DOI:
10.1039/D2FO00753C
(Paper)
Food Funct., 2022,
13, 8967-8976
Ameliorative effects of chickpea flavonoids on redox imbalance and mitochondrial complex I dysfunction in type 2 diabetic rats
Received
18th March 2022
, Accepted 20th July 2022
First published on 8th August 2022
Abstract
Chickpeas are an important source of flavonoids in the human diet, and researchers have demonstrated that flavonoids have antidiabetic compositions in chickpeas. Because the NAD+/NADH redox balance is heavily perturbed in diabetes and complex I is the only site for NADH oxidation and NAD+ regeneration, in the present study, mitochondrial complex I was used as a target for anti-diabetes. The objective of this study was to investigate the effects of a crude chickpea flavonoid extract (CCFE) on NAD+/NADH redox imbalance and mitochondrial complex I dysfunction in the pancreas as well as oxidative stress in type 2 diabetes mellitus (T2DM) rats. Our results demonstrated that the degree of NAD+/NADH redox imbalance in the pancreas of T2DM rats was alleviated by CCFE, which is likely attributed to the inhibition of the polyol pathway and the decrease in poly ADP ribose polymerase (PARP) and sirtuin 3 (Sirt3) activities. Moreover, mitochondrial complex I dysfunction in the pancreas of T2DM rats was ameliorated by CCFE through the suppression of the activity of complex I. Furthermore, CCFE treatment could attenuate oxidative stress in T2DM rats, which was proven by the reduction in hydrogen peroxide (H2O2) and malondialdehyde (MDA) as well as the upregulation of glutathione peroxidase (GSH-Px) and superoxide dismutase (SOD) in serum. CCFE treatment significantly improved dyslipidemia in T2DM rats.
1. Introduction
Diabetes mellitus, which is often responsible for severe health problems, is a global disease that is a major cause of morbidity worldwide.1 Although the treatment of diabetes with insulin and oral drugs has remarkable therapeutic effects, macrovascular, retinal, and neuropathic function complications are still associated with patients receiving insulin injections or synthetic medicine.2 Improvement in the blood glucose control through a combination of lifestyle modifications and oral modifications may slow the rate of this progression and enhance the quality of life for people with type 2 diabetes mellitus (T2DM).3 The use of alternative medicine, particularly the consumption of natural products with antidiabetic activities, has been increasing.
Epidemiological evidence suggests that several dietary patterns and abundant plant food content are favorably associated with the prevention of obesity and T2DM,4–6 and the health benefits of bean or legume consumption have received increasing attention. Among the legumes, chickpea (Cicer arietinum L.) is the third most important grain legume in the world in terms of total grain production.7 Chickpeas are rich in carbohydrates (starch, dietary fiber, glucose, sucrose, and oligosaccharides) and proteins (all essential amino acids excluding sulfur-containing amino acids) and thus serve as an important source of nutrients in vegetarian diets.8,9 Furthermore, chickpeas contain biologically active compounds such as flavonoids. Previous studies have shown that the flavonoid baicalin from Scutellaria baicalensis mitigates oxidative stress and reduces hepatic triglyceride and cholesterol levels in diabetic rats.10 A diet rich in isoflavones has a lower risk of diabetes and diabetes-related complications, such as cardiovascular diseases.11,12 Formononetin treatment reduces insulin resistance and attenuates hyperglycemia by increasing the expression of Sirt1 in the pancreatic tissues of T2DM rats.13 Moreover, biochanin A exerts hypoglycemic and antilipemic effects through mechanisms that might be associated with the repair of pancreatic β cells in diabetic rats.14 Soy isoflavones, namely genistein and daidzein, have been found to regulate lipid metabolism through an AKT/mTORC1 pathway in diet-induced obesity (DIO) male rats.15
Complex I of mammalian cell mitochondria is a major enzyme in the mitochondrial electron transport chain that oxidizes NADH to NAD+ and is a major site for the generation of reactive oxygen species (ROS).16,17 In diabetes, however, numerous branching-off glucose metabolic pathways, including the polyol pathway and hexosamine pathway, which are usually dormant under normal conditions, are activated or upregulated by high levels of blood glucose.18,19 On the other hand, complex I is able to induce ROS production that leads to DNA oxidative damage, resulting in the excessive activation of poly ADP ribose polymerase (PARP) in diabetes.20–22 The outcome of the activation of the polyol pathway and PARP is thus a redox imbalance, reflected by the decreased levels of the NAD+/NADH ratio.23 As complex I handles the additional amount of NADH produced by the polyol pathway, complex I dysfunction would likely mean increased complex I activity.24 Moreover, oxidative stress has been considered to have a great influence on diabetes and its complications. Therefore, efficient NADH oxidation by complex I would be beneficial for diabetic individuals.
In the present study, after inducing diabetes in rats as a T2DM model by feeding a high-fat diet (HFD) combined with streptozotocin (STZ) injection, we assessed the effects of the crude chickpea flavonoid extract (CCFE) on NAD+/NADH redox imbalance and mitochondrial complex I dysfunction in the pancreas of T2DM rats. Moreover, we studied the effects of CCFE on the polyol pathway and the PARP and sirtuin 3 (Sirt3) activities in the pancreas of T2DM rats. In addition, the effects of CCFE on oxidative stress in T2DM rats were also observed by measuring the protein carbonylation content in the pancreas and the H2O2 content, MDA content, SOD activity, and GSH-Px activity in serum.
2. Materials and methods
2.1 Plant materials
Kabuli chickpeas were purchased from Yingge Biotechnology Co, Ltd (Mulei, China). After removing broken and diseased seeds, chickpeas were ground to a powder using a grinder. Then, the powder was dried for 3 h at 50 °C and stored at 4 °C for later use.
2.2 Chemicals and reagents
The STZ used in the present study was purchased from (Sigma Chemicals, USA), and rutin (HPLC ≥ 98%) was purchased from Solarbio (Beijing, China). All the other chemicals used in the present work were at least of analytical grade.
2.3 Extraction of CCFE
Flavonoid extraction was performed as described previously25 with slight modifications. In brief, the powder was defatted by homogenization with petroleum ether with a solid/liquid ratio of 1
:
10 g mL−1 at room temperature for 12 h. Then, the defatted samples were extracted with 70% ethanol with a solid/liquid ratio of 1
:
20 g mL−1 for 4 h at 70 °C. Next, the extracts were filtered and concentrated with a rotavapor device. Finally, the CCFE was obtained using vacuum freeze drying and was stored at −80 °C until use. The CCFE was determined via the aluminum nitrate coloration approach. Rutin was the reference standard, and the concentration of flavonoids was determined by UV spectroscopy at a wavelength of 504 nm.
2.4 Animals
The experimental protocols were approved by the Animal Ethics Committee of Xinjiang Medical University in China (Approval number: IACUC20200924-22), and the use of animals was in accordance with the National Institutes of Health's Guide for the Care and Use of Laboratory Animals. Male SD rats (164 ± 16 g) were purchased from the Animal Center of the College of Medicine, Xinjiang Medical University (Xinjiang, China). All rats were kept under controlled temperatures (25 ± 2 °C) and relative humidity (50–70%) on a 12/12 h light/dark cycle with free access to water and a normal diet for 7 days before the experiment.
2.5 Establishment of the T2DM model in rats
The T2DM rat model was established according to the following method. In brief, the rats in the normal control (NC) group were fed a normal chow diet, whereas all the others were given a high-fat diet (HFD) (45% of kcal from fat, Medicience Ltd, Jiangsu, China) for 4 weeks. After fasting for 12 h, all HFD-fed rats received a single intraperitoneal injection of STZ at a dosage of 45 mg kg−1. STZ solutions were made fresh by dissolving in 0.1 mol L−1 sodium citrate buffer (pH 4.5), and NC rats received sodium citrate buffer only. One week later, the rats were fasted for 12 h, and blood glucose levels were determined in blood collected by tail-cutting using blood glucose test strips (PRO Doctor, Taipei). Animals with blood levels exceeding 11.1 mmol L−1 were considered to have T2DM.
2.6 Treatment of T2DM rats with CCFE
Subsequently, the confirmed T2DM rats were divided into five groups consisting of 10 diabetic rats each: diabetic model control (DM), positive control group (PC), and three CCFE-treated groups. The animal experimental design flowchart is shown in Fig. 1. For the CCFE treatment, diabetic rats were intragastrically (ig) administered 0.244 (CCFE-L), 0.732 (CCFE-M), and 1.222 (CCFE-H) g kg−1 CCFE (1 g of CCFE contains 4.9242 mg of flavonoids) once a day for 6 weeks. All T2DM rats were fed an HFD, while NC group rats were fed a normal chow diet throughout the experimental period. All rats were fasted for 12 h after the administration for 6 weeks. Blood samples were collected from the abdominal aorta for serum preparation and biochemical analysis. Then, the rats were sacrificed, and pancreas tissue were immediately taken. One part of the pancreas tissue was stored at −80 °C for analysis of the NAD+/NADH ratio, complex I to V activities, PARP activity, aldose reductase (AR) activity, and Sirt3 activity, and the other part was fixed in 10% formalin for histopathological examination.
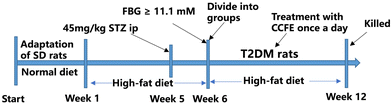 |
| Fig. 1 Animal experimental design. | |
2.7 Serum biochemical analysis
Serum total cholesterol (TC), triglyceride (TG), low-density lipoprotein cholesterol (LDLC), and high-density lipoprotein cholesterol (HDLC) were measured using TC, TG, LDLC, and HDLC determination kits (Nanjing Jiancheng Bioengineering Institute, Nanjing, China), respectively, according to the manufacturer's instructions. Serum fasting insulin (FINS) was tested by ELISA kits (Shanghai Jianglai Industrial Limited by Share Ltd, Shanghai, China). The homeostasis model assessment (HOMA)-insulin sensitivity (HOMA-IS) index and HOMA-insulin resistance (HOMA-IR) index were calculated using the following formulas: HOMA-IS = ln
[1/(FINS × FBG)], HOMA-IR = (FINS × FBG)/22.5.
2.8 Isolation of pancreatic mitochondria
Pancreatic mitochondrial preparation from rats was achieved using a gradient centrifugation method. Essentially, 0.1000 g of rat pancreatic tissues was homogenized on ice with 1 mL of the extracting solution. The homogenates were centrifuged at 600g for 10 min at 4 °C, and the precipitate was discarded. The supernatant was further centrifuged at 11
000g for 15 min at 4 °C. The final mitochondrial pellet was either used immediately or frozen at −80 °C until use.
2.9 Measurements of the NAD+/NADH ratio, ATP, and the mitochondrial membrane potential in the pancreas
The NAD+/NADH ratio in the pancreas was measured using a coenzyme I NAD(H) content kit purchased from Solarbio (Beijing, China) following the manufacturer's instructions. The ATP content and mitochondrial membrane potential in the pancreas were determined by ATP and membrane potential detection kits purchased from Solarbio (Beijing, China).
2.10 Enzyme activity assays
Mitochondrial complex I activity, complex II activity, complex III activity, complex IV activity, and complex V activity were measured by spectrophotometric methods using the complex I enzyme activity microplate assay kit, complex II enzyme activity microplate assay kit, complex III enzyme activity microplate assay kit, complex IV enzyme activity microplate assay kit and complex V enzyme activity microplate assay kit (Solarbio, Beijing, China). The activities of AR, PARP, and Sirt3 and the protein carbonylation content in pancreatic tissue were tested by ELISA kits (Shanghai Jianglai Industrial Limited by Share Ltd, Shanghai, China).
2.11 Measurement of MDA, H2O2, GSH-Px, and SOD
The serum malondialdehyde (MDA) content was measured by the thiobarbituric acid (TBA) method using a kit purchased from Solarbio (Beijing, China). The serum H2O2 content and SOD activity were measured using kits from Solarbio (Beijing, China). The serum GSH-Px activity was measured by the colorimetric method using a kit purchased from Nanjing Jiancheng Bioengineering Institute (Nanjing, China).
2.12 Isolation of pancreatic tissue and histological examination
After euthanasia, the pancreas of the rats was quickly removed, placed in a cold saline solution, fixed in 10% formalin for one week at room temperature, and then embedded in paraffin wax. Each paraffin-blocked pancreas and sections were stained with hematoxylin and eosin before a microtome was used. After the slides were coded, the pancreatic tissue was examined under a light microscope.
2.13 Statistical analysis
The results are expressed as the mean ± standard deviation, and the data were analyzed by SPSS 20.0 software (SPSS, Inc., Chicago, IL). One-way analysis of variance (ANOVA) with the Duncan's multiple range test was used to compare the differences among various groups. A value of p < 0.05 was considered statistically significant.
3. Results and discussion
3.1 Effects of CCFE on fasting blood glucose (FBG) and insulin resistance in T2DM rats
In the study, after HFD feeding for 4 weeks and STZ injection, rats with FBG levels greater than 11.1 mmol L−1 were selected for the experiment and divided into groups. As shown in Fig. 2A, FBG levels in the DM control group were dramatically increased (p < 0.001) compared with those in the NC group. After the administration of CCFE for 6 weeks, FBG levels in CCFE-treated groups were potently reduced (p < 0.001) compared with the DM control group.
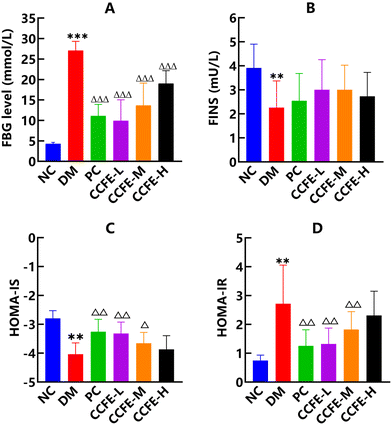 |
| Fig. 2 Effects of CCFE on FBG, FINS, HOMA-IR, and HOMA-IS levels in T2DM rats. (A) FBG; (B) FINS; (C) HOMA-IS; (D) HOMA-IR. **p < 0.01 and ***p < 0.001, compared with the NC group; Δp < 0.05, ΔΔp < 0.01 and ΔΔΔp < 0.001, compared with the DM control group. NC: normal control group, DM: diabetic model control group, PC: positive control group, CCFE-(L, M, H): (low, middle, high) dose CCFE-treated group. | |
Insulin resistance (IR), a hallmark of type 2 diabetes (T2D), is characterized by a blunted ability of peripheral tissues to regulate glucose homeostasis in response to insulin.26 As shown in Fig. 2B–D, the FINS and HOMA-IS levels were markedly reduced (p < 0.01), whereas HOMA-IR was substantially increased (p < 0.01) in the DM control group compared with the NC group. After the administration of CCFE, we observed a great decrease in HOMA-IR (p < 0.01) and a significant increase in HOMA-IS (p < 0.01 and p < 0.05) in the CCFE-L and CCFE-M groups compared with the DM control group. These results indicated that insulin resistance was clearly relieved and that insulin sensitivity was elevated in T2DM rats by the administration of CCFE.
3.2 Effects of CCFE on dyslipidemia in T2DM rats
Studies have shown that dyslipidemia is a metabolic feature of T2D.27 As shown in Fig. 3A–D, the DM control group exhibited significantly higher levels of TG, TC, and LDLC and a lower level of HDLC in serum than those of the NC group, which agreed with a previous report showing that a continuous high-fat and high-glucose diet could induce dyslipidemia in diabetic rats.28 After supplementation with CCFE, there was a great decrease in TG, TC, and LDLC (p < 0.05) and a significant increase in HDLC (p < 0.05) compared with levels in the DM control group. These results showed that dyslipidemia in T2DM rats could be regulated via treatment with CCFE. Moreover, Bhathena et al.29 investigated the effects of soy isoflavones on diabetes and found that biochanin A possesses excellent hypolipidemic properties. Aqueous extract of alfalfa revealed ameliorative effects on blood lipids in alloxan-induced diabetic Wistar rats as reported by Farsani et al.30
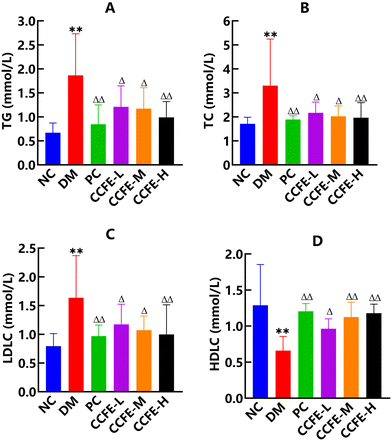 |
| Fig. 3 Effects of CCFE on dyslipidemia in the serum of T2DM rats. (A) TG; (B) TC; (C) LDLC; (D) HDLC. **p < 0.01, compared with the NC group; Δp < 0.05 and ΔΔp < 0.01, compared with the DM control group. NC: normal control group, DM: diabetic model control group, PC: positive control group, CCFE-(L, M, H): (low, middle, high) dose CCFE-treated group. | |
3.3 CCFE ameliorated pancreatic damage in T2DM rats
It has been shown that insulin resistance is closely associated with impaired pancreatic β-cell function.31 Photomicrographs of hematoxylin and eosin staining of the pancreas are shown in Fig. 4. We found that HFD and STZ triggered severe injury to the pancreas of the diabetic rats. Intact pancreatic structure and neatly aligned islets with the clusters of purple-granulated cells were observed in the NC group (Fig. 4A), while histopathological changes characterized by the degeneration and vacuolation of pancreatic cells, blood-filled interlobular ducts, and a decrease in the number of islets were seen in the DM control group (Fig. 4B). However, all these injury features were significantly reduced in CCFE-L (Fig. 4D), CCFE-M (Fig. 4E), CCFE-H (Fig. 4F) and the PC groups (Fig. 4C). The data demonstrated that CCFE could ameliorate pancreatic injuries in T2DM rats and may be a potential candidate for diabetes treatment.
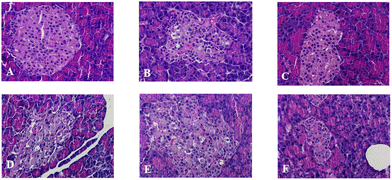 |
| Fig. 4 Histological sections of the pancreas of the normal control and T2DM rats (hematoxylin and eosin histochemical staining). (A) NC; (B) DM; (C) PC; (D) CCFE-L; (E) CCFE-M; (F) CCFE-H. Scale bar: 50 μm. NC: normal control group, DM: diabetic model control group, PC: positive control group, CCFE-(L, M, H): (low, middle, high) dose CCFE-treated group. | |
3.4 Effects of CCFE on NAD+/NADH redox imbalance in the pancreas of T2DM rats
In this study, we investigated the NAD+/NADH redox state in the pancreas of T2DM rats. Fig. 5A shows that the ratio of NAD+ to NADH in the pancreas of the DM control group decreased (p < 0.001) compared with that in the NC group, indicating that the NAD+/NADH redox balance was perturbed in the pancreas of T2DM rats with NADH being in excess and NAD+ being depleted. Studies have claimed that there is NAD+/NADH redox imbalance in the diabetic pancreas and lungs.32,33 After treatment with CCFE, the NAD+/NADH ratio in the CCFE-M and CCFE-H groups significantly increased (p < 0.05) compared with that in the DM control group, which confirmed that the NAD+/NADH redox imbalance in the pancreas of T2DM rats was alleviated by CCFE. Moreover, Ogura et al.34 found that apigenin increased the intracellular NAD+/NADH ratio in the kidneys of diabetic rats by inhibiting the CD38 activity. Luo et al.35 mentioned that resveratrol attenuated ethanol-induced insulin resistance in rats by improving the NAD+/NADH ratio.
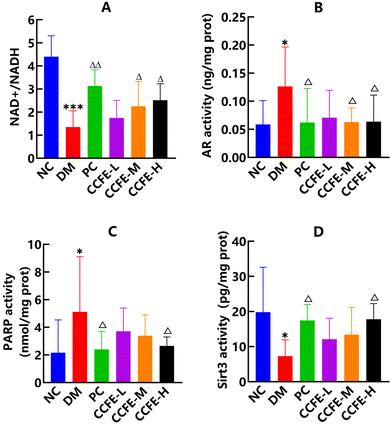 |
| Fig. 5 Effects of CCFE on NAD+/NADH redox balance in the pancreas of T2DM rats. (A) NAD+/NADH; (B) AR; (C) PARP; (D) Sirt3. *p < 0.05 and ***p < 0.001, compared with the NC group; Δp < 0.05 and ΔΔp < 0.01, compared with the DM control group. NC: normal control group, DM: diabetic model control group, PC: positive control group, CCFE-(L, M, H): (low, middle, high) dose CCFE-treated group. | |
NAD+/NADH redox imbalance is believed to mainly originate from two enzyme systems activated by persistent hyperglycemia. One reaction is the polyol pathway, including aldose reductase (AR) and sorbitol dehydrogenase. This pathway involves two consecutive reactions in which AR catalyzes the reduction of glucose to sorbitol, which is further oxidized to fructose by sorbitol dehydrogenase and generates NADH from NADPH via NAD+36Fig. 5B shows that AR activity, the rate-limiting enzyme of the polyol pathway,37 was indeed higher (p < 0.05) in the pancreas of the DM control group compared to that in the NC group. After administration of CCFE, the AR activity in the pancreas of the CCFE-M and CCFE-H groups was dramatically decreased (p < 0.05) compared with that in the DM control group, indicating that the polyol pathway in the pancreas of T2DM rats was suppressed by CCFE, likely resulting in a reduction in NAD+ consumption (Fig. 6). Additionally, Huang et al.38 found that Phellinus merrillii materials had inhibitory effects in vitro against R-glucosidase and rat lens AR. Kato et al.39 reported that a dietary supplement of ginger extract as an AR inhibitor would contribute to the protection against or improvement in diabetic complications.
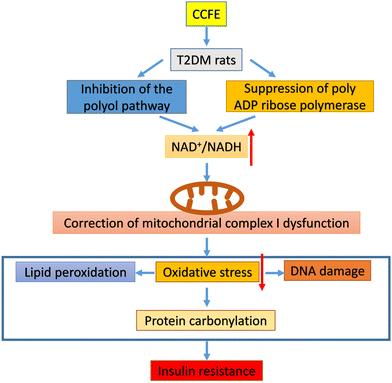 |
| Fig. 6 Schematic showing the potential mechanism of correcting complex I dysfunction by CCFE. CCFE suppressed the up-regulation of the polyol pathway and poly ADP ribose polymerase in the pancreas to alleviate NAD+/NADH redox imbalance. Additionally, CCFE reduced oxidative stress by lowering lipid peroxidation and protein carbonylation. | |
The second pathway is the PARP pathway, which can be overactivated due to DNA damage caused by hyperglycemia, resulting in the potential depletion of NAD+, which would further perturb the NAD+/NADH redox balance.40Fig. 5C demonstrates that PARP activity in the pancreas of the DM control group was greatly up-regulated (p < 0.05) compared with that in the NC group. After treatment with CCFE, the PARP activity in the pancreas of the CCFE-H group was significantly repressed (p < 0.05) compared with that in the DM control group, indicating that the reduction in NAD+ consumption and improvement in the NAD+/NADH redox imbalance in the pancreas of T2DM rats were achieved by CCFE (Fig. 6). Wu et al.41 reported that the PARP-1 protein content was upregulated in STZ-induced diabetic pancreas. Several pharmacological classes of compounds, such as 3-aminobenzamide and nicotinamide, have been shown to inhibit the catalytic activity of PARP.42
Moreover, Sirt3 is an NAD+-dependent deacetylase that is involved in redox signaling and metabolic control and is known to be downregulated when the level of NAD+ is low.43,44 Increasing the NAD+ levels through various strategies could enhance the activity of sirtuins, improve organ function and increase longevity.45 Li et al.46 also reported that the modulation of adipose tissue remodeling by activating Sirt3 could contribute to the antihyperlipidemic and antihyperglycemic effects of berberine. In this study, as shown in Fig. 5D, the Sirt3 activity in the pancreas of the DM control group was decreased (p < 0.05) compared with that in the NC group. After treatment with CCFE, a great increase in the Sirt3 activity (p < 0.05) in the pancreas of the CCFE-H group was found compared with that in the DM control group, suggesting that CCFE was beneficial for upregulating Sirt3 activity in the pancreas of T2DM rats due to the increase in the NAD+ content.
3.5 The effects of CCFE on complexes of the mitochondrial electron transport chain in the pancreas of T2DM rats
Complex I is a major site for NAD+ recycling from NADH, and pancreatic mitochondrial complex I activity is elevated in diabetes.41 The results in Fig. 7A show that complex I activity in the pancreas of the DM control group was tremendously upregulated (p < 0.01) compared with that in the NC group, suggesting that complex I was dysfunctional in the pancreas of T2DM rats, which meant to handle the additional amount of NADH likely produced by the activated polyol pathway. In addition, the mitochondrial complex I activity was upregulated in the livers of diabetic mice and in the heart tissue of type 1 diabetes mellitus mice.47,48 After treatment with CCFE, complex I activity in the pancreas of the CCFE-M and CCFE-H groups decreased significantly (p < 0.05) compared with that in the DM control group, demonstrating that dysfunction of complex I in the pancreas of T2DM rats could be corrected by CCFE. Iglesias et al.49 also confirmed that catechin inhibited heart mitochondrial complex I in rats and that extracts of Ta-ermi exhibited an inhibitory effect on complex I activity increases in the pancreas of DM rats.50
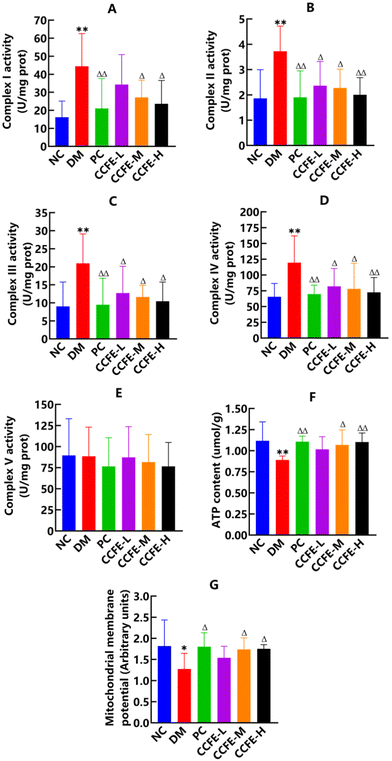 |
| Fig. 7 Effects of CCFE on complexes of the mitochondrial electron transport chain in the pancreas of T2DM rats. (A) Complex I; (B) Complex II; (C) Complex III; (D) Complex IV; (E) Complex V; (F) ATP; (G) Mitochondrial membrane potential. **p < 0.01, compared with the NC group; Δp < 0.05 and ΔΔp < 0.01, compared with the DM control group. NC: normal control group, DM: diabetic model control group, PC: positive control group, CCFE-(L, M, H): (low, middle, high) dose CCFE-treated group. | |
Then, we investigated the effects of CCFE on mitochondrial complexes II to V activities in the pancreas of T2DM rats. Fig. 7B–E show that the activities of complexes II to IV in the pancreas of the DM control group were significantly higher (p < 0.01), while complex V activity demonstrated no detectable changes compared with the activities of the NC group, which was similar to a previous study on the lungs of diabetic rats.51 Moreover, the NADH content in the pancreas of the DM control group was dramatically increased (p < 0.01) compared with that in the NC group. These results indicated that there was an enhanced electron transport by excess NADH in diabetes. Moreover, Fig. 7F and G show that a marked decrease in the ATP content (p < 0.01) and mitochondrial membrane potential level (p < 0.05) were observed in the pancreas of the DM control group compared with the NC group. Our results suggested that the up-regulated activities of complexes I to IV were not related to ATP production. Rather, more electrons were diverted to, or leaked, likely leading to ROS production and oxidative stress in T2DM rats. After treatment with CCFE, compared with the DM control group, the activities of complexes II to IV in the pancreas of CCFE treatment groups decreased significantly (p < 0.05), while the ATP content and mitochondrial membrane potential level were elevated significantly (p < 0.05) in the pancreas of the CCFE-M and CCFE-H groups. Collectively, CCFE downregulated the levels of complexes I to IV and upregulated the ATP content and mitochondrial membrane potential in the pancreas of T2DM rats.
3.6 Effects of CCFE on oxidative stress in T2DM rats
A study showed that the upregulation of the mitochondrial electron transport chain could lead to increased ROS production and oxidative stress in hyperglycemia.52 In the present study, our results showed that the H2O2 content (Fig. 8A) and MDA content (Fig. 8B) in serum and the protein carbonylation content (Fig. 8C) in the pancreas were all increased (p < 0.05) and that the GSH-Px activity (Fig. 8D) and SOD activity (Fig. 8E) in serum were both decreased (p < 0.01) in the DM control group compared with the NC group, indicating that the overflow of NADH via complex I was partially used for leak respiration and that the increase contributed to oxidative stress. In addition, an increase in the MDA content and a decrease in the SOD activity were reported by An et al.53 and a decrease in the GSH-Px activity was observed in diabetes.54 Diabetic rats had a higher protein carbonylation content than non-diabetic rats, as described by Jang et al.55 and Cumaoglu et al.56
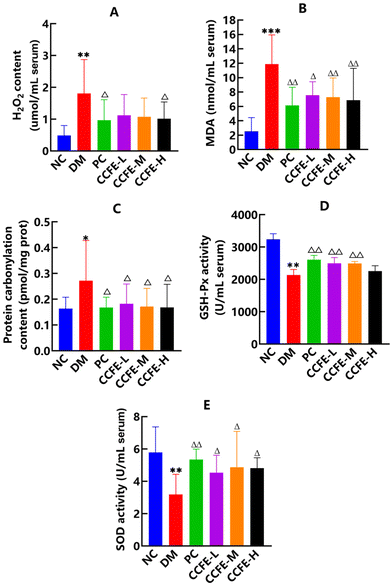 |
| Fig. 8 Effects of CCFE on oxidative stress in T2DM rats. (A) H2O2; (B) MDA; (C) protein carbonylation; (D) GSH-Px; (E) SOD. *p < 0.05, **p < 0.01 and ***p < 0.001, compared with the NC group; Δp < 0.05 and ΔΔp < 0.01, compared with the DM control group. NC: normal control group, DM: diabetic model control group, PC: positive control group, CCFE-(L, M, H): (low, middle, high) dose CCFE-treated group. | |
After the treatment with CCFE, compared with levels in the DM control group, the MDA content in the serum and the protein carbonylation content in the pancreas were markedly decreased (p < 0.05), and the SOD activity in the serum was greatly increased (p < 0.05) in the CCFE treatment groups. Furthermore, the H2O2 content in the serum of the CCFE-H treatment group was decreased significantly (p < 0.05) and the GSH-Px activity in the serum of the CCFE-L and CCFE-M treatment groups was markedly increased (p < 0.01). These results indicated that oxidative stress was potently attenuated in T2DM rats by CCFE administration. Some studies have shown decreased MDA levels and increased SOD levels in Duvalia corderoyi extract-treated diabetic rats,57 and quercetin could prevent oxidative stress in STZ-induced diabetic rats.58 Tabassum et al.59 considered that catechin prevented protein carbonylation in the liver. Protein carbonylation in T2DM rats was evidently suppressed by Aronia melanocarpa berry extract supplementation.60
4. Conclusions
In conclusion, we presented evidence that the aberrant activity of complex I in the pancreas of T2DM rats, which was upregulated by diabetic hyperglycemia, was reduced in response to the NAD+/NADH redox imbalance caused by the overproduction of NADH resulting from the activation of the polyol pathway and the upregulation of PARP activity, which were ameliorated by chickpea flavonoids. Furthermore, the upregulated activities of complexes I to IV due to NAD+/NADH redox imbalance and oxidative stress were decreased, while ATP production in the pancreas of T2DM rats was increased by the chickpea flavonoid treatment. These results, together with the findings of the decreased activities of AR and PARP and the increased activity of Sirt3 in the pancreas of T2DM rats, provide insights into the mechanisms of correcting the NAD+/NADH redox imbalance and complex I dysfunction in the pancreas of T2DM rats by chickpea flavonoids. In summary, chickpea flavonoids could be used as a potential natural product for preventing diabetes and its complications.
Abbreviations
CCFE | Crude chickpea flavonoid extract |
STZ | Streptozotocin |
HFD | High-fat diet |
T2DM | Type 2 diabetes mellitus |
IP | Intraperitoneal |
NC | Normal control group |
DM | Diabetic model control group |
PC | Positive control group |
CCFE-L | Low dose CCFE-treated group |
CCFE-M | Middle dose CCFE-treated group |
CCFE-H | High dose CCFE-treated group |
FBG | Fasting blood glucose |
IR | Insulin resistance |
TG | Triglyceride |
HDLC | High-density lipoprotein |
TC | Total cholesterol |
LDLC | Low-density lipoprotein |
AR | Aldose reductase |
PARP | Poly ADP ribose polymerase |
Sirt3 | Sirtuin 3 |
MDA | Malondialdehyde |
SOD | Superoxide dismutase |
GSH-Px | Glutathione peroxidase |
H2O2 | Hydrogen peroxide |
Author contributions
Yinghua Fu: conceptualization, methodology, validation, resources, writing-review and editing, supervision, project administration, funding acquisition; Zhenglei Li: software, formal analysis, investigation, data curation, writing-original draft preparation; Shiqi Xiao: formal analysis, investigation, data curation, writing-original draft preparation; Caiyun Zhao: investigation; Keqiang Zhou: investigation; Shenyi Cao: investigation.
Conflicts of interest
There are no conflicts of interest to declare.
Acknowledgements
This work was supported by the National Natural Science Foundation of China (No. 31960089), Natural Science Foundation of Xinjiang (No. 2018D01C058). We would like to thank the Experimental Animal Facility of the Xinjiang Medical University for providing rats specimens and technical support.
References
- S. Amanat, S. Ghahri, A. Dianatinasab, M. Fararouei and M. Dianatinasab, Exercise and type 2 diabetes, Adv. Exp. Med. Biol., 2020, 1228, 91–105 CrossRef CAS PubMed.
- P. Aschner, Insulin therapy in type 2 diabetes, Am. J. Ther., 2020, 27, e79–e90 CrossRef PubMed.
- M. Eguilaz, M. A. Batlle, B. Morentin, R. San-Cristóbal, S. Pérez-Díez, S. Navas-Carretero and J. A. Martínez, Alimentary and lifestyle changes as a strategy in the prevention of metabolic syndrome and diabetes mellitus type 2: milestones and perspectives, An. Sist. Sanit. Navar., 2016, 39, 269–289 CrossRef.
- Y. B. Wang, N. Shivappa, J. R. Hébert, A. J. Page, T. K. Gill and Y. A. Melaku, Association between dietary inflammatory index, dietary patterns, plant-based dietary index and the risk of obesity, Nutrients, 2021, 13, 1536 CrossRef CAS.
- M. Pearce, A. Fanidi, T. R. P. Bishop, S. J. Sharp, F. Imamura, S. Dietrich, T. Akbaraly, M. Bes-Rastrollo, J. W. J. Beulens and L. Byberg,
et al., Associations of total legume, pulse, and soy consumption with incident type 2 diabetes: federated meta-analysis of 27 studies from diverse world regions, J. Nutr., 2021, 151, 1231–1240 CrossRef.
- G. Pestoni, A. Riedl, T. A. Breuninger, N. Wawro, J. P. Krieger, C. Meisinger, W. Rathmann, B. Thorand, C. Harris, A. Peters, S. Rohrmann and J. Linseisen, Association between dietary patterns and prediabetes, undetected diabetes or clinically diagnosed diabetes: results from the KORA FF4 study, Eur. J. Nutr., 2021, 60, 2331–2341 CrossRef CAS PubMed.
- N. R. Mostacero, N. M. V. Castelli, M. I. Barolo, S. L. Amigot, C. L. Fulgueira and S. N. López, Fungal endophytes in peperomia obtusifolia and their potential as inhibitors of chickpea fungal pathogens, World J. Microbiol. Biotechnol., 2021, 4(37), 14 CrossRef PubMed.
- R. Chibbar, P. Ambigaipalan and R. Hoover, Review: molecular diversity in pulse seed starch and complex carbohydrates and its role in human nutrition and health, Cereal Chem., 2010, 87, 342–352 CrossRef CAS.
- A. K. Jukanti, P. M. Gaur, C. L. Gowda and R. N. Chibbar, Nutritional quality and health benefits of chickpea (Cicer arietinum L.): a review, Br. J. Nutr., 2012, 108, S11–S26 CrossRef CAS PubMed.
- V. Y. Waisundara, A. Hsu, K. H. Tan and D. Huang, Baicalin improves antioxidant status of streptozotocin-induced diabetic wistar rats, J. Agric. Food Chem., 2009, 57, 4096–4102 CrossRef CAS PubMed.
- K. Yamagata, Soy isoflavones inhibit endothelial cell dysfunction and prevent cardiovascular disease, J. Cardiovasc. Pharmacol., 2019, 74, 201–209 CrossRef CAS PubMed.
- C. Nagata, Soy intake and chronic disease risk: findings from prospective cohort studies in Japan, Eur. J. Clin. Nutr., 2021, 75, 890–901 CrossRef.
- M. J. Oza and Y. A. Kulkarni, Formononetin treatment in type 2 diabetic rats reduces insulin resistance and hyperglycemia, Front. Pharmacol., 2018, 9, 739 CrossRef.
- R. Azizi, M. T. Goodarzi and Z. Salemi, Effect of biochanin a on serum visfatin level of streptozocin-induced diabetic rats, Iran. Red. Crescent Med. J., 2014, 16, e15424 Search PubMed.
- C. Huang, D. Pang, Q. Luo, X. Chen, Q. Gao, L. Shi, W. Liu, Y. Zou, L. Li and Z. Chen, Soy isoflavones regulate lipid metabolism through an AKT/mTORC1 pathway in diet-induced obesity (DIO) male rats, Molecules, 2016, 21, 586 CrossRef PubMed.
- J. Hirst, Mitochondrial complex I, Annu. Rev. Biochem., 2013, 82, 551–575 CrossRef CAS PubMed.
- C. Wirth, U. Brandt, C. Hunte and V. Zickermann, Structure and function of mitochondrial complex I, Biochim. Biophys. Acta, 2016, 1857, 902–914 CrossRef CAS PubMed.
- Q. Li, Y. C. Hwang, R. Ananthakrishnan, P. J. Oates, D. Guberski and R. Ramasamy, Polyol pathway and modulation of ischemia-reperfusion injury in type 2 diabetic BBZ rat hearts, Cardiovasc. Diabetol., 2008, 7, 33 CrossRef PubMed.
- R. D. Semba, H. Huang, G. A. Lutty, J. E. Van Eyk and G. W. Hart, The role of O-GlcNAc signaling in the pathogenesis of diabetic retinopathy, Proteomics: Clin. Appl., 2014, 8, 218–231 CAS.
- W. Ying, NAD+/NADH and NADP+/NADPH in cellular functions and cell death: regulation and biological consequences, Antioxid. Redox Signaling, 2008, 10, 179–206 CrossRef CAS PubMed.
- C. Escande, V. Nin, N. L. Price, V. Capellini, A. P. Gomes, M. T. Barbosa, L. O'Neil, T. A. White, D. A. Sinclair and E. N. Chini, Flavonoid apigenin is an inhibitor of the NAD+ ase CD38: implications for cellular NAD+ metabolism, protein acetylation, and treatment of metabolic syndrome, Diabetes, 2013, 62, 1084–1093 CrossRef CAS PubMed.
- W. Ying, G. Wei, D. Wang, Q. Wang, X. Tang, J. Shi, P. Zhang and H. Lu, Intranasal administration with NAD+ profoundly decreases brain injury in a rat model of transient focal ischemia, Front. Biosci., 2007, 12, 2728–2734 CrossRef CAS PubMed.
- J. Chiu, B. Y. Xu, S. Chen, B. Feng and S. Chakrabarti, Oxidative stress-induced, poly (ADP-ribose) polymerase-dependent upregulation of ET-1 expression in chronic diabetic complications, Can. J. Physiol. Pharmacol., 2008, 86, 365–372 CrossRef CAS PubMed.
- X. Luo, R. Li and L. J. Yan, Roles of pyruvate, NADH, and mitochondrial complex I in redox balance and imbalance in β cell function and dysfunction, J. Diabetes Res., 2015, 2015, 512618 Search PubMed.
- J. Sun, L. An, Z. Zhang, N. Zhao, G. Yuan and P. Du, Extraction methods and sedative–hypnotic effects of polysaccharide and total flavonoids of Cordyceps militaris, Biotechnol. Biotechnol. Equip., 2018, 32, 498–505 CrossRef CAS.
- L. K. Park, E. J. Parks, R. J. Pettit-Mee, M. L. Woodford, T. Ghiarone, J. A. Smith, A. R. K. Sales, L. A. Martinez-Lemus, C. Manrique-Acevedo and J. Padilla, Skeletal muscle microvascular insulin resistance in type 2 diabetes is not improved by eight weeks of regular walking, J. Appl. Physiol., 2020, 129, 283–296 CrossRef CAS PubMed.
- W. Tang, S. Li, Y. Liu, M. T. Huang and C. T. Ho, Anti-diabetic activity of chemically profiled green tea and black tea extracts in a type 2 diabetes mice model via different mechanisms, J. Funct. Foods, 2013, 5, 1784–1793 CrossRef CAS.
- Y. M. Tian, Y. Liu, S. Wang, Y. Dong, T. Su, H. J. Ma and Y. Zhang, Anti-diabetes effect of chronic intermittent hypobaric hypoxia through improving liver insulin resistance in diabetic rats, Life Sci., 2016, 150, 1–7 CrossRef CAS PubMed.
- S. J. Bhathena and M. T. Velasquez, Beneficial role of dietary phytoestrogens in obesity and diabetes, Am. J. Clin. Nutr., 2002, 76, 1191–1201 CrossRef CAS PubMed.
- M. K. Farsani, E. Amraie, P. Kavian and M. Keshvari, Effects of aqueous extract of alfalfa on hyperglycemia and dyslipidemia in alloxan-induced diabetic Wistar rats, Interv. Med. Appl. Sci., 2016, 8, 103–108 Search PubMed.
- J. F. Cui, H. Ye, Y. J. Zhu, Y. P. Li, J. F. Wang and P. Wang, Characterization and hypoglycemic activity of a rhamnan-type sulfated polysaccharide derivative, Mar. Drugs, 2019, 17, 21 CrossRef CAS PubMed.
- Y. A. Chiao, A. D. Chakraborty, C. M. Light, R. Tian, J. Sadoshima, X. Shi, H. Gu and C. F. Lee, NAD+ redox imbalance in the heart exacerbates diabetic cardiomyopathy, Circ. Heart Fail., 2021, 14, e008170 CrossRef PubMed.
- J. S. Teodoro, A. P. Rolo and C. M. Palmeira, The NAD ratio redox paradox: why does too much reductive power cause oxidative stress, Toxicol. Mech. Methods, 2013, 23, 297–302 CrossRef CAS PubMed.
- Y. Ogura, M. Kitada, J. Xu, I. Monno and D. Koya, CD38 inhibition by apigenin ameliorates mitochondrial oxidative stress through restoration of the intracellular NAD+/NADH ratio and Sirt3 activity in renal tubular cells in diabetic rats, Aging, 2020, 12, 11325–11336 CrossRef CAS PubMed.
- G. Luo, B. Huang, X. Qiu, L. Xiao, N. Wang, Q. Gao, W. Yang and L. Hao, Resveratrol attenuates excessive ethanol exposure induced insulin resistance in rats via improving NAD+/NADH ratio, Mol. Nutr. Food Res., 2017, 61, 170087 CrossRef PubMed.
- J. M. Forbes and M. E. Cooper, Mechanisms of diabetic complications, Physiol. Rev., 2013, 93, 137–188 CrossRef CAS PubMed.
- S. S. K. Chung and S. K. Chung, Aldose reductase in diabetic microvascular complications, Curr. Drug. Targets, 2005, 6, 475–486 CrossRef CAS PubMed.
- G. J. Huang, W. T. Hsieh, H. Y. Chang, S. S. Huang, Y. C. Lin and Y. H. Kuo,
α-Glucosidase and aldose reductase inhibitory activities from the fruiting body of Phellinus merrillii, J. Agric. Food Chem., 2011, 59, 5702–5706 CrossRef CAS PubMed.
- A. Kato, Y. Higuchi, H. Goto, H. Kizu, T. Okamoto, N. Asano, J. Hollinshead, R. J. Nash and I. Adachi, Inhibitory effects of zingiber officinale roscoe derived components on aldose reductase activity in vitro and in vivo, J. Agric. Food Chem., 2006, 54, 6640–6644 CrossRef CAS PubMed.
- K. Sas, E. Szabó and L. Vécsei, Mitochondria, oxidative stress and the kynurenine system, with a focus on ageing and neuroprotection, Molecules, 2018, 23, 191 CrossRef PubMed.
- J. Z. Wu, X. T. Luo, N. Thangthaeng, N. Sumien, Z. L. Chen, M. A. Rutledge, S. Q. Jing, M. J. Forster and L. J. Yan, Pancreatic mitochondrial complex I exhibits aberrant hyperactivity in diabetes, Biochem. Biophy. Rep., 2017, 11, 119–129 Search PubMed.
- P. Jagtap and C. Szabó, Poly (ADP-ribose) polymerase and the therapeutic effects of its inhibitors, Nat. Rev. Drug Discovery, 2005, 4, 421–440 CrossRef CAS PubMed.
- S. Vedantham, D. Thiagarajan, R. Ananthakrishnan, L. Wang, R. Rosario, Y. S. Zou, I. Goldberg, S. F. Yan, A. M. Schmidt and R. Ramasamy, Aldose reductase drives hyperacetylation of Egr-1 in hyperglycemia and consequent upregulation of proinflammatory and prothrombotic signals, Diabetes, 2014, 63, 761–774 CrossRef CAS PubMed.
- R. Cerutti, E. Pirinen, C. Lamperti, S. Marchet, A. A. Sauve, W. Li, V. Leoni, E. A. Schon, F. Dantzer, J. Auwerx, C. Viscomi and M. Zeviani, NAD+-dependent activation of Sirt1 corrects the phenotype in a mouse model of mitochondrial disease, Cell. Metab., 2014, 19, 1042–1049 CrossRef CAS PubMed.
- M. S. Bonkowski and D. A. Sinclair, Slowing ageing by design: the rise of NAD+ and sirtuin-activating compounds, Nat. Rev. Mol. Cell Biol., 2016, 17, 679–690 CrossRef CAS PubMed.
- D. Li, C. Yang, J. Z. Zhu, E. Lopez, T. Zhang, Q. Tong, C. Peng and L. G. Lin, Berberine remodels adipose tissue to attenuate metabolic disorders by activating sirtuin 3, Acta Pharmacol. Sin., 2021, 1–14 Search PubMed.
- M. Alimujiang, X. Y. Yu, M. Y. Yu, W. L. Hou, Z. H. Yan, Y. Yang, Y. Q. Bao and J. Yin, Enhanced liver but not muscle OXPHOS in diabetes and reduced glucose output by complex I inhibition, J. Cell. Mol. Med., 2020, 24, 5758–5771 CrossRef CAS PubMed.
- M. Wu, W. Chen, S. Zhang, S. Huang, A. Zhang, Y. Zhang and Z. Jia, Rotenone protects against β-cell apoptosis and attenuates type 1 diabetes mellitus, Apoptosis, 2019, 24, 879–891 CrossRef CAS PubMed.
- D. E. Iglesias, S. S. Bombicino, A. Boveris and L. B. Valdez, (+)-Catechin inhibits heart mitochondrial complex I and nitric oxide synthase: functional consequences on membrane potential and hydrogen peroxide production, Food Funct., 2019, 10, 2528–2537 RSC.
- S. Jing, Z. Zhao, J. Wu and L. J. Yan, Antioxidative and hypoglycemic effect of ta-ermi extracts on streptozotocin-induced diabetes, Diabetes, Metab. Syndr. Obes.: Targets Ther., 2020, 13, 2147–2155 CrossRef CAS PubMed.
- J. Wu, Z. Jin and L. J. Yan, Redox imbalance and mitochondrial abnormalities in the diabetic lung, Redox Biol., 2017, 11, 51–59 CrossRef CAS PubMed.
- S. K. Panigrahy, R. Bhatt and A. Kumar, Reactive oxygen species: sources, consequences and targeted therapy in type 2 diabetes, J. Drug Targeting, 2017, 25, 93–101 CrossRef CAS PubMed.
- S. An, D. Niu, T. Wang, B. Han, C. He, X. Yang, H. Sun, K. Zhao, J. Kang and X. Xue, Total saponins isolated from Corni Fructus via ultrasonic microwave-assisted extraction attenuate diabetes in mice, Foods, 2021, 10, 670 CrossRef CAS PubMed.
- S. Y. Ou, G. M. Jackson, X. Jiao, J. Chen, J. Z. Wu and X. S. Huang, Protection against oxidative stress in diabetic rats by wheat bran feruloyl oligosaccharides, J. Agric. Food Chem., 2007, 55, 3191–3195 CrossRef CAS PubMed.
- Y. Y. Jang, J. H. Song, Y. K. Shin, E. S. Han and C. S. Lee, Protective effect of boldine on oxidative mitochondrial damage in streptozotocin-induced diabetic rats, Pharmacol. Res., 2000, 42, 361–371 CrossRef CAS PubMed.
- A. Cumaoglu, C. Cevik, L. Rackova, N. Ari and C. Karasu, Effects of antioxidant stobadine on protein carbonylation, advanced oxidation protein products and reductive capacity of liver in streptozotocin-diabetic rats: role of oxidative/nitrosative stress, Biofactors, 2007, 30, 171–178 CrossRef CAS PubMed.
- N. A. Alfaris, G. M. Alshammari, M. M. Alsayadi, M. A. Alfaris and M. A. Yahya, Concise anti-oxidative stress defence effects of Duvalia corderoyi, in the liver and kidney tissues of streptozotocin-induced diabetic rats, J. Taibah Univ. Sci., 2020, 14, 524–533 CrossRef.
- L. Tang, K. Li, Y. Zhang, H. Li, A. Li, Y. Xu and B. Wei, Quercetin liposomes ameliorate streptozotocin-induced diabetic nephropathy in diabetic rats, Sci. Rep., 2020, 10, 2440 CrossRef CAS PubMed.
- H. Tabassum, S. Parvez, H. Rehman, B. D. Banerjee and S. Raisuddin, Catechin as an antioxidant in liver mitochondrial toxicity: inhibition of tamoxifen-induced protein oxidation and lipid peroxidation, J. Biochem. Mol. Toxicol., 2007, 21, 110–117 CrossRef CAS PubMed.
- J. J. Mu, G. Xin, B. Zhang, Y. H. Wang, C. Ning and X. J. Meng, Beneficial effects of Aronia melanocarpa berry extract on hepatic insulin resistance in type 2 diabetes mellitus rats, J. Food Sci., 2020, 85, 1307–1318 CrossRef CAS PubMed.
Footnote |
† These authors equally contributed to the work. |
|
This journal is © The Royal Society of Chemistry 2022 |