DOI:
10.1039/D1FO02752B
(Paper)
Food Funct., 2022,
13, 102-112
Saccharomyces boulardii alleviates DSS-induced intestinal barrier dysfunction and inflammation in humanized mice†
Received
21st August 2021
, Accepted 11th November 2021
First published on 16th November 2021
Abstract
Recent clinical studies have demonstrated a beneficial effect of Saccharomyces boulardii (S. boulardii) in inflammatory bowel disease (IBD). However, the underlying mechanisms remain poorly defined. In this study, we investigated the modulating effect of S. boulardii on the intestinal microbiota in humanized mice with dextran sulfate sodium (DSS)-induced colitis. The mice were fed an S. boulardii-supplement diet for 16 days before DSS treatment. The results showed that feeding S. boulardii significantly ameliorated the colon damage and regulated inflammatory responses by modulating the cytokine profile. These changes were found to be associated with an altered microbiome composition and short-chain fatty acid (SCFA) metabolism. Further analysis demonstrated that S. boulardii-derived polysaccharides and polypeptides promoted the growth of certain probiotics and increased the microbial metabolite SCFAs levels. Overall, these findings demonstrated the role of S. boulardii as a potential gut microbiota modulator to prevent and treat IBD.
Introduction
Inflammatory bowel disease, which is characterized by chronic inflammation of the gastrointestinal tract, has evolved into a global disease.1 Although a number of medical treatments, such as steroids, immunomodulators, and antibodies, are available to IBD patients, low treatment responses and high incidence of recurrence highlight the need for novel therapeutics.2
For a long time, the gut microbiota has been considered to play an important role in the maintenance of the health of the host. Dysbacteriosis has been suggested as pivotal in promoting epithelial injury and inflammation.3 Clinical data have identified a significant alteration of the composition and function of gut microbiota in IBD patients.4 Compared with those of healthy individuals, the gut microbiota of IBD patients has been characterized by a lower microbial diversity, a reduced growth of probiotic bacteria such as Bifidobacterium and Lactobacillus5 and an expansion of inflammation-related bacteria such as Clostridium difficile.6 Although the precise pathogenesis of IBD is currently not fully understood, animal studies have shown that the gut microbiota is indispensable for pathogenesis in most colitis models.3 Thus, gut microbiota should be considered as a new therapeutic target in IBD.
The fungal community is an important part of mammalian microbiota and has been linked with the inflammatory bowel conditions.7Saccharomyces was found to be the most abundant fungal genus in healthy individuals, followed by Malassezia and Candida.8S. boulardii is the only probiotic of this genus widely used in clinical practice, mainly for prophylaxis and treatment of a variety of diarrheal diseases.9,10 Findings from clinical studies have suggested that S. boulardii has striking therapeutic benefits for IBD.11,12 Experimental evidence in intestinal inflammatory rodent models also showed the anti-inflammatory effects of this probiotic.13,14 However, little is known about the underlying mechanisms.
The cell wall of yeast cells is rich in polysaccharides and polypeptides. Some yeast polysaccharides such as β-glucan and mannan have immune-regulatory properties.15 Animal studies have suggested that β-glucans in food modify the host gut microbiota and may play a role in DSS-induced colitis.16–18 In an interleukin-10 knockout mouse model, dietary β-glucan reduced the inflammation associated with IBD.19
To date, little attention has been paid to the impact of S. boulardii and S. boulardii-derived molecules on the gut microbiota in intestinal inflammation, which could contribute to its beneficial effects. In the present work, we aimed to explore the role of S. boulardii in the gut microbial ecosystem and uncover the potential mechanisms of the intestinal anti-inflammatory activity of S. boulardii.
Materials and methods
Strain preparation and animals
S. boulardii strain and S. boulardii-derived molecules were obtained from Angel Nutritech, Co., Ltd (Hubei, China) (Table S1†). Sterile yeast was propagated by inoculation in yeast extract–peptone–dextrose (YEPD) broth under agitation at 30 °C. Bifidobacterium animalis BB12 and Lactobacillus reuteri M1011 were isolated and preserved in our laboratory. Clostridium leptum DSM 753 was purchased from DSMZ (Braunschweig, Germany). Bifidobacterium animalis BB12 and Lactobacillus reuteri M1011 were cultured at 37 °C in fresh sterile MRS broth, while Clostridium leptum DSM 753 was cultured in PYG medium (modified, DSMZ Medium 104).
Specific pathogen-free (SPF) C57BL/6J mice (6 weeks old, male) were purchased from the Hubei Province Center for Disease Control and Prevention (Wuhan, China). The mice were housed (no more than four per cage) under humidity- and temperature-controlled conditions and a 12-hour light/dark cycle with free access to food and water. All procedures strictly conformed to the Guide for the Care and Use of Laboratory Animals published by the United States National Institutes of Health.
Synthetic microbiota (SM) preparation
The 77 strains of culturable commensal human gut bacteria were purchased from companies or isolated from freshly collected fecal samples obtained from healthy individuals (Table S2†). Each strain was activated and cultured until the early stationary phase in an appropriate medium. All of the procedures were performed in an anaerobic chamber (Coy Laboratory Products) to maintain anaerobic conditions. Next, the cells of each strain were washed, resuspended in saline with a lyoprotectant, and lyophilized in a freeze dryer (Labconco Corporation, Kansas, MO, USA). The lyophilized bacterial powders were then separately suspended in saline to a concentration of 1 × 1011 CFU mL−1. SM solution for oral administration was prepared by mixing 77 bacterial suspensions in equal proportions.
Animal experiments
After a week of adaptive feeding, mice were randomly divided into three groups (n = 5): a regular diet (DSS) group, an SM-colonized group fed a regular diet (DSS + SM) and an SM-colonized group fed a S. boulardii-supplement diet (DSS + SM + S.b). Mice then received a cocktail of broad-spectrum antibiotics including vancomycin (0.1 g L−1), neomycin (0.2 g L−1), ampicillin (0.2 g L−1), and metronidazole (0.2 g L−1) in their drinking water20 for 7 days. After that, SM (1 × 109 CFU suspended in 100 μL of saline) was given daily to the mice in the DSS + SM group and DSS + SM + S.b group by oral administration for 7 days. For the DSS group, 100 μL of saline was administered daily to the mice. Meanwhile, mice from the DSS + SM + S.b group were fed a S. boulardii-supplement diet (created by mixing S. boulardii in finely crushed chow and drying as food pellets; 1 × 108 CFU of S. boulardii per mouse daily), while mice from the other two groups were fed a regular diet. 16 days later, colitis was induced in all the groups by adding 2.5% (w/v) DSS (Millipore, Billerica, MA, USA) to the drinking water for 12 days. Mice were sacrificed at the end of the treatment period. All animals received humane care and experimental protocols were carried out in accordance with the Guide for the Care and Use of Laboratory Animals of Huazhong University of Science and Technology, as approved by the Animal Care Committee of Hubei Province.
For each mouse, the body weight, presence of gross blood in feces, and stool consistency were evaluated and recorded daily by a blind observer. A score was assigned for each item, and the colitis disease activity index (DAI) was calculated (Table S3†). Once the treatment was complete, the animals were euthanized. Mice were then dissected, and their colons were removed aseptically for length measurement. The colon tissues were then collected immediately and stored at −80 °C until analysis.
Histological analysis
Partial colon tissues were fixed in 4% paraformaldehyde, processed into paraffin blocks, sectioned, and stained with H&E according to the manufacturer's instructions. Images of the stained sections were acquired by fluorescence microscopy (Nikon Eclipse CI, Japan). Colonic histological damage was observed under a light microscope and scored based on two subscores: cell infiltration (0–3, from rare inflammatory cells in the lamina propria to transmural extension of the infiltration of inflammatory cells) and tissue damage (0–3, from no mucosal damage to extensive mucosal damage and extension through deeper structures of the bowel wall).21 Six random optical fields per colon were taken and counted.
Gene expression in the colon tissues
RNA from the harvested colon tissues was extracted with TRIzol reagent (Invitrogen, USA). To generate cDNA, we used the HiScript II 1st Strand cDNA Synthesis Kit (Vazyme, China) with 2 μg of RNA for each sample. mRNA relative expression was measured using a CFX Connect Real-Time PCR Detection System (Bio-Rad). PCR was carried out with 10 μL of SYBR Green Master Mix (Yeasen, Shanghai, China), 2 μL of complementary DNA (cDNA), 0.4 μL of forward primer, 0.4 μL of reverse primer, and 7.2 μL of nuclease-free water. The samples were subjected to 40 cycles of amplification. Preincubation was for 30 seconds at 95 °C, followed by denaturation at 95 °C for 10 seconds, annealing at 58 °C for 20 seconds, and extension at 72 °C for 30 seconds. The primers used in the present study are listed in Table S4.†
Microbial DNA extraction and sequencing
Mouse fecal samples at the end point of DSS treatment were collected and frozen at −80 °C immediately after collection. Total genomic DNA from approximately 200 mg of stool was extracted using a QIAamp DNA Stool Mini Kit (Qiagen, Valencia, CA) according to the manufacturer's instructions. The V3–V4 region of the bacterial 16S ribosomal RNA (rRNA) genes was amplified by PCR with universal primers (338F, ACTCCTACGGGAGGCAGCAG; 806R, GGACTACHVGGGTWTCTAAT) and FastPfu polymerase. Amplicons were then purified by gel extraction (AxyPrep DNA Gel Extraction Kit, Axygen Biosciences, USA) and quantified using a QuantiFluor-ST (Promega, USA). The purified amplicons were pooled in equimolar concentrations, and paired-end sequencing was performed using an Illumina MiSeq platform (Illumina, San Diego, USA).
Targeted SCFAs quantitative analysis
Fecal samples collected at the end point of DSS treatment were used for SCFAs analysis. 50 mg of fecal contents was supplemented with 10 μL of 5 mol L−1 HCl and 200 μL of methyl tertiary butyl ether (MTBE). 50 μL of 500 μmol L−1 internal standards (3-methylpentanoic acid, Sigma-Aldrich) was added, and then the mixtures were vortexed for 10 min and sonicated for an additional 10 min at 4 °C. Next, the samples were centrifuged at 1000g at 4 °C for 10 min, followed by 20 min of rest at −20 °C. The extracted samples were then analyzed by a GC-MS method slightly modified from the references22,23 using a 5975C GC-MS system (Agilent Technologies, Santa Clara, CA). SCFAs standards were mixtures of acetate, propionate, and butyrate. All standards were purchased from Merck (Darmstadt, Germany).
In vitro growth assay
Bifidobacterium animalis, Lactobacillus reuteri and Clostridium leptum were activated and cultured at 37 °C overnight. Then, 1 mL of saturated O/N culture of these three strains was inoculated into 100 mL of an appropriate medium separately. The S. boulardii-supplement group received 107 CFU mL−1S. boulardii or 5 mg mL−1 polysaccharides and polypeptides at the same time. After culturing to the log phase, the bacterial solution of each strain was serially diluted 10-fold, and viable cells were enumerated using a quantitative plate count method. All experiments were performed under anaerobic conditions.
Statistical analysis
Statistical analysis was performed using GraphPad Prism 8 statistical software. Comparisons between two groups were performed using an unpaired two-tailed Student's t-test. One-way analysis of variance was used for comparisons of more than two groups. The results are presented as the mean ± SD. Differences were considered significant at *P < 0.05, **P < 0.01, ***P < 0.001, and ****P < 0.0001.
Results
S. boulardii supplementation eliminated DSS-induced colitis in a humanized mouse model
To gain a better understanding of the possible effects of the human intestinal microbiota, we designed an SM containing 77 species of culturable commensal human gut bacteria from the Culturable Genome Reference (CGR) (Table S2†).24 Antibiotic-treated mice received a daily oral gavage of SM (1 × 109 CFU in a volume of 100 μL saline) for a week. Meanwhile, 1 × 108 CFU S. boulardii was administered daily to the mice via diet. After 16 days, mice were challenged with 2.5% DSS in drinking water to induce colitis (Fig. 1A). Compared to the DSS group and DSS + SM group, the DSS + SM + S.b group showed a significantly lower DAI score and a higher trend of body weight (Fig. 1B and Fig. S1†). In addition, S. boulardii treatment prevented mucosal damage in the colon tissue, resulting in a decreased histological score in the mice (Fig. 1C). Moreover, the DSS + SM + S.b group showed relieved colon shortening compared to that of the DSS group and DSS + SM group (Fig. 1D). We next assessed typical pro-inflammatory and anti-inflammatory cytokines via biochemical analysis of the colon tissues. As shown in Fig. 1E, the expressions of the Th17-type cytokine tumor necrosis factor alpha (TNF-α) and IL-6 significantly decreased in the DSS + SM + S.b group compared to those of the other two groups. Treg-type anti-inflammatory cytokine IL-10 expression, on the other hand, significantly increased in the DSS + SM + S.b group. Other cytokines such as IL-18 and IL-22 showed no significant difference between these groups (Fig. 1E). Taken together, these findings suggested that S. boulardii may facilitate anti-inflammatory immune responses and ameliorate DSS-induced colitis.
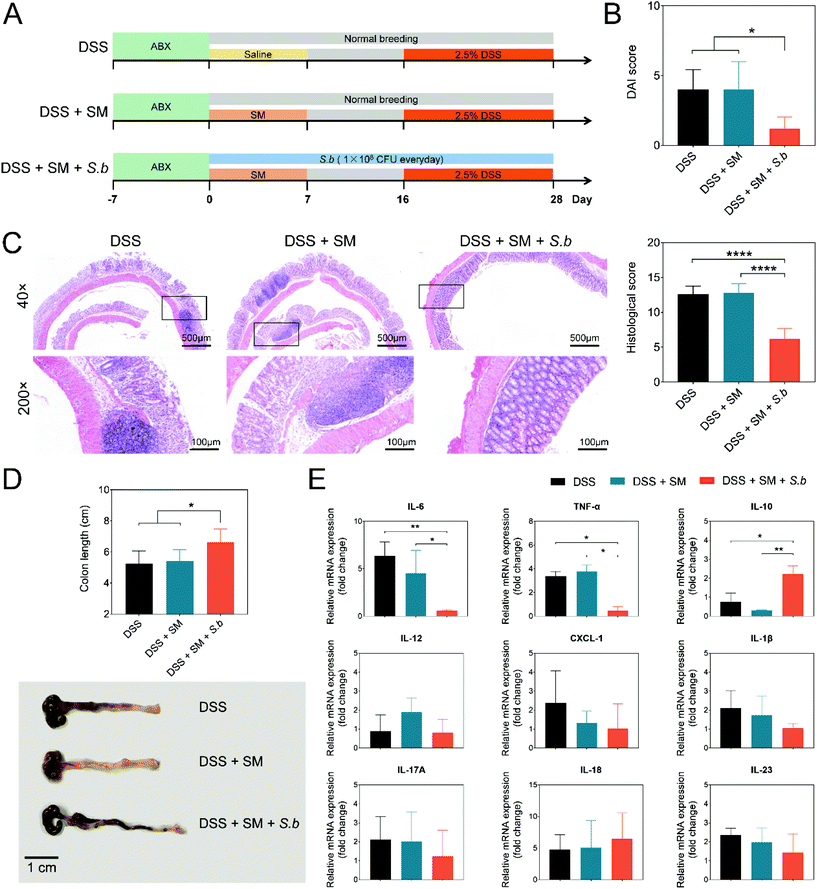 |
| Fig. 1
S. boulardii supplementation eliminates DSS-induced colitis in a humanized mouse model. (A) Schematic of the administration schedule. (B) Disease activity index (DAI) score. (C) Representative microscopic pictures of H&E staining (×40 and ×200 magnification). (D) Representative pictures of the gross colon appearance and colon length. (E) Cytokine expression levels in colon tissue homogenates were measured by real-time qPCR and normalized by the β-actin expression. Graph represents the mean of the fold expression of each cytokine with the expression level measured in the control animals (normal mice with no DSS treated) used as a reference and set to one. Mean values ± SDs are presented, and p values were calculated using an unpaired t-test; *p < 0.05 and **p < 0.01. Data are pooled from three independent experiments with n = 5 mice per group. | |
S. boulardii significantly altered the gut microbiota composition
To investigate the influence of S. boulardii supplementation on the gut microbiota composition, we collected mouse stools at the end point of DSS administration. Microbial DNA extraction and 16S rRNA gene sequencing were conducted. The alpha diversity revealed no significant differences among the groups (Fig. 2A). We also performed principal coordinate analysis (PCoA) on OTU levels to further examine the altered structure of the gut microbiota. The results clearly showed an apparent clustering separation among the three groups, suggesting that these gut communities differed markedly in their microbiota compositional structure (Fig. 2B). To further identify the specific bacteria altered by S. boulardii, which may play a role in colitis progression, we performed LEfSe analysis to identify differentially abundant bacterial communities between the DSS + SM group and DSS + SM + S.b group (Fig. 2C). We found that S. boulardii treated mice harbored distinctively higher abundances of the genera such as Prevotella, Lactobacillus, and Bifidobacterium. On the other hand, the abundances of the genera Akkermansia, Odoribacter, and Butyricimonas were lower in the DSS + SM + S.b group than in the DSS + SM group. Pearson correlation analysis showed that relative abundance of Lactobacillus and Bifidobacterium exhibited significant and negative correlations with the indicators of colitis, including the DAI score, weight loss, histological score and pro-inflammatory cytokine levels. On the other hand, the relative abundance of these two probiotics showed remarkable and positive correlations with the colon length and anti-inflammatory cytokine IL-10 level (Fig. 2D). These data indicated that supplementation with S. boulardii leads to global alterations of the commensal microbiota, which may dictate the status of intestinal inflammation.
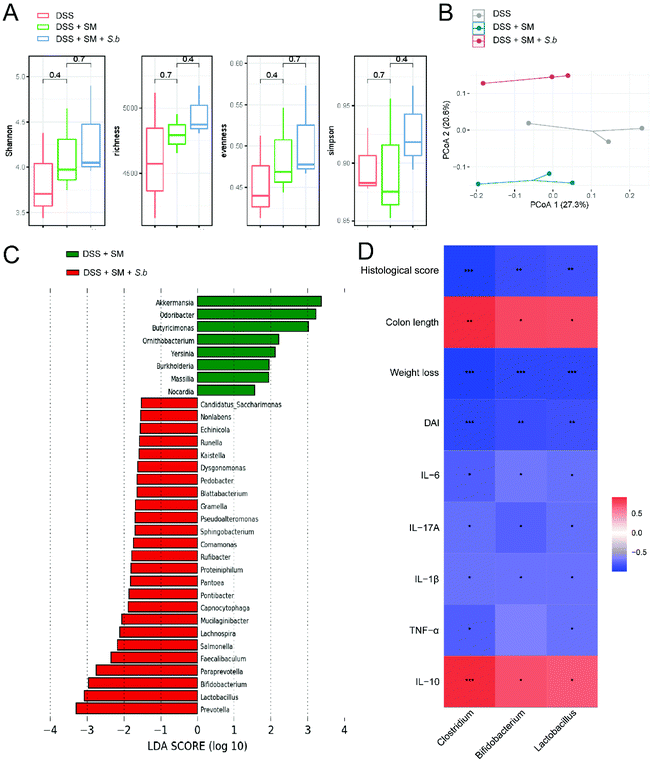 |
| Fig. 2
S. boulardii significantly altered the gut microbiota composition. (A) Alpha diversity boxplot analysis. (B) The principal coordinate analysis (PCoA) profile of microbial diversity (p = 0.007). (C) LDA score computed from features differentially abundant between the DSS + SM and DSS + SM + S.b groups. (D) Pearson correlation analysis. Significant values (p < 0.05 after FDR adjustment) are shown. Red and blue colors represent significant positive correlations and negative correlations. | |
S. boulardii increased the production of microbial metabolite SCFAs
The predominant genera in the S. boulardii-supplement group, Lactobacillus and Bifidobacterium, have been reported to generate SCFAs by fermentation of dietary fibers. SCFAs, specifically acetate, propionate, and butyrate, play crucial roles in the maintenance of intestinal homeostasis and the regulation of intestinal mucosal immunity.25 In the present work, the levels of acetate, propionate, and butyrate exhibited significant and negative correlations with the DAI score, weight loss, histological score, and pro-inflammatory cytokine levels, while showed remarkable and positive correlations with the colon length and anti-inflammatory cytokine IL-10 level (Fig. 3A).
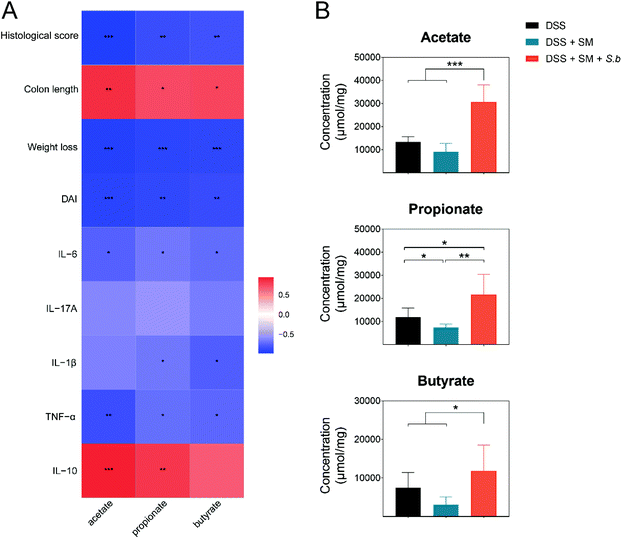 |
| Fig. 3
S. boulardii treatment increased the production of microbial metabolite SCFAs. (A) Pearson correlation analysis between the SCFAs and intestinal inflammatory-related index. Significant values (p < 0.05 after FDR adjustment) are shown. Red and blue colors represent significant positive correlations and negative correlations. (B) Fecal SCFAs concentrations in the DSS + SM and DSS + SM + S.b groups. (A and B) Mean values ± SDs are presented, and p values were calculated using unpaired t-tests; *p < 0.05, **p < 0.01, ***p < 0.001 and ****p < 0.0001. n = 5 mice per group. | |
Then, we measured the SCFAs level in fecal samples of all the groups by a targeted metabolomics assay. The levels of SCFAs in different groups are shown in Fig. 3B. The concentrations of SCFAs in the intestinal tract contents were all significantly increased in the DSS + SM + S.b group. Collectively, the increased levels of SCFAs in the S. boulardii treatment group have positive effects on colitis alleviation.
S. boulardii promoted the growth of certain probiotics and increased their production of SCFAs
Based on the results above, S. boulardii might facilitate the growth of certain probiotics and enhance their SCFAs production. To validate this conjecture, we performed an in vitro assay on Bifidobacterium and Lactobacillus, which we mentioned previously were enriched in the S. boulardii supplement group. Considering the great impact of Clostridium on gut SCFAs levels and its significant correlations with inflammation-related indicators (Fig. 2D), Clostridium leptum was also examined. Consistent with the in vivo experiment results, supplementation with S. boulardii promoted the growth of B. animalis, L. reuteri, and C. leptum (Fig. 4A). Then, the supernatant from each culture was filtered separately, and the concentration of SCFAs produced by each strain was also measured by GC-MS. In the S. boulardii-supplement group, B. animalis produced significantly more acetate and propionate, with no detectable butyrate (Fig. 4B). L. reuteri culture also showed a significantly higher concentration of acetate in the S. boulardii-supplement group, with no detectable propionate or butyrate (Fig. 4B). Interestingly, a significant decrease in C. leptum-produced acetate and significant increases in C. leptum-produced propionate and butyrate were observed in the S. boulardii supplement group (Fig. 4B). These data suggested that S. boulardii can promote the growth of certain probiotics and increase their SCFAs production.
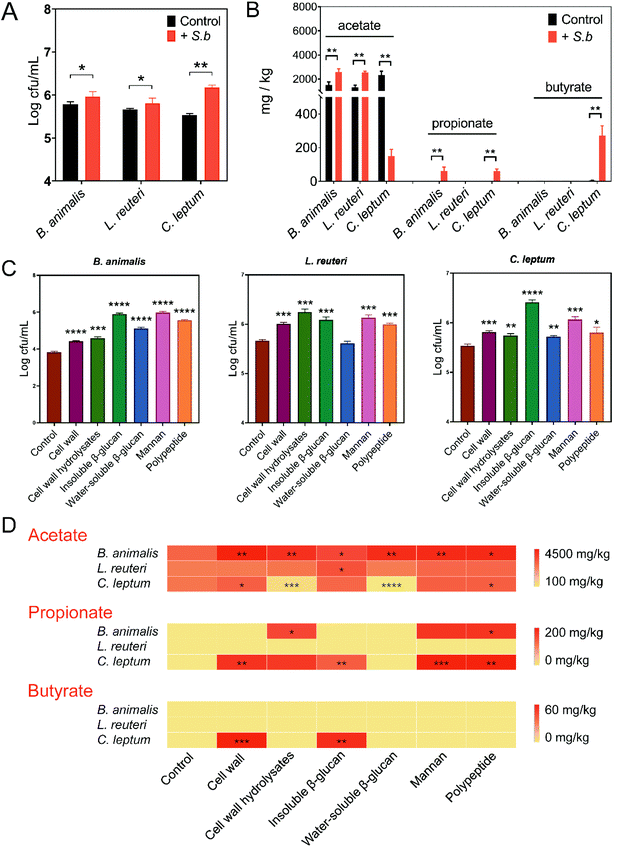 |
| Fig. 4
S. boulardii promoted the growth of certain probiotics and increased their production of SCFAs. (A) S. boulardii promoted the growth of the three probiotics. (B) S. boulardii modified the production of microbial metabolite acetate, propionate, and butyrate. (C) S. boulardii components affect the growth of certain probiotics. (D) S. boulardii components modified the production of microbial metabolite SCFAs. (A–D) n = 3 samples per group. Mean values ± SDs are presented, and p values were calculated using unpaired t-tests; *p < 0.05, **p < 0.01, ***p < 0.001 and ****p < 0.0001. | |
To identify the structural molecules of S. boulardii that are responsible for the above effects, we explored the function of different components from S. boulardii, including cell wall, β-glucans, mannan, and polypeptides. Given the different functional roles of water-soluble or insoluble polysaccharides,26 the effects of S. boulardii cell wall, cell wall hydrolysates, water-soluble β-glucans, and insoluble β-glucans were assessed separately. The effects of these S. boulardii-derived components on the growth and SCFAs production of B. animalis, L. reuteri, and C. leptum were evaluated. As shown in Fig. 4C, all these S. boulardii-derived molecules significantly promoted the growth of B. animalis and C. leptum. In the case of L. reuteri, significantly increased growth was observed except for the group supplied with water-soluble β-glucans (Fig. 4C). Next, acetate, propionate and butyrate in the supernatant were measured. The heatmap in Fig. 4D provides the statistics of the facilitatory effect of each component on microbial metabolite SCFAs. The results showed that all of these structural molecules enhanced the acetate production of B. animalis to varying degrees. Insoluble β-glucans promoted the acetate production of L. reuteri, while the cell wall and polypeptides promoted the acetate production of C. leptum. The concentration of propionate in B. animalis and C. leptum culture also significantly increased when some of these molecules were added. Moreover, the cell wall and insoluble β-glucan of S. boulardii significantly increased the butyrate production of C. leptum. In the case of L. reuteri, however, no significant changes were observed in the concentrations of propionate and butyrate.
Discussion
Various murine models have been established to mimick the colonic inflammatory cascade of IBD. However, translating these results from murine models to humans is limited due to the large differences in the intestinal microbiota composition between the two systems.27,28 In the present study, we developed a mouse model colonized with synthetic human microbiota to better explore the effect of S. boulardii on the human gut microbiota.
Our results showed that the gut microbiota profile of DSS-treated mice is drastically changed after S. boulardii supplementation. The S. boulardii treated mice possessed more Lactobacillus and Bifidobacterium but less Akkermansia. Clinical studies have shown that Lactobacillus and Bifidobacterium are effective for the maintenance of remission in IBD patients.29,30 Although Akkermansia has been reported to have potential anti-inflammatory properties and is widely accepted as the new generation of probiotics, Akkermansia-derived mucolytic enzymes can lead to the erosion of the colonic mucus layer and exacerbate colitis.31 In an azoxymethane and DSS-induced colitis mouse model, the percentage of Bacillus and Lactococcus increased, but that of Lachnoclostridium, Oscillibacter, Bacteroides, and Pseudomonas decreased after intervention with S. boulardii.32 The Firmicutes/Bacteroidetes (F/B) ratio, which has been used as a biomarker for different pathological conditions including intestinal inflammation, was almost restored after S. boulardii treatment in a DSS-induced mouse model.13 Previous studies and our work have indicated that S. boulardii might provide symptomatic relief by changing the structure of intestinal microbiota (Fig. 5).
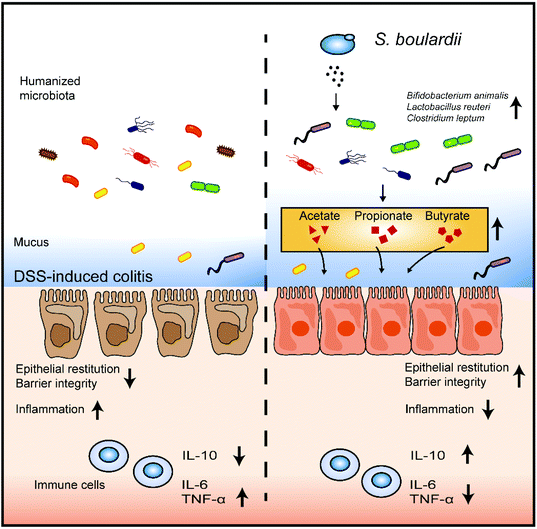 |
| Fig. 5 The potential SCFA-mediated regulation pathway of S. boulardii in DSS-induced colitis. S. boulardii modified the composition of the intestinal microbiota in humanized mice and promoted the synthesis of SCFAs. S. boulardii treatment prevented mucosal damage in the colon tissue and alleviated gut barrier disruption. S. boulardii also promoted a protective host intestinal immune response, shown as an increase in the expression level of IL-10 and a decrease in the expression levels of IL-6 and TNF-α. | |
The gut microbiota contributes to host physiology through the production of a myriad of metabolites which act as signalling molecules and substrates for metabolic reactions.33 Our results showed that S. boulardii significantly altered not only the gut microbiota composition but also the fecal metabolic phenotype. Production of SCFAs was promoted by S. boulardii (Fig. 3B and 4B). In the large intestine, SCFAs are generated by the gut microbiota through fermentation of undigested dietary fibers.34 SCFAs provide a major energy source for colon epithelial cells,35 involve in maintaining the intestinal epithelial barrier,36 and have beneficial effects on the host immune system.37,38 Several previous studies have reported that IBD is associated with impairment in SCFAs production, mainly acetate, propionate, and butyrate.39,40 The beneficial effect of epigallocatechin-3-gallate on the remission of IBD progression is mainly brought about through SCFAs producing bacteria.41 Increased microbiota-derived SCFAs levels can restore the gut barrier in DSS-induced colitis.41,42 Our analysis also suggested that SCFAs are significantly correlated with the markers of colitis severity (Fig. 3A). Among these SCFAs, butyrate is the most commonly studied. Patients with IBD have less butyrate-producing bacteria in their stools.43 Commensal microbe-derived butyrate induces the differentiation of colonic regulatory T cells and alleviates experimental colitis.44 A recent study also reported that microbiota-derived butyrate can restore the epithelial barrier through the regulation of the actin-associated protein synaptopodin.42 Enhancing the bacterial SCFAs pathway is a possible direction of IBD treatment.
Some polymers, such as polysaccharides, can be directly fermented to SCFAs by gut microbiota.45 The yeast cell wall represents 26%–32% of the dry weight of the cell and is composed primarily of polysaccharides and proteins.46 Here, we investigated the potential functional molecules derived from S. boulardii related to SCFAs production. The results showed that polysaccharides and polypeptides of S. boulardii accelerated the physiological growth and the SCFAs generation of certain probiotics. Similarly, yeast mannan selectively increased B. thetaiotaomicron and B. ovatus and increased the production of acetate, propionate, and total SCFAs.47 The exploitation of these S. boulardii-derived molecules provides new therapeutic ideas for further synbiotic formulation development for IBD patients.
Several studies have reported that S. boulardii alleviates colitis carcinogenesis in mice by regulating a variety of immune cells and modulating pro- and anti-inflammatory responses.32,48 The results of our study on the cytokine profile are consistent with those reported previously (Fig. 1E). It is worth mentioning that gut microbiota-derived SCFAs can activate G-protein-coupled receptors, stimulate macrophages and dendritic cells (DCs), regulate Treg cell differentiation, increase the expression of IL-10, and decrease the expression of IL-6.49 Those studies and ours indicate that the immunomodulatory activity of S. boulardii could be partly due to the increased levels of SCFAs.
Conclusion
In this study, our data demonstrated that the probiotic S. boulardii relieved colon inflammation in a gut microbiota-dependent manner. The underlying protective mechanism was associated with the increased production of microbiota-derived SCFAs. The findings of this research provided insights into the role of S. boulardii as a potential gut microbiota modulator to prevent and treat IBD.
Author contributions
The authors Bei Li and Haibo Zhang conducted the experiments and prepared the manuscript. Linlin Shi and Rong Li contributed to figure drawing and data analysis. Yanan Luo, Yun Deng and Shihan Li helped with the mouse experiments and manuscript proofreading. Ruizhen Li and Zhi Liu supervised the work and revised the final version of the manuscript. All the authors have read and approved the final version of the manuscript.
Conflicts of interest
There are no conflicts to declare.
Acknowledgements
This work is supported by the National Key Research and Development Project of China (2019YFA0905600). We thank Research Core Facilities for Life Science (RCFLS) in Huazhong University of Science and Technology for assistance with the GC-MS analysis.
References
- G. G. Kaplan, The Global Burden of IBD: From 2015 to 2025, Nat. Rev. Gastroenterol. Hepatol., 2015, 12, 720–727 CrossRef.
- C. L. Hvas, M. Bendix, A. Dige, J. F. Dahlerup and J. Agnholt, Current, Experimental, and Future Treatments in Inflammatory Bowel Disease: A Clinical Review, Immunopharmacol. Immunotoxicol., 2018, 40, 446–460 CrossRef CAS.
- M. Candela, S. Turroni, E. Biagi, F. Carbonero, S. Rampelli, C. Fiorentini and P. Brigidi, Inflammation and Colorectal Cancer, When Microbiota-Host Mutualism Breaks, World J. Gastroenterol., 2014, 20, 908–922 CrossRef PubMed.
- T. Lee, T. Clavel, K. Smirnov, A. Schmidt, I. Lagkouvardos, A. Walker, M. Lucio, B. Michalke, P. Schmitt-Kopplin, R. Fedorak and D. Haller, Oral Versus Intravenous Iron Replacement Therapy Distinctly Alters the Gut Microbiota and Metabolome in Patients with IBD, Gut, 2017, 66, 863–871 CrossRef CAS PubMed.
- L. O'Mahony, J. McCarthy, P. Kelly, G. Hurley, F. Luo, K. Chen, G. C. O'Sullivan, B. Kiely, J. K. Collins, F. Shanahan and E. M. Quigley, Lactobacillus and Bifidobacterium in Irritable Bowel Syndrome: Symptom Responses and Relationship to Cytokine Profiles, Gastroenterology, 2005, 128, 541–551 CrossRef.
- G. E. Barber, S. Hendler, P. Okafor, D. Limsui and B. N. Limketkai, Rising Incidence of Intestinal Infections in Inflammatory Bowel Disease: A Nationwide Analysis, Inflammatory Bowel Dis., 2018, 24, 1849–1856 CrossRef.
- I. D. Iliev, V. A. Funari, K. D. Taylor, Q. Nguyen, C. N. Reyes, S. P. Strom, J. Brown, C. A. Becker, P. R. Fleshner, M. Dubinsky, J. I. Rotter, H. L. Wang, D. P. McGovern, G. D. Brown and D. M. Underhill, Interactions between Commensal Fungi and the C-Type Lectin Receptor Dectin-1 Influence Colitis, Science, 2012, 336, 1314–1317 CrossRef CAS.
- A. K. Nash, T. A. Auchtung, M. C. Wong, D. P. Smith, J. R. Gesell, M. C. Ross, C. J. Stewart, G. A. Metcalf, D. M. Muzny, R. A. Gibbs, N. J. Ajami and J. F. Petrosino, The Gut Mycobiome of the Human Microbiome Project Healthy Cohort, Microbiome, 2017, 5, 153 CrossRef PubMed.
- H. Szajewska, M. Kołodziej and B. M. Zalewski, Systematic Review with Meta-Analysis: Saccharomyces Boulardii for Treating Acute Gastroenteritis in Children-a 2020 Update, Aliment. Pharmacol. Ther., 2020, 51, 678–688 CrossRef PubMed.
- C. M. Surawicz, G. W. Elmer, P. Speelman, L. V. McFarland, J. Chinn and G. V. Belle, Prevention of Antibiotic-Associated Diarrhea by Saccharomyces Boulardii: A Prospective Study, Gastroenterology, 1989, 96, 981–988 CrossRef CAS.
- M. Guslandi, G. Mezzi, M. Sorghi and P. A. Testoni, Saccharomyces Boulardii in Maintenance Treatment of Crohn's Disease, Dig. Dis Sci., 2000, 45, 1462–1464 CrossRef CAS PubMed.
- M. Guslandi, P. Giollo and P. A. Testoni, A Pilot Trial of Saccharomyces Boulardii in Ulcerative Colitis, Eur. J. Gastroenterol. Hepatol., 2003, 15, 697–698 CrossRef.
- A. Rodríguez-Nogales, F. Algieri, J. Garrido-Mesa, T. Vezza, M. P. Utrilla, N. Chueca, F. García, M. E. Rodríguez-Cabezas and J. Gálvez, Intestinal Anti-Inflammatory Effect of the Probiotic Saccharomyces Boulardii in DSS-Induced Colitis in Mice: Impact on Micrornas Expression and Gut Microbiota Composition, J. Nutr. Biochem., 2018, 61, 129–139 CrossRef.
- J. Garrido-Mesa, F. Algieri, A. Rodriguez-Nogales, Mª. P. Utrilla, Mª. E. Rodriguez-Cabezas, A. Zarzuelo, Mª. A. Ocete, N. Garrido-Mesa and J. Galvez, A New Therapeutic Association to Manage Relapsing Experimental Colitis: Doxycycline Plus Saccharomyces Boulardii, Pharmacol. Res., 2015, 97, 48–63 CrossRef CAS.
- C. Lee, R. Verma, S. Byun, E. J. Jeun, G. C. Kim, S. Lee, H. J. Kang, C. J. Kim, G. Sharma, A. Lahiri, S. Paul, K. S. Kim, D. S. Hwang, Y. Iwakura, I. Speciale, A. Molinaro, C. De Castro, D. Rudra and S. H. Im, Structural Specificities of Cell Surface Beta-Glucan Polysaccharides Determine Commensal Yeast Mediated Immuno-Modulatory Activities, Nat. Commun., 2021, 12, 3611 CrossRef CAS PubMed.
- T. Kamiya, C. Tang, M. Kadoki, K. Oshima, M. Hattori, S. Saijo, Y. Adachi, N. Ohno and Y. Iwakura, B-Glucans in Food Modify Colonic Microflora by Inducing Antimicrobial Protein, Calprotectin, in a Dectin-1-Induced-Il-17f-Dependent Manner, Mucosal Immunol., 2018, 11, 763–773 CrossRef CAS PubMed.
- L. Shi, Q. Lin, T. Yang, Y. Nie, X. Li, B. Liu, J. Shen, Y. Liang, Y. Tang and F. Luo, Oral Administration of Lentinus Edodes B-Glucans Ameliorates Dss-Induced Ulcerative Colitis in Mice Via Mapk-Elk-1 and Mapk-Pparγ Pathways, Food Funct., 2016, 7, 4614–4627 RSC.
- B. Liu, Q. Lin, T. Yang, L. Zeng, L. Shi, Y. Chen and F. Luo, Oat B-Glucan Ameliorates Dextran Sulfate Sodium (DSS)-Induced Ulcerative Colitis in Mice, Food Funct., 2015, 6, 3454–3463 RSC.
- M. B. Ye, J. P. Bak, C. S. An, H. L. Jin, J. M. Kim, H. J. Kweon, D. K. Choi, P. J. Park, Y. J. Kim and B. O. Lim, Dietary B-Glucan Regulates the Levels of Inflammatory Factors, Inflammatory Cytokines, and Immunoglobulins in Interleukin-10 Knockout Mice, J. Med. Food, 2011, 14, 468–474 CrossRef CAS.
- Z. Wang, E. Klipfell, B. J. Bennett, R. Koeth, B. S. Levison, B. Dugar, A. E. Feldstein, E. B. Britt, X. Fu, Y. M. Chung, Y. Wu, P. Schauer, J. D. Smith, H. Allayee, W. H. Tang, J. A. DiDonato, A. J. Lusis and S. L. Hazen, Gut Flora Metabolism of Phosphatidylcholine Promotes Cardiovascular Disease, Nature, 2011, 472, 57–63 CrossRef CAS PubMed.
- M. Schenk, A. Bouchon, F. Seibold and C. Mueller, Trem-1–Expressing Intestinal Macrophages Crucially Amplify Chronic Inflammation in Experimental Colitis and Inflammatory Bowel Diseases, J. Clin. Invest., 2007, 117, 3097–3106 CrossRef CAS.
- L. Zhao, F. Zhang, X. Ding, G. Wu, Y. Y. Lam, X. Wang, H. Fu, X. Xue, C. Lu, J. Ma, L. Yu, C. Xu, Z. Ren, Y. Xu, S. Xu, H. Shen, X. Zhu, Y. Shi, Q. Shen, W. Dong, R. Liu, Y. Ling, Y. Zeng, X. Wang, Q. Zhang, J. Wang, L. Wang, Y. Wu, B. Zeng, H. Wei, M. Zhang, Y. Peng and C. Zhang, Gut Bacteria Selectively Promoted by Dietary Fibers Alleviate Type 2 Diabetes, Science, 2018, 359, 1151–1156 CrossRef CAS.
- S. Zhang, H. Wang and M. J. Zhu, A Sensitive Gc/Ms Detection Method for Analyzing Microbial Metabolites
Short Chain Fatty Acids in Fecal and Serum Samples, Talanta, 2019, 196, 249–254 CrossRef CAS PubMed.
- Y. Zou, W. Xue, G. Luo, Z. Deng, P. Qin, R. Guo, H. Sun, Y. Xia, S. Liang, Y. Dai, D. Wan, R. Jiang, L. Su, Q. Feng, Z. Jie, T. Guo, Z. Xia, C. Liu, J. Yu, Y. Lin, S. Tang, G. Huo, X. Xu, Y. Hou, X. Liu, J. Wang, H. Yang, K. Kristiansen, J. Li, H. Jia and L. Xiao, 1,520 Reference Genomes from Cultivated Human Gut Bacteria Enable Functional Microbiome Analyses, Nat. Biotechnol., 2019, 37, 179–185 CrossRef CAS.
- M. Schirmer, A. Garner, H. Vlamakis and R. J. Xavier, Microbial Genes and Pathways in Inflammatory Bowel Disease, Nat. Rev. Microbiol., 2019, 17, 497–511 CrossRef CAS PubMed.
- C. H. Su, Y. T. Tseng, K. Y. Lo, M. N. Lai and L. T. Ng, Differences in Anti-Inflammatory Properties of Water Soluble and Insoluble Bioactive Polysaccharides in Lipopolysaccharide-Stimulated Raw264.7 Macrophages, Glycoconjugate J., 2020, 37, 565–576 CrossRef CAS.
- T. L. A. Nguyen, S. Vieira-Silva, A. Liston and J. Raes, How Informative Is the Mouse for Human Gut Microbiota Research?, Dis. Models Mech., 2015, 8, 1–16 CrossRef.
- F. Hugenholtz and W. M. de Vos, Mouse Models for Human Intestinal Microbiota Research: A Critical Evaluation, Cell. Mol. Life Sci., 2018, 75, 149–160 CrossRef CAS.
- T. Kühbacher, S. J. Ott, U. Helwig, T. Mimura, F. Rizzello, B. Kleessen, P. Gionchetti, M. Blaut, M. Campieri, U. R. Fölsch, M. A. Kamm and S. Schreiber, Bacterial and Fungal Microbiota in Relation to Probiotic Therapy (Vsl#3) in Pouchitis, Gut, 2006, 55, 833 CrossRef.
- A. Tursi, G. Brandimarte, A. Papa, A. Giglio, W. Elisei, G. M. Giorgetti, G. Forti, S. Morini, C. Hassan, M. A. Pistoia, M. E. Modeo, S. Rodino, T. D'Amico, L. Sebkova, N. Sacca, E. Di Giulio, F. Luzza, M. Imeneo, T. Larussa, S. Di Rosa, V. Annese, S. Danese and A. Gasbarrini, Treatment of Relapsing Mild-to-Moderate Ulcerative Colitis with the Probiotic Vsl#3 as Adjunctive to a Standard Pharmaceutical Treatment: A Double-Blind, Randomized, Placebo-Controlled Study, Am. J. Gastroenterol., 2010, 105, 2218–2227 CrossRef PubMed.
- S. Khan, S. Waliullah, V. Godfrey, M. A. W. Khan, R. A. Ramachandran, B. L. Cantarel, C. Behrendt, L. Peng, L. V. Hooper and H. Zaki, Dietary Simple Sugars Alter Microbial Ecology in the Gut and Promote Colitis in Mice, Sci. Transl. Med., 2020, 12(567), eaay6218 CrossRef CAS.
- C. Wang, W. Li, H. Wang, Y. Ma, X. Zhao, X. Zhang, H. Yang, J. Qian and J. Li, Saccharomyces Boulardii Alleviates Ulcerative Colitis Carcinogenesis in Mice by Reducing Tnf-Alpha and Il-6 Levels and Functions and by Rebalancing Intestinal Microbiota, BMC Microbiol., 2019, 19, 246 CrossRef PubMed.
- K. A. Krautkramer, J. Fan and F. Backhed, Gut Microbial Metabolites as Multi-Kingdom Intermediates, Nat. Rev. Microbiol., 2021, 19, 77–94 CrossRef CAS.
- D. L. Topping and P. M. Clifton, Short-Chain Fatty Acids and Human Colonic Function: Roles of Resistant Starch and Nonstarch Polysaccharides, Physiol. Rev., 2001, 81(3), 1031–1064 CrossRef CAS.
- D.
R. Donohoe, N. Garge, X. Zhang, W. Sun, T. M. O'Connell, M. K. Bunger and S. J. Bultman, The Microbiome and Butyrate Regulate Energy Metabolism and Autophagy in the Mammalian Colon, Cell Metab., 2011, 13, 517–526 CrossRef CAS PubMed.
- C. J. Kelly, L. Zheng, E. L. Campbell, B. Saeedi, C. C. Scholz, A. J. Bayless, K. E. Wilson, L. E. Glover, D. J. Kominsky, A. Magnuson, T. L. Weir, S. F. Ehrentraut, C. Pickel, K. A. Kuhn, J. M. Lanis, V. Nguyen, C. T. Taylor and S. P. Colgan, Crosstalk between Microbiota-Derived Short-Chain Fatty Acids and Intestinal Epithelial Hif Augments Tissue Barrier Function, Cell Host Microbe, 2015, 17, 662–671 CrossRef CAS.
- N. Kamada, S. U. Seo, G. Y. Chen and G. Nunez, Role of the Gut Microbiota in Immunity and Inflammatory Disease, Nat. Rev. Immunol., 2013, 13, 321–335 CrossRef CAS PubMed.
- W. Guo, Q. Xiang, B. Mao, X. Tang, S. Cui, X. Li, J. Zhao, H. Zhang and W. Chen, Protective Effects of Microbiome-Derived Inosine on Lipopolysaccharide-Induced Acute Liver Damage and Inflammation in Mice Via Mediating the Tlr4/Nf-Kb Pathway, J. Agric. Food Chem., 2021, 69, 7619–7628 CrossRef CAS PubMed.
- M. Sun, W. Wu, Z. Liu and Y. Cong, Microbiota Metabolite Short Chain Fatty Acids, Gpcr, and Inflammatory Bowel Diseases, J. Gastroenterol., 2017, 52, 1–8 CrossRef CAS.
- C. De Filippo, D. Cavalieri, M. Di Paola, M. Ramazzotti, J. B. Poullet, S. Massart, S. Collini, G. Pieraccini and P. Lionetti, Impact of Diet in Shaping Gut Microbiota Revealed by a Comparative Study in Children from Europe and Rural Africa, Proc. Natl. Acad. Sci. U. S. A., 2010, 107, 14691–14696 CrossRef.
- Z. Wu, S. Huang, T. Li, N. Li, D. Han, B. Zhang, Z. Z. Xu, S. Zhang, J. Pang, S. Wang, G. Zhang, J. Zhao and J. Wang, Gut Microbiota from Green Tea Polyphenol-Dosed Mice Improves Intestinal Epithelial Homeostasis and Ameliorates Experimental Colitis, Microbiome, 2021, 9, 184 CrossRef.
- R. X. Wang, J. S. Lee, E. L. Campbell and S. P. Colgan, Microbiota-Derived Butyrate Dynamically Regulates Intestinal Homeostasis through Regulation of Actin-Associated Protein Synaptopodin, Proc. Natl. Acad. Sci. U. S. A., 2020, 117, 11648–11657 CrossRef CAS.
- V. Eeckhaut, K. Machiels, C. Perrier, C. Romero, S. Maes, B. Flahou, M. Steppe, F. Haesebrouck, B. Sas, R. Ducatelle, S. Vermeire and F. Van Immerseel, Butyricicoccus Pullicaecorum in Inflammatory Bowel Disease, Gut, 2013, 62, 1745–1752 CrossRef CAS.
- Y. Furusawa, Y. Obata, S. Fukuda, T. A. Endo, G. Nakato, D. Takahashi, Y. Nakanishi, C. Uetake, K. Kato, T. Kato, M. Takahashi, N. N. Fukuda, S. Murakami, E. Miyauchi, S. Hino, K. Atarashi, S. Onawa, Y. Fujimura, T. Lockett, J. M. Clarke, D. L. Topping, M. Tomita, S. Hori, O. Ohara, T. Morita, H. Koseki, J. Kikuchi, K. Honda, K. Hase and H. Ohno, Commensal Microbe-Derived Butyrate Induces the Differentiation of Colonic Regulatory T Cells, Nature, 2013, 504, 446–450 CrossRef CAS PubMed.
- V. Kumar, A. K. Sinha, H. P. Makkar, G. de Boeck and K. Becker, Dietary Roles of Non-Starch Polysaccharides in Human Nutrition: A Review, Crit. Rev. Food Sci. Nutr., 2012, 52, 899–935 CrossRef CAS PubMed.
- T. H. Nguyen, G. H. Fleet and P. L. Rogers, Composition of the Cell Walls of Several Yeast Species, Appl. Microbiol. Biotechnol., 1998, 50, 206–212 CrossRef CAS.
- S. Oba, T. Sunagawa, R. Tanihiro, K. Awashima, H. Sugiyama, T. Odani, Y. Nakamura, A. Kondo, D. Sasaki and K. Sasaki, Prebiotic Effects of Yeast Mannan, Which Selectively Promotes Bacteroides Thetaiotaomicron and Bacteroides Ovatus in a Human Colonic Microbiota Model, Sci. Rep., 2020, 10, 17351 CrossRef CAS.
- K. Sivananthan and A. M. Petersen, Review Ofsaccharomyces Boulardiias a Treatment Option in Ibd, Immunopharmacol. Immunotoxicol., 2018, 40, 465–475 CrossRef CAS PubMed.
- N. Singh, A. Gurav, S. Sivaprakasam, E. Brady, R. Padia, H. Shi, M. Thangaraju, P. D. Prasad, S. Manicassamy, D. H. Munn, J. R. Lee, S. Offermanns and V. Ganapathy, Activation of Gpr109a, Receptor for Niacin and the Commensal Metabolite Butyrate, Suppresses Colonic Inflammation and Carcinogenesis, Immunity, 2014, 40, 128–139 CrossRef CAS.
Footnote |
† Electronic supplementary information (ESI) available. See DOI: 10.1039/d1fo02752b |
|
This journal is © The Royal Society of Chemistry 2022 |
Click here to see how this site uses Cookies. View our privacy policy here.