of 2.5 mg kg−1. According to Sun Kyoung Lee's report, the efficacy in blocking breast cancer is produced under the conditions of using 1 mg kg−1 PD.56 Therefore, further studies are needed to determine the dosing of PD for energy metabolism regulation.
5. Conclusion
In summary, our results show that the molecular mechanism of PD regulation of glucose and lipid metabolism disorders preliminarily involved the AMPK/PCK1/G6Pase gluconeogenesis pathway and the AMPK/ACC/CPT-1 fatty acid metabolic pathway (Fig. 7) in the liver of HFD/STZ induced T2D mice. We believe that PD might be considered a potential adjuvant entity in T2D patients.
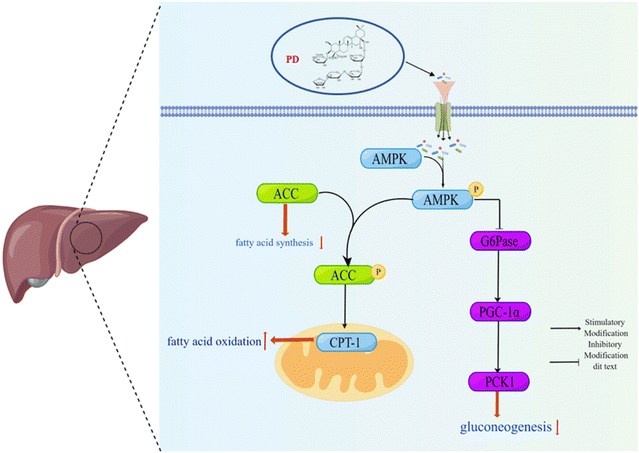 |
| Fig. 7 Schematic representation of the mechanisms of the AMPK/PCK1/G6Pase gluconeogenesis pathway and the AMPK/ACC/CPT-1 fatty acid metabolic pathway under HFD/STZ conditions. | |
Author contributions
Qiong Shen, Wei Li, and Ying-ping Wang conceived and designed the experiments. Jun-nan Hu, Si-min Qi, and Ke Li performed the experiments. Xin-dian Li and Ying-ping Wang analyzed data. Qiong Shen,Yu-te Zhong and Xiang-xiang Liu wrote the paper.
Abbreviations
T2D | Type 2 diabetes |
PG |
Platycodon grandiflorum
|
PD | Platycodin D |
HFD | High-fat diet |
STZ | Streptozotocin |
FBG | Fasting blood glucose |
TC | Total cholesterol |
TG | Triglyceride |
LDL-C | Low-density lipoprotein cholesterol |
AST | Aspartate aminotransferase |
ALT | Alanine aminotransferase |
NAFLD | Non-alcoholic fatty liver disease |
HOMA-IR | Homeostasis model assessment of insulin resistance |
OGTT | Oral glucose tolerance test |
ITT | Insulin tolerance test |
AUC | Area under the curve |
H&E | Hematoxylin–eosin |
PAS | Periodic acid-Schiff |
INS | Insulin |
Conflicts of interest
The authors declare that there are no conflicts of interest.
Acknowledgements
This work was supported by the grants of the Jilin Science & Technology Development Plan (No. 20200404007YY).
References
- S. Huang, S. Huang, X. Wang, Q. Zhang, J. Liu and Y. Leng, Downregulation of lipin-1 induces insulin resistance by increasing intracellular ceramide accumulation in C2C12 myotubes, Int. J. Biol. Sci., 2017, 13, 1–12 CrossRef CAS PubMed.
- X. Meng, J. Ma, A. N. Kang, S. Y. Kang, H. W. Jung and Y. K. Park, A Novel Approach Based on Metabolomics Coupled With Intestinal Flora Analysis and Network Pharmacology to Explain the Mechanisms of Action of Bekhogainsam Decoction in the Improvement of Symptoms of Streptozotocin-Induced Diabetic Nephropathy in Mice, Front. Pharmacol., 2020, 11, 633 CrossRef CAS PubMed.
- K. G. Tolman, V. Fonseca, A. Dalpiaz and M. H. Tan, Spectrum of liver disease in type 2 diabetes and management of patients with diabetes and liver disease, Diabetes Care, 2007, 30, 734–743 CrossRef CAS PubMed.
- S. Roumeliotis, A. Roumeliotis, A. Stamou, K. Leivaditis, K. Kantartzi, S. Panagoutsos and V. Liakopoulos, The Association of dp-ucMGP with Cardiovascular Morbidity and Decreased Renal Function in Diabetic Chronic Kidney Disease, Int. J. Mol. Sci., 2020, 21, 6035 CrossRef CAS PubMed.
- K. X. Ding, T. L. Gao, R. Xu, J. Cai, H. Q. Zhang, Y. Y. Sun, F. Zhong and A. G. Ma, Quantifying the Effect of Supplementation with Algae and Its Extracts on Glycolipid Metabolism: A Meta-Analysis of Randomized Controlled Trials, Nutrients, 2020, 12, 1712 CrossRef CAS PubMed.
- C. J. Bailey, Metformin: historical overview, Diabetologia, 2017, 60, 1566–1576 CrossRef CAS PubMed.
- E. Tseng, H. C. Yeh and N. M. Maruthur, Metformin Use in Prediabetes Among US Adults, 2005-2012, Diabetes Care, 2017, 40, 887–893 CrossRef PubMed.
- P. Tiwari, Recent Trends in Therapeutic Approaches for Diabetes Management: A Comprehensive Update, J. Diabetes Res., 2015, 2015, 340838 Search PubMed.
- L. J. McCreight, C. J. Bailey and E. R. Pearson, Metformin and the gastrointestinal tract, Diabetologia, 2016, 59, 426–435 CrossRef CAS PubMed.
- A. B. Shori, Screening of antidiabetic and antioxidant activities of medicinal plants, J. Integr. Med., 2015, 13, 297–305 CrossRef PubMed.
- M. C. Thounaojam, S. Nammi and R. Jadeja, Natural products for the treatment of obesity, metabolic syndrome, and type 2 diabetes, J. Evidence-Based Complementary Altern. Med., 2013, 2013, 871018 Search PubMed.
- T. H. Chen, M. J. Tsai, Y. S. Fu and C. F. Weng, The Exploration of Natural Compounds for Anti-Diabetes from Distinctive Species Garcinia linii with Comprehensive Review of the Garcinia Family, Biomolecules, 2019, 9, 641 CrossRef CAS PubMed.
- L. T. Bai, J. L. Gao, F. Wei, J. Zhao, D. W. Wang and J. P. Wei, Therapeutic Potential of Ginsenosides as an Adjuvant Treatment for Diabetes, Front. Pharmacol., 2018, 9, 423 CrossRef.
- A. M. L. Seca and D. Pinto, Plant Secondary Metabolites as Anticancer Agents: Successes in Clinical Trials and Therapeutic Application, Int. J. Mol. Sci., 2018, 19, 263 CrossRef PubMed.
- G. H. Kim, J. Y. Ju, K. S. Chung, S. Y. Cheon, T. Y. Gil, D. C. Cominguez, Y. Y. Cha, J. H. Lee, S. S. Roh and H. J. An, Rice Hull Extract (RHE) Suppresses Adiposity in High-Fat Diet-Induced Obese Mice and Inhibits Differentiation of 3T3-L1 Preadipocytes, Nutrients, 2019, 11, 1162 CrossRef CAS PubMed.
- Y. H. Choi, D. S. Yoo, C. W. Choi, M. R. Cha, Y. S. Kim, H. S. Lee, K. R. Lee and S. Y. Ryu, Platyconic Acid A, a Genuine Triterpenoid Saponin from the Roots of Platycodon grandiflorum, Molecules, 2008, 13, 2871–2879 CrossRef CAS PubMed.
- M. Y. Ji, A. Bo, M. Yang, J. F. Xu, L. L. Jiang, B. C. Zhou and M. H. Li, The Pharmacological Effects and Health Benefits of Platycodon grandiflorus-A Medicine Food Homology Species, Foods, 2020, 9, 142 CrossRef CAS.
- M. Park, S. Y. Park, H. J. Lee and C. E. Kim, A Systems-Level Analysis of Mechanisms of Platycodon grandiflorum Based on A Network Pharmacological Approach, Molecules, 2018, 23, 2841 CrossRef PubMed.
- W. Z. Zhang, J. A. Hou, X. T. Yan, J. Leng, R. Y. Li, J. Zhang, J. J. Xing, C. Chen, Z. Wang and W. Li, Platycodon grandiflorum Saponins Ameliorate Cisplatin-Induced Acute Nephrotoxicity through the NF-B-Mediated Inflammation and PI3K/Akt/Apoptosis Signaling Pathways, Nutrients, 2018, 10, 1328 CrossRef PubMed.
- Y. S. Park, Y. Yoon and H. S. Ahn, Platycodon grandiflorum extract represses up-regulated adipocyte fatty acid binding protein triggered by a high fat feeding in obese rats, World J. Gastroenterol., 2007, 13, 3493–3499 CrossRef CAS PubMed.
- Y. J. Kim, J. Y. Choi, R. Ryu, J. Lee, S. J. Cho, E. Y. Kwon, M. K. Lee, K. H. Liu, Y. Rina, M. K. Sung and M. S. Choi, Platycodon grandiflorus Root Extract Attenuates Body Fat Mass, Hepatic Steatosis and Insulin Resistance through the Interplay between the Liver and Adipose Tissue, Nutrients, 2016, 8, 532 CrossRef PubMed.
- H. M. Park, K. T. Park, E. C. Park, S. I. Kim, M. S. Choi, K. H. Liu and C. H. Lee, Mass Spectrometry-Based Metabolomic and Lipidomic Analyses of the Effects of Dietary Platycodon grandiflorum on Liver and Serum of Obese Mice under a High-Fat Diet, Nutrients, 2017, 9, 71 CrossRef PubMed.
- X. Su, Y. Liu, L. Han, Z. Wang, M. Cao, L. Wu, W. Jiang, F. Meng, X. Guo, N. Yu, S. Gui, S. Xing and D. Peng, A candidate gene identified in converting platycoside E to platycodin D from Platycodon grandiflorus by transcriptome and main metabolites analysis, Sci. Rep., 2021, 11, 9810 CrossRef CAS PubMed.
- Y. H. Fu, Z. Y. Xin, B. Liu, J. X. Wang, J. J. Wang, X. Zhang, Y. N. Wang and F. Li, Platycodin D Inhibits Inflammatory Response in LPS-Stimulated Primary Rat Microglia Cells through Activating LXR alpha-ABCA1 Signaling Pathway, Front. Immunol., 2018, 8, 1929 CrossRef PubMed.
- Y. P. Hwang, J. H. Choi, H. G. Kim, T. Khanal, G. Y. Song, M. S. Nam, H. S. Lee, Y. C. Chung, Y. C. Lee and H. G. Jeong, Saponins, especially platycodin D, from Platycodon grandiflorum modulate hepatic lipogenesis in high-fat diet-fed rats and high glucose-exposed HepG2 cells, Toxicol. Appl. Pharmacol., 2013, 267, 174–183 CrossRef CAS PubMed.
- K. Srinivasan and P. Ramarao, Animal models in type 2 diabetes research: An overview, Indian J. Med. Res., 2012, 136, 451–472 Search PubMed.
- L. H. Glimcher and A. H. Lee, From Sugar to Fat How the Transcription Factor XBP1 Regulates Hepatic Lipogenesis, Integr. Physiol., 2009, 1173, E2–E9 CAS.
- Y. Li, J. G. Hou, Z. Liu, X. J. Gong, J. N. Hu, Y. P. Wang, W. C. Liu, X. H. Lin, Z. Wang and W. Li, Alleviative effects of 20(R)-Rg3 on HFD/STZ-induced diabetic nephropathy via MAPK/NF-kappa B signaling pathways in C57BL/6 mice, J. Ethnopharmacol., 2021, 267, 113500 CrossRef CAS PubMed.
- K. H. Yap, G. S. Yee, M. Candasamy, S. C. Tan, S. Md, A. B. Abdul Majeed and S. K. Bhattamisra, Catalpol Ameliorates Insulin Sensitivity and Mitochondrial Respiration in Skeletal Muscle of Type-2 Diabetic Mice Through Insulin Signaling Pathway and AMPK/SIRT1/PGC-1alpha/PPAR-gamma Activation, Biomolecules, 2020, 10, 1360 CrossRef CAS PubMed.
- J. Wang, M. Huang, J. Yang, X. Ma, S. Zheng, S. Deng, Y. Huang, X. Yang and P. Zhao, Anti-diabetic activity of stigmasterol from soybean oil by targeting the GLUT4 glucose transporter, Food Nutr. Res., 2017, 61, 1364117 CrossRef PubMed.
- W. T. Wang, M.
L. Fan, J. N. Hu, J. Y. Sha, H. Zhang, Z. Wang, J. J. Zhang, S. H. Wang, S. W. Zheng and W. Li, Maltol, a naturally occurring flavor enhancer, ameliorates cisplatin-induced apoptosis by inhibiting NLRP3 inflammasome activation by modulating ROS-mediated oxidative stress, J. Funct. Foods, 2022, 94, 105127 CrossRef CAS.
- Y. Chang, C. K. Kim, M. K. Kim, W. K. Seo and K. Oh, Insulin resistance is associated with poor functional outcome after acute ischemic stroke in non-diabetic patients, Sci. Rep., 2021, 11, 1129 CrossRef PubMed.
- R. W. Alfa and S. K. Kim, Using Drosophila to discover mechanisms underlying type 2 diabetes, Dis. Models Mech., 2016, 9, 365–376 CrossRef CAS PubMed.
- S. Ballestri, S. Zona, G. Targher, D. Romagnoli, E. Baldelli, F. Nascimbeni, A. Roverato, G. Guaraldi and A. Lonardo, Nonalcoholic fatty liver disease is associated with an almost twofold increased risk of incident type 2 diabetes and metabolic syndrome. Evidence from a systematic review and meta-analysis, J. Gastroenterol. Hepatol., 2016, 31, 936–944 CrossRef CAS PubMed.
- M. E. Healy, S. Lahiri, S. R. Hargett, J. D. Chow, F. L. Byrne, D. S. Breen, B. M. Kenwood, E. P. Taddeo, C. Lackner, S. H. Caldwell and K. L. Hoehn, Dietary sugar intake increases liver tumor incidence in female mice, Sci. Rep., 2016, 6, 22292 CrossRef CAS PubMed.
- T. Ye, X. Meng, Y. Zhai, W. Xie, R. Wang, G. Sun and X. Sun, Gastrodin Ameliorates Cognitive Dysfunction in Diabetes Rat Model via the Suppression of Endoplasmic Reticulum Stress and NLRP3 Inflammasome Activation, Front. Pharmacol., 2018, 9, 1346 CrossRef CAS PubMed.
- W. Y. Liu, D. J. Lu, X. M. Du, J. Q. Sun, J. Ge, R. W. Wang, R. Wang, J. Zou, C. Xu, J. Ren, X. F. Wen, Y. Liu, S. M. Cheng, X. Tan, S. Pekkala, E. Munukka, P. Wiklund, Y. Q. Chen, Q. Gu, Z. C. Xia, J. J. Liu, W. B. Liu, X. B. Chen, Y. M. Zhang, R. Li, R. J. H. Borra, J. X. Yao, P. J. Chen and S. L. Cheng, Effect of aerobic exercise and low carbohydrate diet on pre-diabetic non-alcoholic fatty liver disease in postmenopausal women and middle aged men - the role of gut microbiota composition: study protocol for the AELC randomized controlled trial, BMC Public Health, 2014, 14, 48 CrossRef PubMed.
- Y. X. Zhu, H. Q. Hu, M. L. Zuo, L. Mao, G. L. Song, T. M. Li, L. C. Dong, Z. B. Yang and M. S. Ali Sheikh, Effect of oxymatrine on liver gluconeogenesis is associated with the regulation of PEPCK and G6Pase expression and AKT phosphorylation, Biomed. Rep., 2021, 15, 56 CrossRef CAS PubMed.
- K. B. Zhang, X. Q. Guo, H. Yan, Y. X. Wu, Q. Pan, J. Z. Shen, X. P. Li, Y. M. Chen, L. Li, Y. J. Qi, Z. H. Xu, W. Xie, W. P. Zhang, D. Threadgill, L. He, D. Villarreal, Y. X. Sun, M. F. White, H. T. Zheng and S. D. Guo, Phosphorylation of Forkhead Protein FoxO1 at S253 Regulates Glucose Homeostasis in Mice, Endocrinology, 2019, 160, 1333–1347 CrossRef CAS PubMed.
- N. Kumashiro, Y. Tamura, T. Uchida, T. Ogihara, Y. Fujitani, T. Hirose, H. Mochizuki, R. Kawamori and H. Watada, Impact of oxidative stress and peroxisome proliferator-activated receptor gamma coactivator-1alpha in hepatic insulin resistance, Diabetes, 2008, 57, 2083–2091 CrossRef CAS PubMed.
- C. E. Lee, H. J. Hur, J. T. Hwang, M. J. Sung, H. J. Yang, H. J. Kim, J. H. Park, D. Y. Kwon and M. S. Kim, Long-Term Consumption of Platycodi Radix Ameliorates Obesity and Insulin Resistance via the Activation of AMPK Pathways, J. Evidence-Based Complementary Altern. Med., 2012, 2012, 759143 Search PubMed.
- F. L. Meng, X. T. Su, W. Li and Y. N. Zheng, Ginsenoside Rb3 strengthens the hypoglycemic effect through AMPK for inhibition of hepatic gluconeogenesis, Exp. Ther. Med., 2017, 13, 2551–2557 CrossRef CAS PubMed.
- L. A. J. O'Neill and D. G. Hardie, Metabolism of inflammation limited by AMPK and pseudo-starvation, Nature, 2013, 493, 346–355 CrossRef PubMed.
- Y. Adachi, A. L. De Sousa-Coelho, I. Harata, C. Aoun, S. Weimer, X. Shi, K. N. G. Herrera, H. Takahashi, C. Doherty, Y. Noguchi, L. J. Goodyear, M. C. Haigis, R. E. Gerszten and M. E. Patti, L-Alanine activates hepatic AMP-activated protein kinase and modulates systemic glucose metabolism, Mol. Metab., 2018, 17, 61–70 CrossRef CAS PubMed.
- J. Xiang, Q. Lv, F. Yi, Y. Song, L. Le, B. Jiang, L. Xu and P. Xiao, Dietary Supplementation of Vine Tea Ameliorates Glucose and Lipid Metabolic Disorder via Akt Signaling Pathway in Diabetic Rats, Molecules, 2019, 24, 1866 CrossRef CAS PubMed.
- S. C. Chen and C. H. Tseng, Dyslipidemia, kidney disease, and cardiovascular disease in diabetic patients, Rev. Diabet. Stud., 2013, 10, 88–100 CrossRef PubMed.
- M. M. Angrish, C. A. McQueen, E. Cohen-Hubal, M. Bruno, Y. Ge and B. N. Chorley, Mechanistic Toxicity Tests Based on an Adverse Outcome Pathway Network for Hepatic Steatosis, Toxicol. Sci., 2017, 159, 159–169 CrossRef CAS PubMed.
- G. S. Hotamisligil and R. J. Davis, Cell Signaling and Stress Responses, Cold Spring Harbor Perspect. Biol., 2016, 8, a006072 CrossRef PubMed.
- J. Kim, H. Lee, J. Lim, J. Oh, S. S. Shin and M. Yoon, The Angiogenesis Inhibitor ALS-L1023 from Lemon-Balm Leaves Attenuates High-Fat Diet-Induced Nonalcoholic Fatty Liver Disease through Regulating the Visceral Adipose-Tissue Function, Int. J. Mol. Sci., 2017, 18, 846 CrossRef PubMed.
- S. Abdulmalek, A. Eldala, D. Awad and M. Balbaa, Ameliorative effect of curcumin and zinc oxide nanoparticles on multiple mechanisms in obese rats with induced type 2 diabetes, Sci. Rep., 2021, 11, 20667 CrossRef PubMed.
- K. Chen, X. Chen, H. L. Xue, P. W. Zhang, W. J. Fang, X. C. Chen and W. H. Ling, Coenzyme Q10 attenuates high- fat diet- induced non- alcoholic fatty liver disease through activation of the AMPK pathway, Food Funct., 2019, 10, 814–823 RSC.
- M. Kohjima, N. Higuchi, M. Kato, K. Kotoh, T. Yoshimoto, T. Fujino, M. Yada, R. Yada, N. Harada, M. Enjoji, R. Takayanagi and M. Nakamuta, SREBP-1c, regulated by the insulin and AMPK signaling pathways, plays a role in nonalcoholic fatty liver disease, Int. J. Mol. Med., 2008, 21, 507–511 CAS.
- H. Lu, X. Han, J. Ren, K. Ren, Z. Li and Q. Zhang, Metformin attenuates synergic effect of diabetes mellitus and Helicobacter pylori infection on gastric cancer cells proliferation by suppressing PTEN expression, J. Cell. Mol. Med., 2021, 25, 4534–4542 CrossRef CAS PubMed.
- X. Stephenne, M. Foretz, N. Taleux, G. C. van der Zon, E. Sokal, L. Hue, B. Viollet and B. Guigas, Metformin activates AMP-activated protein kinase in primary human hepatocytes by decreasing cellular energy status, Diabetologia, 2011, 54, 3101–3110 CrossRef CAS PubMed.
- C. Agard, M. Rolli-Derkinderen, E. Dumas-de-La-Roque, M. Rio, C. Sagan, J. P. Savineau, G. Loirand and P. Pacaud, Protective role of the antidiabetic drug metformin against chronic experimental pulmonary hypertension, Br. J. Pharmacol., 2009, 158, 1285–1294 CrossRef CAS PubMed.
- S. K. Lee, K. K. Park, H. J. Kim, K. R. Kim, E. J. Kang, Y. L. Kim, H. Yoon, Y. S. Kim and W. Y. Chung, Platycodin D Blocks Breast Cancer-Induced Bone Destruction by Inhibiting Osteoclastogenesis and the Growth of Breast Cancer Cells, Cell. Physiol. Biochem., 2015, 36, 1809–1820 CrossRef CAS PubMed.
|
This journal is © The Royal Society of Chemistry 2023 |
Click here to see how this site uses Cookies. View our privacy policy here.