DOI:
10.1039/D1FO01604K
(Paper)
Food Funct., 2022,
13, 386-397
Fabrication and emulsifying properties of non-covalent complexes between soy protein isolate fibrils and soy soluble polysaccharides†
Received
23rd May 2021
, Accepted 1st October 2021
First published on 3rd October 2021
1. Introduction
As a biocompatible and easily prepared transporter for bioactive substances, oil-in-water (O/W) emulsions are finding increasing applications in the food industry (e.g., sauces, beverages), cosmetic products and drug delivery systems.1 Generally, this class of emulsions consist of a dispersed phase (e.g., oil), a continuous phase (e.g., water) and a surfactant to stabilize the emulsion.2 However, the emulsion system can be unstable and processes, such as creaming, flocculation and aggregation, caused by gravity, temperature and oxidation, result in phase-separation.3 Therefore, the choice of the surfactant is the main determinant of emulsion stability; surfactant is adsorbed onto the oil-droplet surface and reduces the interfacial tension by reducing the free energy, thereby forming a stable surface structure.4 Synthetic surfactants like Spans (quaternary ammonium detergents) and Tweens (polyoxyethylene detergents) have excellent emulsifying properties, but the toxicity and food safety issues of these surfactants bring into question their suitability for food use.5 In contrast, plant or animal extracts with emulsifying properties (e.g., soy protein isolate, or whey protein) can be used without restriction in foods and have strong consumer acceptance.6
To improve the stability of emulsions made with natural emulsifiers, composite-type surfactants made from protein–carbohydrate complexes have been studied extensively.7 In these complexes, carbohydrate combines with protein using the Maillard reaction, hydrogen bonding, electrostatic or hydrophobic interactions, which changes the protein structure and modifies its hydrophobicity, flexibility and surface charge. Liu et al. prepared anisotropic whey protein nanofibrils by glycation with glucose, lactose, or maltodextrin at pH 2.0 and 90 °C. They found that the glycated WPI fibrils with a higher MW of saccharides became more surface active and would form thicker interfacial layers which reduced the effects of pH and ionic strength on emulsion stability.8 Similarly, the interaction between β-lactoglobulin fibrils and chitosan enhanced the oxidative stability of the fish oil in water emulsion, which is attributed to the complex that formed an effective interfacial double layer on the oil–water interface.9 Polysaccharides have great potential to modify the protein structure and enhance its emulsifying properties.10 Protein/polysaccharide complexes possess excellent amphiphilic properties, because the relatively hydrophobic protein and hydrophilic polysaccharide facilitate strong interactions with the oil and water phases, respectively, and the bulky hydrophilic polysaccharide region provides steric stabilization to prevent physical separation of the two phases.11
Soy protein isolate (SPI), a common plant-based natural material used for emulsifying, is composed mainly of 11S and 7S globulins (about 70%), which dominate its functional properties.12 However, because of the high molecular weight, compact globular structure and limited water-solubility of the globulins, the emulsifying capacity of SPI is relatively weak compared with animal-based emulsifiers, such as sodium caseinate, or whey protein.11,13 Hence, it is necessary to make some modifications to boost the emulsifying properties of SPI. Recently, fibrils that were formed from whey protein, soy protein isolate and rice bran protein have been widely reported. Under low pH (2.0) and heat (85 °C) conditions, SPI unfolds its globular structure and goes through a hydrolytic process, the obtained active peptide monomers form oligomers and further assemble into fibrils.14,15 Ultimately, more hydrophobic groups that are wrapped in the globular protein can be exposed to the aqueous phase, resulting in faster adsorption kinetics and a higher emulsifying activity index (EAI) compared with the native SPI.16,17 Nevertheless, in the actual experiment, we found that the emulsions stabilized by SPI fibrils (SPIF) showed obvious phase separation during short-term storage. These results are consistent with a report by Peng et al., revealing that fibrils can induce depletion-flocculation of droplets at low concentrations.18 Therefore, it is necessary to incorporate polysaccharides into the emulsion system to optimize the emulsifying capacity of the protein.
As a byproduct of SPI, soy soluble polysaccharides (SSPS) are pectin-like acidic biopolymers which mainly composed of a rhamnogalacturonan backbone branched by β-1,4-galactan and α-1,3 or α-1,5-arabinan chains.19 Besides, it has low viscosity, high solubility and excellent stability in aqueous solution. In this study, acid/heat treatment was applied to form soy protein isolate fibers (SPIF). Then SSPS was combined with SPIF to form protein–polysaccharide complexes (SPIF/SSPS complexes). The aim was to confirm the complexation between SPIF and SSPS, using turbidimetric measurements, fluorescence spectra and Fourier-transform infrared spectroscopy (FTIR). Then, the determination of dynamic interfacial pressure was used to analyze the interfacial adsorption behavior of SPIF/SSPS complexes on the oil–water interface. Furthermore, the emulsifying activity index (EAI) and emulsion stability index (ESI) of the complex were determined to evaluate its emulsification capacity. The stability of emulsions stabilized by complexes with different protein/polysaccharide ratios was studied for three weeks by observing the particle size distribution, ζ-(zeta)-potential and morphology of emulsified oil-droplets, as well as by photography and the Turbiscan stability index (TSI).
2. Materials and methods
2.1 Materials
Commercial grade soy soluble polysaccharide (SSPS, >75%) was purchased from Shandong Juyuan Biotechnology Co., Ltd (Liaocheng, China). Soy protein isolate (SPI, >90%) was purchased from Shandong Biological Products Co., Ltd (Linyi, China). Soy oil was purchased from Jiu San Grain and Oil Industry Group Co., Ltd (Harbin, China). 1-Anilinonaphthalene-8-sulfonic acid (ANS) was purchased from Aladdin Industrial Corporation (Shanghai, China). All other chemicals were of analytical grade.
2.2 Preparation of SPIF
The SPIF was prepared according to the previous method reported by Wang et al. with minor modifications.14 The SPI sample (6 g) was dissolved in deionized water (4%) under stirring at room temperature for 24 h to ensure complete protein hydration. Then, the pH of the solution was adjusted to 2.0 using 6.0 M HCl and stirred 24 h at 4 °C. The obtained solution was centrifuged at 10
000g for 10 min at 4 °C, and the supernatants were heated in tubes at 85 °C for 48 h. After that, these tubes were immediately placed in an ice bath and dried by vacuum freeze-drying for further experiments.
2.3 Preparation of SPIF/SSPS complex
The SPIF solution (0.2%, w/w) was prepared by dissolving SPIF powders in distilled water and stirred for 12 h at room temperature. Different concentrations of SSPS solution (0.04%, 0.08%, 0.12%, 0.16%, 0.2%, w/w) were prepared under the same conditions. Afterwards, equal volumes of these two solutions were mixed together and stirred overnight at room temperature to obtain the SPIF/SSPS complex solution. The final concentration proportions of SPIF and SSPS were 5
:
1, 5
:
2, 5
:
3, 5
:
4 and 5
:
5 which were used to name the different samples.
2.4 Characterization of SPIF and the SPIF/SSPS complex
2.4.1. Morphological observation.
An atomic force microscope (AFM) (Park Systems, XE-70, Suwon, Korea) and a light microscope (BDS400, Optec Instrument Co., Ltd, Chongqing, China) were used to observe the microstructure of SPIF and the SPIF/SSPS complex at a ratio of 5
:
5. Before observation, the samples were diluted to 1 μg mL−1 and 1 mg ml−1 using ultrapure water. Then 5 μL droplet of these two samples was placed on a freshly stripped mica disc (10 × 10 mm) and dried at room temperature for 30 min. The sample with a concentration of 1 μg mL−1 was used for AFM observation and the other was used for light microscopy observation.
2.4.2. Turbidity, particle size and zeta potential.
The turbidity values were represented by monitoring the absorbance at 600 nm using an ultraviolet spectrophotometer (UV2550, Shimadzu, Japan). The particle size and zeta potential of the mixed solutions were evaluated by a dynamic light scattering method using a Brookhaven Zeta PALS system (90Plus zeta, Brookhaven Instruments Corporation, Holtsville, NY, USA) at 25 °C. All the samples were diluted 50-fold using ultrapure water before measurement. The final values were represented by the average of at least three independent trials.
2.4.3. Fluorescence spectroscopy.
2 mL of the sample solutions were injected into a quartz cuvette and analyzed using a fluorescence spectrophotometer (F7000, Hitachi, Japan) at 25 °C with a scan rate at 2400 nm min−1. The optical path was set to 1 cm, both the excitation and emission slit widths were fixed at 2.5 nm. The excitation wavelength was 290 nm, and the emission spectra ranged from 300 to 400 nm.
2.4.4. Fourier transform infrared spectroscopy (FT-IR).
A portion of the mixed solutions from 2.3.1 was dried out through a vacuum freeze-drying machine. Then the obtained powders were uniformly mixed with KBr at a mass ratio of 1
:
100, and slices were formed to monitor FTIR using an FTIR spectrophotometer (IRTracer-100, Shimadzu, Japan) in the range of 400–4000 cm−1 at 25 °C. Pure KBr was used as the background correction.
2.4.5. Surface hydrophobicity (H0).
The surface hydrophobicity (H0) of SPIF, SSPS and the SPIF/SSPS complex was determined using a fluorescence spectrophotometer (F7000, Hitachi, Japan) with 1-anilino-8-naphthalene sulfonate (ANS) as a fluorescent probe.20 Briefly, ANS was dissolved at a concentration of 0.008 mol L−1 with 5 mM PBS solution (pH 7.0) and then the aliquots (20 μL) of ANS solution was mixed with the sample solution thoroughly. The fluorescence intensity was detected at an excitation wavelength of 390 nm and emission wavelength of 400–650 nm and the emission and excitation slits were set as 2.5 nm. The scanning speed was 1200 nm min−1. The area of the fluorescence spectrum can be used to represent the surface hydrophobicity of the samples.
2.4.6. Dynamic interfacial pressure and adsorption kinetics.
The time-dependent interfacial pressure (π) of SPI, SSPS and SPIF/SSPS at the oil–water interface was recorded using an optical contact angle meter (OCA25, Dataphysics Instruments GmbH, Germany). Oil was placed in a cuvette, and then, a 10 μL drop of sample solution was delivered into the oil phase and maintained for 3 h at 25 °C to achieve sample adsorption. The image of the drop was collected and digitized, and the surface tension (γ) was calculated according to the fundamental Laplace equation and the oil–water interface pressure (π, mN m−1) was calculated using eqn (1):where γ0 (mN m−1) is the interfacial tension of distilled water. Generally, the dynamic adsorption process includes diffusion, penetration and reorganization.21 In the early adsorption stage, a modified form of the Ward and Tordai equation can be used to express the change in interfacial pressure (π) with adsorption time (t) defined using eqn (2):22where C0 is the concentration in the continuous phase, K is the Boltzmann constant, T is the absolute temperature, D is the diffusion coefficient, and t is adsorption time (s). The plot of π against t1/2 will be linear if the adsorption process is diffusion-controlled, and the plot will be the diffusion rate (Kdiff).23 In addition, the penetration and reorganization of the complex onto the oil–water interface can be analyzed using the first-order equation:24 |  | (3) |
where πf, π0, and πt are the interfacial pressure at the final adsorption time, at the initial time, and at any time of each stage, respectively, and ki is the first-order rate constant.
2.5 Preparation and characterization of emulsions
The SPIF/SSPS complex was obtained according to the method described above via vacuum freeze-drying and dissolved in deionized water under stirring at room temperature for 12 h. Emulsion preparation was according to our previous studies with some modifications.25 Briefly, the oil in water emulsion was obtained by homogenizing 20% w/w soybean oil and 80% w/w SPIF/SSPS complex solution using a high-speed homogenizer (FJ-200, Specimen model factory, Shanghai, China) at 10
000 rpm for 3 min and further passed three times through a high-pressure homogenizer (FB-110S, LiTu Machinery Equipment Engineering Co., Ltd, Shanghai, China) at 30 MPa. Sodium azide (0.01% w/w) was added to all samples to prevent microbial growth. The concentration of the SPIF/SSPS complex is 0.5% w/w in the final emulsion and was named with the SPIF and SSPS ratio. As contrasts, emulsions containing 0.5% or 1% w/w SPIF without SSPS, and 0.5% or 1% w/w SSPS without SPIF were prepared and defined as SPIF0.5, SPIF1, SSPS0.5 and SSPS1, respectively.
2.5.1. Emulsifying activity.
The emulsifying activity (EAI) and emulsifying stability index (ESI) were calculated to evaluate the emulsifying properties according to the reports described by Liu et al.26 Equal volumes of fresh emulsions were transferred into glass bottles and placed at room temperature. 20 μL emulsions were pipetted from the bottom of bottles and diluted 100-fold with sodium dodecyl sulphate (SDS) solution (0.1%, W/V) at 0 and 180 min. These absorbances of different samples were recorded at 500 nm using an ultraviolet spectrophotometer (UV2550, Shimadzu, Japan). ESI and EAI were calculated on the basis of these absorbances measured at 0 (A0) and 180 min (A180) using eqn (4) and (5). |  | (4) |
|  | (5) |
where T = 2.303, C is the sample concentrate, N is the dilution factor and Φ is the oil phase ratio.
2.5.2. Particle size and zeta potential.
The particle size distribution of the fresh emulsion was measured using a BT-9300ST laser diffraction analyzer (Baite Instrument Co., Ltd, Dandong, China). The refractive index of deionized water was 213 and those of droplets were 1.333 and 1.520, respectively. Additionally, a Nano-ZS90 system (Malvern Instruments Ltd, Britain) was used only for zeta-potential analysis. Before the test, all samples were diluted 100-fold using ultrapure water and 0.5 mL of sample was injected into 1 cm polystyrene cells equipped with a Pd electrode. All the measurements were repeated three times at 25 °C.
2.5.3. Optical microscopy and digital photography.
The micromorphology of fresh emulsions was observed using a light microscope (Nikon 80i, Japan) fitted with the NIS-Elements imaging analysis software (Nikon, Melville, NY). Before the observation, every sample was diluted 50-fold using deionized water. 20 μL of the sample was put onto a glass slide and covered with a coverslip, and then observed at 500× magnification. Equal volumes of different emulsions were put into glass bottles and take pictures at 1 day and 21 days with a camera to compare the emulsion stability.
2.5.4. Turbiscan stability index of emulsions.
The physical stability of emulsions was evaluated using a Turbiscan Lab Expert analyzer (Lab expert, Formulaction Inc., Toulouse, France). Fresh emulsions (20 mL) were immediately placed in the test glass tube and then the entire height of the samples was scanned at 25 °C after different storage times. The transmittance detector and backscattering detector received the light; the Turbiscan Stability Index (TSI) was obtained after the analysis of light intensity using the Turbiscan software.
2.6 Statistical analysis
The experiments were performed at least three times and the results were analyzed using SSPS Statistics 19 (Chicago, IL, USA) and expressed as the mean ± standard deviation of triplicate determinations. Treatments were considered significantly different at p < 0.05.
3. Results and discussion
3.1 Effect of SSPS on the formation of complexes
3.1.1 Turbidity, particle size and zeta potential analysis.
Fig. 1 shows the turbidity (A), particle size/zeta potential (B) and microstructure (C–F) of SSPS, SPIF, and SPIF/SSPS complexes with different SPIF/SSPS ratios. Generally, the generation of turbidity is a complex phenomenon, which is usually associated with increased complexation; turbidity can be influenced by factors such as the color of the sample, particle diameter, refractive index and interactions between particles.10,27 Both SPIF and SSPS were clear solutions (Fig. 1A), whereas the complexes had a milky appearance, which increased with the proportion of SSPS in the complex, in agreement with the absorbance values at 420 nm. The highest turbidity (0.49) corresponded to the highest SSPS content (5
:
5 ratio), whereas the lowest turbidity (0.04) was for the SSPS control, indicating that the increase in turbidity results from an increase in the complexation between SPIF and SSPS.
 |
| Fig. 1 (A) Turbidity of the SPIF, SSPS and SPIF/SSPS complexes (0.1%, w/w) with different SPIF and SSPS ratios. Inset: The corresponding optical photographs of samples. (B) Particle sizes of SPIF, SSPS and SPIF/SSPS complexes (0.1%, w/w) with different SPIF and SSPS ratios. Inset: The corresponding zeta potential of the samples. Values are given as the means ± SD from triplicate measurements; different characteristics (a–d) on the top of the columns represent the significant difference at p < 0.05. (C) AFM images of SPIF (1 μg mL−1, scale bar: 500 nm). (D) AFM images of the SPIF/SSPS complex at 5 : 5 ratio (1 μg mL−1 scale bar: 500 nm). (E) Light microscopy images of SPIF (1 mg mL−1). (F) Light microscopy images of the SPIF/SSPS complex at 5 : 5 ratio (1 mg mL−1). 400×; scale bar: 10 μm. | |
Zeta-potential measurements (Fig. 1B) revealed that SPIF (21.9 mV) and SSPS (−20.1 mV) had potentials of similar size, but were oppositely charged. As the proportion of SSPS increased, the zeta-potential of the complex became more negative and at the SPIF/SSPS ratios of 5
:
2 (−0.49 mV) and 5
:
3 (−0.93 mV) the complexes were close to overall electrical neutrality. The decrease of zeta-potential generally considers as a result of electrostatic bonding between oppositely charged molecules or ions.9 Similarly, the negatively charged SSPS forms complexes with positively charged proteins through both electrostatic and hydrophobic interactions as demonstrated in the previous work.28 Hence, the changes in zeta-potential and turbidity appear to result from the formation of SPIF/SSPS complexes, and the interactions between them appear to be mainly electrostatic. Observations of the microstructure of SPIF and the SPIF/SSPS complex (5
:
5 ratio) by AFM (Fig. 1C and D) revealed that the average length of SPIF is about 500 nm with heights of a few nanometers. After equal SSPS was added to SPIF, only small particles were observed. However, laser light-scattering particle size measurements (Fig. 1B) indicate that SPIF had by far the largest particle size (1658 nm), but the particle size decreased significantly in mixtures of SPIF and SSPS (p < 0.05). The light-scattering particle size of SPIF was much larger than that observed by AFM (Fig. 1C). This difference may result from a higher concentration (0.5% w/w) leading to aggregation via hydrophobic interactions among the SPIF. Aggregation of SPIF in the control sample is supported by a light microscopy image (Fig. 1E), which shows an extensive dendritic structure, however, this dendritic structure appears to be disrupted by the presence of SSPS (Fig. 1F). A similar result was reported in which steric repulsion derived from SSPS is attributed to the deaggregation effect.29 The conjugation of SPIF with SSPS would be expected to reduce its potential for intermolecular hydrophobic interactions and this appears to weaken the binding interactions in the aggregates sufficiently to disperse them. Overall, it appears that the SPIF/SSPS complex was obtained successfully and had both higher dispersity and a smaller particle size in solution.
3.1.2 Fluorescence spectroscopy analysis.
Fluorescence spectroscopy has been widely used for analyzing the structure of protein hydrolysate complexes with other molecules. The fluorescence intensity and maximum emission wavelength depend on the content of aromatic amino acids (e.g., tyrosine and tryptophan) and their microenvironment, which is modified by changes in the protein conformation.7 SSPS produced essentially no fluorescence emission, because it contains very little protein (Fig. 2). The fluorescence intensity of SPIF decreased, as the proportion of SSPS in the mixture increased; this fluorescence quenching phenomenon might be derived from two aspects: (I) the presence of SSPS induced conformational changes in the protein. (II) The addition of SSPS coincided with increased turbidity, and reduced fluorescence emission could also be caused in part by loss from scattering. In addition, the maximum absorption wavelength (λmax) changed slightly as the proportion of SSPS increased (341, 343, 344, 342, 342 and 344 nm for SPIF, 5
:
1, 5
:
2, 5
:
3, 5
:
4, and 5
:
5, respectively), i.e., a slight red shift. These changes in fluorescence indicated that the presence of SSPS changed the conformation of SPIF and exposed the fluorescent chromophores to a more polar microenvironment.19,30 A similar result for the interaction of soy protein isolate fibrils with betalain was reported by Zhao et al.31
 |
| Fig. 2 Intrinsic fluorescence of SPIF, SSPS and the SPIF/SSPS complex with different SPIF and SSPS ratios. | |
3.1.3 Fourier transform infrared spectroscopy analysis.
The FTIR spectra of SPIF, SSPS and the SPIF/SSPS complexes were recorded (Fig. 3). The intensity and position of FTIR absorption peaks reflect changes in the protein secondary structure involving hydrogen bonding, polypeptide backbone conformation, or dipole–dipole interactions during the conjugation process.9 In the spectrum of SSPS, the band in 3600–3050 cm−1 was attributed to the O–H stretching vibration of the hydroxyl group and the characteristic peak at 1060 cm−1 was attributed to the rhamnogalacturonan moiety.32,33 Besides, for SPIF/SSPS complexes, the intensity of the characteristic peak at 1060 cm−1 was gradually enhanced with the increase of SSPS, suggesting the formation of a complex. The spectrum of SPIF showed characteristic peaks at 3293 cm−1 (O–H stretching, N–H stretching), 1651 cm−1 (amide I, C
O stretching), 1536 cm−1 (amide II, C–N stretching coupled with NH bending modes) and 1234 cm−1 (amide III, C–N stretching).34 Furthermore, after the addition of SSPS, the intensities for amide I (1700–1600 cm−1), amide II (1600–1500 cm−1) and amide III (1300–1200 cm−1) changed, and the peak position shifted from 1234 to 1230 cm−1 for amide III, which indicated that the secondary structure of SPIF was altered. Moreover, the PeakFit 4.04 software was used to quantify the secondary structure of SPIF and SPIF/SSPS complexes by analyzing the amide I band (1700–1600 cm−1), and the details are shown in Table S1.† Obviously, The β-sheet (66.72%) and β-turn (31.59%) accounted for most of the secondary structures of SPIF, and no α-helix was found. However, with an increase of SSPS, the content of the β-sheet decreased and the content of the α-helix increased. This phenomenon might be due to the interactions between SPIF and SSPS, and lower β-sheet content could reduce the hydrophobicity of the SPIF/SSPS complex, which agrees with the result shown in the 3.1.4 section. Additionally, the position of the O–H and N–H stretching in the SPIF/SSPS complexes changed significantly, shifting to higher wavenumbers (3294–3392 cm−1) as the proportion of SSPS in the complexes increased. These spectral changes indicate that hydrogen bonding was involved in the formation of the SPIF/SSPS complexes.19
 |
| Fig. 3 FT-IR spectra of SPIF, SSPS and the SPIF/SSPS complex with different SPIF and SSPS ratios. | |
3.1.4 Surface hydrophobicity (H0) analysis.
Surface hydrophobicity (H0) is an important indicator that is closely related to the structural changes and surface properties of proteins.12 The H0 values of the SPIF/SSPS complexes were markedly lower than that of SPIF and decreased with increasing proportions of SSPS (Fig. 4). SSPS had no fluorescence emission spectrum in the range 400–650 nm, which indicates that its H0 is close to zero. Structural rearrangement during the formation of SPIF resulted in hydrophobic residues being exposed to the aqueous medium and consequently, a higher H0.34 However, the surface hydrophobicity of SPIF/SSPS complexes decreased from 1249.56 (SPIF) to 761.56 (5
:
5 SSPS ratio). This apparently resulted from binding between the hydrophobic regions of SPIF and ANA being impeded by the presence of SSPS, some exposed hydrophobic sites being covered, and hydrophilic groups like –OH being introduced into the SPIF/SSPS complexes. This finding is consistent with a previous report in which shielding of the hydrophobic binding site decreased the surface hydrophobicity of the protein.35
 |
| Fig. 4 Surface hydrophobicity of SPIF, SSPS and the SPIF/SSPS complex with different SPIF and SSPS ratios. | |
3.1.5 Dynamic interfacial pressure and adsorption kinetics analysis.
The square root of absorption time (t1/2) dependence of the interfacial pressure (π) for SPIF, SSPS and SPIF/SSPS complexes at the oil–water interface was determined (Fig. 5A), as well as the details of diffusion, penetration and structural rearrangement (Fig. 5B and Table 1). For all samples (Fig. 5A), the interfacial pressure (π) initially increased rapidly, up to about 400 s and then more slowly, which indicates that the initial adsorption of the molecules at the interface is a diffusion-controlled process.21 However, the π10
800 of SSPS (19.59 mN m−1) was significantly lower than the other samples (Table 1). The greater potential of SPIF and SPIF/SSPS complexes to increase the interfacial pressure may be related to the accessible hydrophobic regions on SPIF.23 Although the π10
800 values of SPIF and SPIF/SSPS complexes are not significantly different (Table 1), the increasing trend of π values of the complex with a 5
:
1 ratio was similar to that of SPIF and slightly larger than that at the other ratios. These patterns of variation indicate that a higher proportion of SSPS may shelter the hydrophobic binding sites on SPIF, resulting in lower surface hydrophobicity and a less amount of complex adsorbed at the oil–water interface.24,36
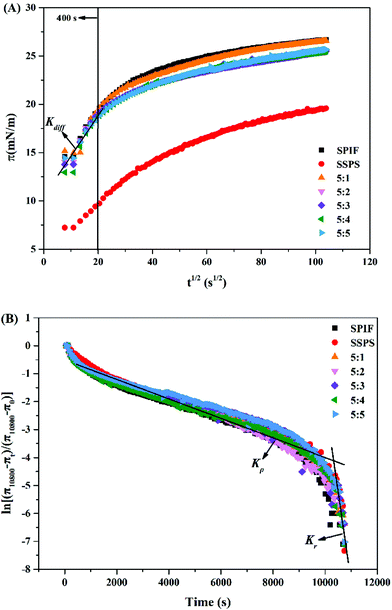 |
| Fig. 5 (A) The square root of time (t1/2) dependence of interfacial pressure (π) of SPIF, SSPS and the SPIF/SSPS complex with different SPIF and SSPS ratio adsorbed layers at the oil-in-water interface. Kdiff represents the diffusion rate. (B) The profile of the molecular penetration and configurational rearrangement steps at the oil–water interface for SPIF, SSPS and the SPIF/SSPS complex with different SPIF and SSPS ratios. Kp and Kr represent the first-order rate constants of penetration and rearrangement, respectively. | |
Table 1 Characteristic parameters, including the apparent diffusion rate (Kdiff), constants of penetration and structural rearrangement at the interface (Kp and Kr), and surface pressure at the end of adsorption (π10
800), of SPIF, SSPS and the SPIF/SSPS complex
|
K
diff (mN m−1 s−0.5) (LR) |
K
p × 104 (LR) |
K
r × 104 (LR) |
π
10 800 (mN m−1) |
Values are given as the means ± SD from triplicate measurements; different characteristics (a–d) on the top of the columns represent the significant difference at p < 0.05. |
SPIF |
0.44 ± 0.014 (0.946)c |
−3.64 ± 0.000(0.976)g |
−24.1 ± 0.000(0.891)a |
26.67 ± 0.132a |
SSPS |
0.22 ± 0.005(0.940)a |
−3.41 ± 0.000(0.977)d |
−27.5 ± 0.002(0.872)e |
19.59 ± 0.124c |
5 : 1 |
0.44 ± 0.017(0.918)c |
−3.46 ± 0.000(0.984)e |
−25.5 ± 0.001(0.913)b |
26.55 ± 0.127a |
5 : 2 |
0.45 ± 0.016(0.930)c |
−3.40 ± 0.000(0.975)f |
−26.2 ± 0.000(0.925)c |
25.45 ± 0.09b |
5 : 3 |
0.48 ± 0.017(0.936)d |
−3.12 ± 0.000(0.956)b |
−29.3 ± 0.000(0.943)d |
25.49 ± 0.063a |
5 : 4 |
0.53 ± 0.018(0.930)e |
−3.25 ± 0.000(0.984)c |
−31.3 ± 0.001(0.934)f |
25.47 ± 0.068a |
5 : 5 |
0.38 ± 0.017(0.943)b |
−3.05 ± 0.000(0.972)a |
−34.5 ± 0.001(0.917)g |
25.67 ± 0.052a |
As shown in Fig. 5B, two linear regions in the curves represent the first-order rate constants of penetration (Kp) and molecular reorganization (Kr).37 Furthermore, in combination with Table 1, the Kdiff value first increases and then decreases with an increase in the proportion of SSPS, while the penetration rate (Kp) of the complex was significantly decreased (p < 0.05). The SPIF/SSPS complex with a 5
:
5 ratio had the smallest Kdiff (0.38 mN m−1 s−0.5) and (Kp) (−3.31 × 10−4 s−1). Xiong et al. concluded that the adsorption kinetics is a complicated process that might be affected by particle size, zeta potential, surface hydrophobicity, etc.21 After the complex with SSPS, the zeta potential, particle size and surface hydrophobicity of SPIF was significantly decreased which synthetically influenced the Kdiff and Kp of SPIF/SSPS complexes, and surface hydrophobicity seems had more significant effects. A similar result was reported regarding the interaction between oxidized bacterial cellulose and soy protein isolate; decreased penetration of protein at the oil–water interface resulted from the formation of a network structure.38 In practice, the interaction between SPIF and SSPS appears to seriously affect the rearrangement of the interface, the Kr values for all samples were considerably higher than the Kp values. The lower hydrophobicity weakened the aggregation among molecules and smaller particle size provides more space for further rearrangement, which led to the Kp increased with the proportion of SSPS in SPIF/SSPS complexes.
3.2 Emulsion characterization
3.2.1 Emulsifying properties.
The emulsifying activity index (EAI) and emulsifying stability index (ESI) of emulsions stabilized by different concentrations of SPIF, SSPS and the SPIF/SSPS complex were determined (Table 2). To better understand the emulsifying properties, the apparent viscosity of all emulsions was also measured and is shown in Fig. 1S.† It is clearly all of the emulsions were typical non-Newtonian fluid and lower viscosity was found in the emulsions stabilized by the SPIF/SSPS complex. The emulsion containing 0.5% SSPS had the lowest EAI (17.99 m2 g−1), but the addition of 1% SSPS increased the EAI to 22.45 m2 g−1, although that was still a relatively low value. However, the EAI values of emulsions stabilized by SPIF/SSPS complexes significantly increased with an increase in the SSPS content (p < 0.05) and there was a similar trend for their ESI values. The emulsion with a SPIF/SSPS ratio of 5
:
5 had the highest EAI (26.17 m2 g−1) and ESI (93.01%) values which were significantly higher than the other emulsions (p < 0.05). These results indicate that the emulsification properties of the complexes are significantly improved by increasing the ratio of SSPS to SPIF.
Table 2 Emulsifying properties of the emulsions stabilized by SPIF, SSPS and the SPIF/SSPS complex with different SPIF and SSPS ratios
Samples |
EAI (m2 g−1) |
ESI (%) |
Values are given as the means ± SD from triplicate measurements; different characteristics (a–d) on the top of the columns represent the significant difference at p < 0.05. |
SPIF0.5 |
23.77 ± 0.023f |
24.36 ± 0.524h |
SPIF1.0 |
25.95 ± 0.040b |
90.56 ± 0.177b |
SSPS0.5 |
17.99 ± 0.012h |
65.74 ± 0.301e |
SSPS1.0 |
22.45 ± 0.023g |
88.53 ± 0.281c |
5 : 1 |
23.83 ± 0.232f |
24.46 ± 0.050h |
5 : 2 |
24.29 ± 0.063e |
38.97 ± 0.267g |
5 : 3 |
24.72 ± 0.052d |
64.58 ± 0.255f |
5 : 4 |
25.62 ± 0.064c |
86.10 ± 0.254d |
5 : 5 |
26.17 ± 0.023a |
93.01 ± 0.137a |
EAI indicates the ability of a surfactant to be absorbed at the O/W interface and to form an emulsion under high-shear conditions. ESI indicates the resistance of the resulting emulsion creaming, coalescence, or flocculation.39 The observed improvement in the emulsifying properties of SPIF appears to be related to the changes in its conformation and physicochemical properties, induced by the addition of SSPS, which make it more amphiphilic and enhance its surface activity, compared with pure SPIF, or SSPS. Notably, the emulsifying properties of SPIF/SSPS at 5
:
1 were very similar to those of 0.5% SPIF, indicating that SPIF dominated the emulsifying properties at the lowest SSPS concentration. The EAI and ESI of the soy protein isolate were improved by conjugation with glucose, using the Maillard reaction, changing the surface activity and conformation of the protein.12
3.2.2 Particle size distribution.
The particle size distribution (Fig. 6A) and volume-weighted mean particle diameter (d4,3) of the emulsion droplets (Fig. 6A, inset table) were determined. Emulsions containing 0.5%, or 1% w/w SSPS had broad size distributions and relatively large mean particle diameters (3.51 and 3.01 μm, respectively; Fig. 6A). Combining their size-distribution data with the presence of large oil droplets in their microscopic images (Fig. 6C), it appears that emulsions containing 0.5% or 1% w/w SSPS were partially phase-separated. Similarly, at low SSPS contents, emulsions exhibited phase separation, indicating that there was insufficient anionic polysaccharides to completely physically separate the droplets.40 In contrast, the particle size of emulsions stabilized by SPIF/SSPS complexes had uniform size-distributions and the mean particle diameter decreased with an increase in the SSPS content; the mean particle sizes of emulsions stabilized by SPIF/SSPS at ratios of 5
:
1–5
:
5 were 2.9, 2.6, 2.55, 2.21 and 1.74 μm, respectively. These mean particle sizes were significantly lower than those of the emulsions with 0.5% w/w SPIF (3.06 μm) (p < 0.05) and all but 5
:
1 were smaller than SPIF at 1% (p < 0.05). These findings were in good agreement with the emulsifying properties and micromorphology observed by light microscopy (Fig. 6C), which indicate that the SPIF/SSPS complexes with higher SSPS concentrations form emulsions with small, uniform droplets. It appears that the surface hydrophilicity/hydrophobicity balance of the complexes changes with an increase in the SSPS content, so that the increasingly amphiphilic complex anchors at the oil–water interface more evenly, strengthens the interfacial layer of the emulsion and stabilizes a smaller particle size.
 |
| Fig. 6 (A) Particle size distributions of emulsions. Inset: Volume-mean diameters of emulsions droplets. (B) Zeta potential of emulsions. Values are given as the means ± SD from triplicate measurements; different characteristics (a–i) on the top or at the bottom of the columns represent the significant difference at p < 0.05. (C) Optical microscopy images of emulsions (scale bar: 10 μm). | |
3.2.3 Zeta potential.
The zeta potentials of emulsions with SPIF, SSPS and SPIF/SSPS complexes with different ratios were determined (Fig. 6B). For the emulsions without SSPS, the zeta potential values were 23.8 and 30.07 mV, respectively, whereas those with SSPS had strongly negative potentials (∼−40 mV). The emulsions stabilized by the SPIF/SSPS complex had an increasingly negative zeta potential as the SSPS content increased, i.e., ranging from −2.43 mV for 5
:
1 to −29.3 mV for 5
:
5 (p < 0.05). It is generally accepted that the zeta potential value represents the electrostatic potential near the particle surface and contributes to electrostatic repulsion among droplets.41 Emulsion droplets with a higher zeta potential repel each other more strongly and are more resistant to coagulate or flocculate.39 For the emulsions without SSPS, the zeta potential values were 23.8 and 30.07 mV, respectively, whereas those with SSPS had strongly negative potentials (∼−40 mV). Even though emulsions stabilized by pure SSPS have a higher negative zeta potential, it is unstable. This phenomenon may result from this unstability, which is derived from oil phase separation (Fig. 6C) rather than aggregation and flocculation and the higher zeta potential may, however, result from the combined potential of adsorbed polysaccharides at the droplet surface and non-absorbed polysaccharides in the bulk aqueous medium, which make no contribution to emulsion stability. The emulsions stabilized by the SPIF/SSPS complex had an increasingly negative zeta potential as the SSPS content increased, i.e., ranging from −2.43 mV for 5
:
1 to −29.3 mV for 5
:
5 (p < 0.05). It is worth noting that although emulsions stabilized by the 5
:
5 ratio complex and 1% w/w pure SPIF have similar absolute values of zeta potential, the microstructures of these two samples possess obvious differences. In the presence of SSPS, no flocculation (Fig. 6C) was observed in the emulsion stabilized by SPIF/SSPS at 5
:
5 ratio, which may contribute to lower surface hydrophobicity and effective steric stabilization provided by the bulky hydrophilic polysaccharide.11
3.2.4 Physical stability.
The Turbiscan stability index (TSI) of the various emulsions was monitored for 24 h (Fig. 7A), and then for a further three weeks (Fig. 7B). A lower TSI corresponds to higher emulsion stability. Emulsions stabilized by SPIF/SSPS complexes at ratios of 5
:
4 and 5
:
5 had the highest stability after three weeks of storage, with final TSI values of 17.86 and 15.14, respectively. After three weeks, emulsions stabilized by SSPS had the highest TSI (36.16 and 38.64) and all the others had TSI values of 25 or more. The TSI values after three weeks correspond well with the visual appearance of the emulsions (Fig. 7C), and the emulsions stabilized by SPIF/SSPS complexes at ratios of 5
:
4 and 5
:
5 being the only ones with no visible separation, and the 5
:
2 and 5
:
3 ratios partially separating to form a less-turbid, rather than a mostly clear lower phase. Comparison with the particle size distributions (Fig. 6A) shows a rough correlation between the particle size and tendency to phase-separate, with the smallest particle size emulsions being the most resistant. The TSI of emulsions stabilized by SPIF0.5 or complexes with lower SSPS contents (5
:
1, 5
:
2, 5
:
3 ratios) increased rapidly during the first 24 hours (Fig. 7A), and then much more slowly. It appears that a relatively high content of SSPS inhibits phase-separation by steric hindrance, i.e., physically separating the oil droplets and without SSPS, phase-separation is extensive.
 |
| Fig. 7 (A) Turbiscan stability index of emulsions within 24 h and (B) three weeks. (C) Visual observations of the emulsions during storage at room temperature for three weeks. | |
Taken together, the above findings demonstrate that emulsion stability can be significantly enhanced by SPIF/SSPS complexes and especially those with a higher SSPS content. The hydrophobic protein region and hydrophilic polysaccharide moiety of SPIF/SSPS complexes with 5
:
5 ratio enable it to be strongly anchored at the oil–water interface, while providing steric hindrance against aggregation.42 In addition, the multiple hydrogen bond donors and flexibility of soy soluble polysaccharides contribute to the formation of inter- and intramolecular hydrogen bonds which help form a compact network to effectively retain oil droplets.
4. Conclusion
In conclusion, the addition of SSPS changed the microstructure and physicochemical property of SPIF mainly via electrostatic and hydrogen bonding interactions, a cooperative effect of them was found in emulsion stabilization. SPIF/SSPS complexes with higher SSPS ratios possessed smaller particle sizes and lower surface hydrophobicity. The adsorption kinetics results showed that the diffusion and penetration rate decreased due to the increase of the polysaccharide region, but increased the reorganization rates of SPIF/SSPS complexes absorbed at the oil/water interface. For the emulsifying properties, the EAI and ESI of the emulsions stabilized by SPIF/SSPS complexes increased significantly upon increasing the SSPS ratio. The emulsion stabilized by a SPIF/SSPS complex at 5
:
5 ratio produced the smallest droplet, and had great potential to improve the physical stability of the emulsion. The bulk hydrophilic polysaccharide region provides efficient steric stabilization that contributes to preventing flocculation, and emulsions stabilized by SPIF/SSPS complexes at 5
:
4 and 5
:
5 ratios had no phase separation after three weeks. Overall, our findings suggest that the combination of SPIF and SSPS has great potential to stabilize emulsions in a food-acceptable manner and at a relatively low cost.
Author contributions
Hekai Zhao: conceptualization, methodology, data curation, and writing-original draft. Shengnan Wang: conceptualization, methodology, data curation, writing-original draft, and supervision. Guilan Zhao: data curation, and software. Yangyang Li: visualization and investigation. Xiulin Liu: validation and software. Lina Yang: software and data curation. Lijie Zhu: investigation and software. He Liu: resources and project administration.
Conflicts of interest
The authors declare no competing financial interests.
Acknowledgements
This work was supported by the National Natural Science Foundation of China (No. 32102005).
References
- T. Sheth, S. Seshadri, T. Prileszky and M. E. Helgeson, Multiple nanoemulsions, Nat. Rev. Mater., 2020, 5, 214–228 CrossRef.
- L. Tian, Y. Kejing, S. Zhang, J. Yi, Z. Zhu, E. A. Decker and D. J. McClements, Impact of tea polyphenols on the stability of oil-in-water emulsions coated by whey proteins, Food Chem., 2021, 343, 128448 CrossRef CAS.
- F. Ravera, K. Dziza, E. Santini, L. Cristofolini and L. Liggieri, Emulsification and emulsion stability: The role of the interfacial properties, Adv. Colloid Interface Sci., 2021, 288, 102344 CrossRef CAS PubMed.
- H. Liu, G. Han, H. Zhang, Q. Liu and B. Kong, Improving the physical and oxidative stability of emulsions based on the interfacial electrostatic effects between porcine bone protein hydrolysates and porcine bone protein hydrolysate-rutin conjugates, Food Hydrocolloids, 2019, 94, 418–427 CrossRef CAS.
- B. Chassaing, O. Koren, J. K. Goodrich, A. C. Poole, S. Srinivasan, R. E. Ley and A. T. Gewirtz, Dietary emulsifiers impact the mouse gut microbiota promoting colitis and metabolic syndrome, Nature, 2015, 519, 92–96 CrossRef CAS PubMed.
- D. J. McClements, L. Bai and C. Chung, Recent Advances in the Utilization of Natural Emulsifiers to Form and Stabilize Emulsions, Annu. Rev. Food Sci. Technol., 2017, 8, 205–236 CrossRef PubMed.
- Y. Pan, Z. Wu, Q. T. Xie, X. M. Li, R. Meng, B. Zhang and Z. Y. Jin, Insight into the stabilization mechanism of emulsions stabilized by Maillard conjugates: Protein hydrolysates-dextrin with different degree of polymerization, Food Hydrocolloids, 2020, 99, 105347 CrossRef CAS.
- G. Liu, W. Li, X. Qin and Q. Zhong, Pickering emulsions stabilized by amphiphilic anisotropic nanofibrils of glycated whey proteins, Food Hydrocolloids, 2020, 101, 105503 CrossRef CAS.
- H. W. Chang, T. B. Tan, P. Y. Tan, F. Abas, O. M. Lai, Y. Wang, Y. Wang, I. A. Nehdi and C. P. Tan, Physical properties and stability evaluation of fish oil-in-water emulsions stabilized u,sing thiol-modified β-lactoglobulin fibrils-chitosan complex, Food Res. Int., 2018, 105, 482–491 CrossRef CAS PubMed.
- X. Pan, Y. Fang, L. Wang, Y. Shi, M. Xie, J. Xia, F. Pei, P. Li, W. Xiong, X. Shen and Q. Hu, Covalent Interaction between Rice Protein Hydrolysates and Chlorogenic Acid: Improving the Stability of Oil-in-Water Emulsions, J. Agric. Food Chem., 2019, 67, 4023–4030 CrossRef CAS PubMed.
- E. Dickinson, Strategies to control and inhibit the flocculation of protein-stabilized oil-in-water emulsions, Food Hydrocolloids, 2019, 96, 209–223 CrossRef CAS.
- R. Li, X. Wang, J. Liu, Q. Cui, X. Wang, S. Chen and L. Jiang, Relationship between Molecular Flexibility and Emulsifying Properties of Soy Protein Isolate-Glucose Conjugates, J. Agric. Food Chem., 2019, 67, 4089–4097 CrossRef CAS PubMed.
- Y. Ding, L. Chen, Y. Shi, M. Akhtar, J. Chen and R. Ettelaie, Emulsifying and emulsion stabilizing properties of soy protein hydrolysates, covalently bonded to polysaccharides: The impact of enzyme choice and the degree of hydrolysis, Food Hydrocolloids, 2021, 113, 106519 CrossRef CAS.
- Y. Wang, Y. Shen, G. Qi, Y. Li, X. S. Sun, D. Qiu and Y. Li, Formation and physicochemical properties of amyloid fibrils from soy protein, Int. J. Biol. Macromol., 2020, 149, 609–616 CrossRef CAS PubMed.
- S. J. Lee, E. Nam, H. J. Lee, M. G. Savelieff and M. H. Lim, Towards an understanding of amyloid-beta oligomers: characterization, toxicity mechanisms, and inhibitors, Chem. Soc. Rev., 2017, 46, 310–323 RSC.
- S. Pang, P. Shao, Q. Sun, C. Pu and W. Tang, Relationship between the emulsifying properties and formation time of rice bran protein fibrils, LWT, 2020, 122, 108985 CrossRef CAS.
- Z. Wan, X. Yang and L. M. Sagis, Nonlinear Surface Dilatational Rheology and Foaming Behavior of Protein and Protein Fibrillar Aggregates in the Presence of Natural Surfactant, Langmuir, 2016, 32, 3679–3690 CrossRef CAS PubMed.
- J. Peng, J. R. Simon, P. Venema and E. van der Linden, Protein Fibrils Induce Emulsion Stabilization, Langmuir, 2016, 32, 2164–2174 CrossRef CAS PubMed.
- Y. T. Xu and L. L. Liu, Structural and Functional Properties of Soy Protein Isolates Modified by Soy Soluble Polysaccharides, J. Agric. Food Chem., 2016, 64, 7275–7284 CrossRef CAS PubMed.
- A. Schröder, C. Berton-Carabin, P. Venema and L. Cornacchia, Interfacial properties of whey protein and whey protein hydrolysates and their influence on O/W emulsion stability, Food Hydrocolloids, 2017, 73, 129–140 CrossRef.
- W. Xiong, J. Li, B. Li and L. Wang, Physicochemical properties and interfacial dilatational rheological behavior at air-water interface of high intensity ultrasound modified ovalbumin: Effect of ionic strength, Food Hydrocolloids, 2019, 97, 105210 CrossRef CAS.
- A. F. H. Ward and L. Tordai, Time Dependence of Boundary Tensions of Solutions I. The Role of Diffusion in Time Effects, J. Chem. Phys., 1946, 14, 453–461 CrossRef CAS.
- J. M. Wang, N. Xia, X. Q. Yang, S. W. Yin, J. R. Qi, X. T. He, D. B. Yuan and L. J. Wang, Adsorption and dilatational rheology of heat-treated soy protein at the oil-water interface: relationship to structural properties, J. Agric. Food Chem., 2012, 60, 3302–3310 CrossRef CAS PubMed.
- W. Xiong, C. Ren, M. Tian, X. Yang, J. Li and B. Li, Emulsion stability and dilatational viscoelasticity of ovalbumin/chitosan complexes at the oil-in-water interface, Food Chem., 2018, 252, 181–188 CrossRef CAS PubMed.
- S. Wang, J. Yang, G. Shao, J. Liu, J. Wang, L. Yang, J. Li, H. Liu, D. Zhu, Y. Li and L. Jiang, pH-induced conformational changes and interfacial dilatational rheology of soy protein isolated/soy hull polysaccharide complex and its effects on emulsion stabilization, Food Hydrocolloids, 2020, 109, 106075 CrossRef CAS.
- H. Liu, Y. Li, X. Diao, B. Kong and Q. Liu, Effect of porcine bone protein hydrolysates on the emulsifying and oxidative stability of oil-in-water emulsions, Colloids Surf., A, 2018, 538, 757–764 CrossRef CAS.
- Y. H. Wang, Z. L. Wan, X. Q. Yang, J. M. Wang, J. Guo and Y. Lin, Colloidal complexation of zein hydrolysate with tannic acid: Constructing peptides-based nanoemulsions for alga oil delivery, Food Hydrocolloids, 2016, 54, 40–48 CrossRef CAS.
- X. Ding and P. Yao, Soy protein/soy polysaccharide complex nanogels: folic acid loading, protection, and controlled delivery, Langmuir, 2013, 29, 8636–8644 CrossRef CAS PubMed.
- F. P. Chen, S. Y. Ou and C. H. Tang, Core-Shell Soy Protein-Soy Polysaccharide Complex (Nano)particles as Carriers for Improved Stability and Sustained Release of Curcumin, J. Agric. Food Chem., 2016, 64, 5053–5059 CrossRef CAS PubMed.
- L. Shang, Y. Z. Wang, J. G. Jiang and S. J. Dong, pH-Dependent Protein Conformational Changes in Albumin: Gold Nanoparticle Bioconjugates: A Spectroscopic Study, Langmuir, 2007, 23, 2714–2721 CrossRef CAS PubMed.
- H. S. Zhao, Z. Ma and P. Jing, Interaction of soy protein isolate fibrils with betalain from red beetroots: Morphology, spectroscopic characteristics and thermal stability, Food Res. Int., 2020, 135, 109289 CrossRef CAS PubMed.
- D. Salarbashi, M. Tafaghodi, B. S. F. Bazzaz and B. Jafari, Characterization of soluble soybean (SSPS) polysaccharide and development of eco-friendly SSPS/TiO2 nanoparticle bionanocomposites, Int. J. Biol. Macromol., 2018, 112, 852–861 CrossRef CAS PubMed.
- C. Yuan, M. Chen, J. Luo, X. Li, Q. Gao and J. Li, A novel water-based process produces eco-friendly bio-adhesive made from green cross-linked soybean soluble polysaccharide and soy protein, Carbohydr. Polym., 2017, 169, 417–425 CrossRef CAS PubMed.
- E. Ansarifar, F. Shahidi, M. Mohebbi, N. Ramezanian, A. Koocheki and A. Mohamadian, Optimization of limonene microencapsulation based on native and fibril soy protein isolate by VIKOR method, LWT, 2019, 115, 107884 CrossRef CAS.
- S. Ren, L. Liu, Y. Li, H. Qian, L. Tong, L. Wang, X. Zhou, L. Wang and S. Zhou, Effects of carboxymethylcellulose and soybean soluble polysaccharides on the stability of mung bean protein isolates in aqueous solution, LWT, 2020, 132, 109927 CrossRef CAS.
- S. Wang, J. Yang, G. Shao, D. Qu, H. Zhao, L. Zhu, L. Yang, R. Li, J. Li, H. Liu and D. Zhu, Dilatational rheological and nuclear magnetic resonance characterization of oil-water interface: Impact of pH on interaction of soy protein isolated and soy hull polysaccharides, Food Hydrocolloids, 2020, 99, 105366 CrossRef CAS.
- N. A. Camino, O. E. Pérez, C. C. Sanchez, J. M. R. Patino and A. M. R. Pilosof, Hydroxypropylmethylcellulose surface activity at equilibrium and adsorption dynamics at the air–water and oil–water interfaces, Food Hydrocolloids, 2009, 23, 2359–2368 CrossRef CAS.
- X. Zhang, Y. Lei, X. Luo, Y. Wang, Y. Li, B. Li and S. Liu, Impact of pH on the interaction between soybean protein isolate and oxidized bacterial cellulose at oil-water interface: Dilatational rheological and emulsifying properties, Food Hydrocolloids, 2021, 115, 106609 CrossRef CAS.
- Y. Li, H. Liu, Q. Liu, B. Kong and X. Diao, Effects of zein hydrolysates coupled with sage (salvia officinalis) extract on the emulsifying and oxidative stability of myofibrillar protein prepared oil-in-water emulsions, Food Hydrocolloids, 2019, 87, 149–157 CrossRef CAS.
- J. Zhao, T. Wei, Z. Wei, F. Yuan and Y. Gao, Influence of soybean soluble polysaccharides and beet pectin on the physicochemical properties of lactoferrin-coated orange oil emulsion, Food Hydrocolloids, 2015, 44, 443–452 CrossRef CAS.
- Y. Wei, Y. Xie, Z. Cai, Y. Guo and H. Zhang, Interfacial rheology, emulsifying property and emulsion stability of glyceryl monooleate-modified corn fiber gum, Food Chem., 2021, 343, 128416 CrossRef CAS PubMed.
- G. Liu and Q. Zhong, Glycation of whey protein to provide steric hindrance against thermal aggregation, J. Agric. Food Chem., 2012, 60, 9754–9762 CrossRef CAS PubMed.
Footnote |
† Electronic supplementary information (ESI) available. See DOI: 10.1039/d1fo01604k |
|
This journal is © The Royal Society of Chemistry 2022 |