Cobalt nanoparticle–carbon nanoplate as the solar absorber of a wood aerogel evaporator for continuously efficient desalination†
Received
20th August 2021
, Accepted 11th November 2021
First published on 12th November 2021
Abstract
Solar-driven interface water evaporation has shown great potential in seawater desalination. A solar steam generation device with excellent performance requires materials that can efficiently absorb solar energy to achieve photothermal conversion. ZIF-L (a Co-based metal–organic framework) after carbonization has the dual role of carbon nanoplates and cobalt nanoparticles to absorb solar light, exhibiting broad light absorption. Herein, based on carbonized ZIF-L and natural wood, we designed a solar-driven interface water evaporation device, in which ZIF-L after carbonization is used to achieve photothermal conversion, and wood aerogel with high porosity is used as a supporting layer to transport seawater to the evaporation interface and realize self-floating of the device. The prepared evaporator exhibited a high evaporation rate of 1.52 kg m−2 h−1 and an evaporation efficiency of 92.42% under 1 sun illumination, and neither salt accumulation nor a significant decrease in the evaporation rate of the device was observed after continuous operation for 10 evaporation cycles. Additionally, the excellent resistance to salt deposition and automatic salt discharge ability of the evaporator were demonstrated, which can ensure its long-term stable operation. The high-efficiency and stable evaporation performance demonstrate the evaporator's great potential in the practical application of solar energy production of clean water.
Water impact
Solar-driven evaporation provides a feasible way to produce clean water and solve the problem of water shortage. It is of great significance to further develop solar-driven interface evaporation devices that are salt-tolerant and can achieve high-efficiency photothermal conversion. We have designed a solar interface evaporation device based on carbon nanoplate–cobalt nanoparticles from a carbonized Co-metal–organic framework and wood aerogel, which has excellent salt resistance and self-salt discharge capacity with high evaporation efficiency.
|
Introduction
With the rapid development of industry, shortage of fresh water resources has become one of the main problems hindering the sustainable development of modern society.1–3 The storage of seawater on the earth is huge, so the production of freshwater from seawater has aroused widespread interest in society.4,5 In response to this solution, environmental workers have explored a variety of feasible methods, such as reverse osmosis,6,7 nanofiltration,8,9 electrodialysis10 and multiple effect distillation.11 However, these methods without exception require a lot of energy and also increase greenhouse gas emissions, making them unsuitable for large-scale production of fresh water.12 Thus the development of techniques to produce fresh water with low energy consumption and environmental protection is needed. In the past decades, photothermal desalination has been regarded as one of the most promising sustainable and low-cost techniques because, in theory, solar energy is an inexhaustible and easily available renewable energy source.13–15 However, the traditional device that uses solar energy to generate steam directly heats a large volume of water, which generates a large amount of heat loss due to the inability to concentrate heat, resulting in low production efficiency. To this end, an emerging solar-driven interface water evaporation technology has drawn great attention in recent years.16–19 Interface evaporation concentrates heat on the gas–liquid interface, which can effectively reduce heat loss and improve the efficiency of light-to-heat conversion. Therefore, the development of high-efficiency solar-driven interface evaporators is a green and energy-saving effective way to solve the problem of shortage of fresh water resources.
A solar-driven interface evaporation device is usually composed of a photothermal layer and a hydrophilic supporting layer. The photothermal layer can absorb solar radiation and convert it into heat energy, and the hydrophilic supporting layer can transport water from the water body to the evaporation interface to achieve continuous steam generation. Based on the above viewpoints, an excellent evaporation device needs to meet the following requirements:20–26 (1) the photothermal layer has broadband light absorption and high light-to-heat conversion efficiency; (2) the supporting layer has low thermal conductivity to reduce the heat loss from the evaporation interface to the water body; (3) the supporting layer has a porous structure with good hydrophilicity to achieve continuous and effective water transport to the evaporation interface; (4) the entire evaporation device needs to be light enough to ensure that it can float on water; (5) the evaporation device needs to have good mechanical strength and resistance to salt deposition in order to achieve its long-term, stable and efficient operation. So the material choice of the photothermal layer and supporting layer as well as the modulation of the interaction between them is of great importance to achieve high efficiency and stable evaporation performance.
So far, many materials have been be applied into the photothermal layer to achieve high light-to-heat conversion, such as gold and silver nanoparticles,27,28 carbon-based materials,29,30 metal oxides31,32 and MXene.33,34 However, some inherent problems of these photothermal materials have hindered their further development. For example, the high cost of gold and MXene nanomaterials limits their commercial development. Silver nanoparticles and non-biodegradable synthetic polymers that are easily detached from the membrane structure may cause additional negative effects on the environment.35 In addition, the salt deposition on the surface of the photothermal layer during the evaporation process blocks the long-term stable operation of most evaporation devices.12,36–38 Therefore, it is of great significance to further develop environment-friendly solar-driven interface evaporation devices that are salt-tolerant and can achieve high-efficiency photothermal conversion.
In this work, we used a cobalt-based metal–organic framework named ZIF-L as the precursor to obtain cobalt nanoparticle–carbon nanoplate (Co–CN) as a light absorber, combined with porous aerogels derived from natural wood as the supporting material for a wood aerogel evaporator (Co–CN–WA), to achieve continuous, efficient and stable solar-driven interface evaporation. This evaporator has several key features. First, the rough surface of the wood aerogel itself can inhibit the reflection of incident light through multiple light scattering and reflection, thereby enhancing the absorption of sunlight. Second, the high photothermal conversion efficiency is the result of the joint action of the plasma local heating of cobalt nanoparticles in the photothermal layer under illumination and the thermal vibration of the carbon atoms. Third, the porous structure of the aerogel can effectively pump water to the evaporation interface, replenish water for the evaporation interface to realize the exchange of concentrated brine and seawater, reduce the concentration of brine at the evaporation interface, and prevent salt deposition, so as to achieve the efficient operation of the evaporator. Fourth, the evaporator is derived from natural wood, which has the features of low cost and sustainability. Based on these key features, the evaporator shows good light absorption in the spectral range of 250–2500 nm. Under the sunlight intensity of 1 kW m−2 (1 sunlight), the evaporator can reach an evaporation rate of 1.52 kg m−2 h−1 and a photothermal conversion efficiency of 92.42%, and in the meantime there is no salt deposit on the surface during evaporation.
Results and discussion
Preparation and characterization of the evaporator
We successfully fabricated a wood aerogel evaporation device Co–CN–WA based on cobalt nanoparticle–carbon nanoplate on the surface of the wood aerogel (WA) using sodium alginate as a binder (Fig. 1). After removing the lignin and hemicellulose, the colour of the wood changes from light yellow to pure white (Fig. 2a and b) with the density decreasing from the original 0.15 g cm−3 to 0.06 g cm−3 (Fig. S1†), which allows the evaporator to achieve long-term floating on the water. As shown in Fig. 2c, the lower part of the Co–CN–WA evaporator is a white aerogel used to pump seawater to the photothermal layer, and the upper layer is the coating of Co–CN with approximately 1 mm thickness for photothermal conversion.
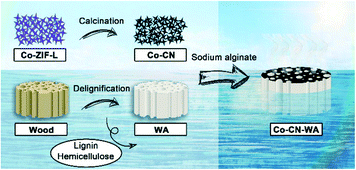 |
| Fig. 1 Construction of the Co–CN–WA evaporator. | |
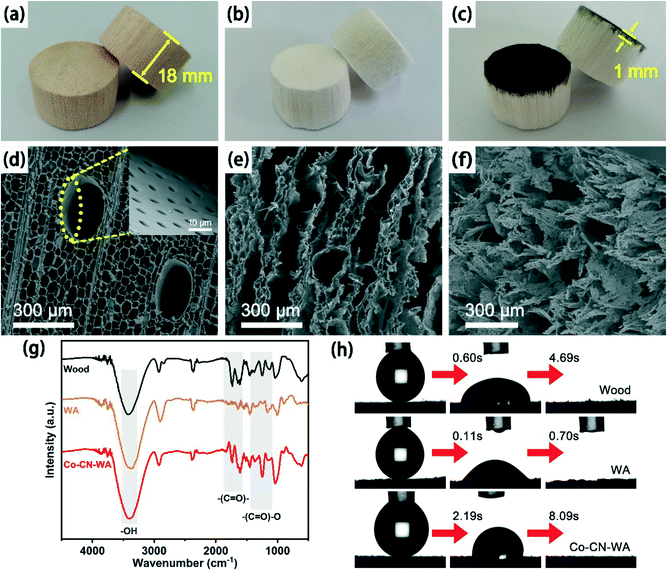 |
| Fig. 2 Optical photos of the (a) balsa wood, (b) WA and (c) Co–CN–WA. SEM images of the balsa wood showing the narrow conduits and wider large channels (d), WA showing a layered structure (e) and Co–CN–WA showing a rough surface (f). FT-IR spectra of the wood, WA and Co–CN–WA (g). The water contact angle images of the wood, WA and Co–CN–WA at different moments (h). | |
SEM was used to observe the surface morphology and structure of the wood, WA and Co–CN–WA. As shown in Fig. 2d, the wood has a three-dimensional porous structure composed of narrow conduits (pore size 30–40 μm) and wider large channels (pore size 200–300 μm) along the direction of wood growth. In addition, there are many mesopores named pits with a size of 3–5 μm on the wall of the macroporous channels of the wood, which can transport water along the horizontal direction (illustration in Fig. 2d).39 These pits provide pathways for the diffusion of salt ions from the concentrated brine at the upper layer to the seawater at the bottom together with the large channels, thus preventing salt crystals from depositing on the evaporation interface. After removing the lignin, WA retains the original conduits and large channels while expanding the channel size, forming a layered structure, constituting a highly porous matrix, which is conducive to efficient water transport (Fig. 2e). The porous structure of WA also gives it low thermal conductivity (Fig. S2†), which is beneficial to reducing the heat loss from the evaporation interface to the water body during the evaporation process, and improving the evaporation rate and efficiency.
FT-IR spectroscopy was used to detect the changes of surface functional groups in the wood, WA and Co–CN–WA. As shown in Fig. 2g, the peak intensities of the C
O group vibration (1550–1750 cm−1) and (C
O)–O group vibration (1200–1500 cm−1) of WA are significantly reduced compared with those of the wood, indicating the successful removal of lignin and hemicellulose. The absorption peaks of the C
O group vibration (1550–1750 cm−1) and (C
O)–O group vibration (1200–1500 cm−1) of Co–CN–WA can be attributed to sodium alginate. At the same time, the wood, WA and Co–CN–WA all have a broadband absorption peak of the OH group at 3300–3500 cm−1, which proves the hydrophilic character.40–42 In order to further verify the hydrophilicity of the materials, we dropped a drop of water on the surface of the wood, WA and Co–CN–WA, respectively, to observe the process of their penetration. As shown in Fig. 2h, the water droplet on the WA surface completely penetrates into the material within 0.70 s, which is 4.7 times faster than that on the wood (4.69 s). After the Co–CN photothermal layer is loaded on the surface, the hydrophilicity of the top surface of Co–CN–WA decreases, but the lower part still maintains the high hydrophilicity as WA. This feature can realize the high water transport ability of the evaporator from the bulk water to the evaporation interface.
The photothermal characteristic of the Co–CN–WA evaporation device is caused by two aspects. The rough surface of Co–CN–WA is beneficial to suppressing the reflection of incident light through multiple light reflections and scattering, resulting in enhanced solar light absorption across a broad spectrum of wavelengths (Fig. 2f). In addition, after calcination, the cobalt ion liberated from ZIF-L is reduced to ultrafine cobalt nanoparticles, and the organic ligands are converted into amorphous carbon nanoplates at the same time.34,43,44 The high light absorption of the photothermal layer is realized through the plasmon resonance generated by the cobalt nanoparticles and the thermal vibration of carbon atoms under sunlight irradiation. The presence of metallic cobalt is confirmed by the occurrence of the characteristic peak at 44.3° in the PXRD pattern of Co–CN (Fig. 3d).44,45 SEM and TEM were used to observe the morphology and structure of Co–CN. Fig. 3a shows that Co–CN maintains the shape of cross leaf of ZIF-L, with an average size of 5–8 μm. In the TEM image of Co–CN (Fig. 3b), a large number of black nanodots, which are assigned to cobalt nanoparticles, are uniformly distributed on Co–CN. As shown in Fig. 3c, the cobalt nanoparticles with an average size of 6–10 nm are segregated in the carbon framework because the cobalt source is molten and local agglomeration is gradually formed at high temperature. The lattice distance of 0.21 nm corresponds to Co (111). The element mapping in Fig. 3e further demonstrates the uniform distribution of Co and C on Co–CN. The surface chemistry of Co–CN was examined by XPS. The survey spectrum demonstrates the existence of Co, O, N and C in Co–CN (Fig. 4a). The two peaks in the high-resolution Co 2p spectrum, located at 778.4 and 780.0 eV, are assigned to metallic Co and Co–N (Fig. 4b). The high-resolution XPS spectrum of N 1s can be divided into two peaks, corresponding to pyridinic N (398.6 eV) and graphitic N (400.9 eV) (Fig. 4c). In addition, the fitted C1s spectrum shows a main peak at around 284.8 eV, corresponding to sp2 carbon, and two peaks at 285.8 and 287.6 eV, assigned to C
N bonds and C–N species, respectively (Fig. 4d).34,43–46 These aforementioned characterizations confirm the presence of cobalt nanoparticles and amorphous carbon nanoplates in Co–CN.
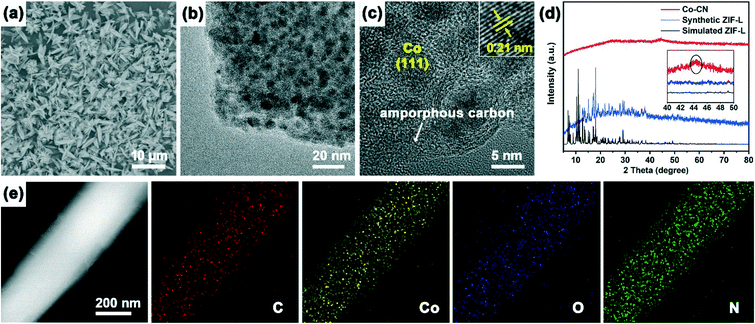 |
| Fig. 3 (a) SEM image of Co–CN. (b) TEM image of Co–CN. (c) High-resolution TEM image of Co–CN. (d) PXRD patterns of ZIF-L and Co–CN. (e) Element mapping of Co–CN. | |
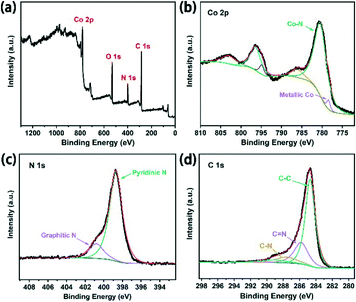 |
| Fig. 4 (a) XPS survey spectrum and (b) Co 2p, (c) N 1s, and (d) C 1s high-resolution XPS spectra of Co–CN. | |
Evaporation performance test
In order to evaluate the light absorption capacity of Co–CN–WA, we measured the UV-vis-NIR absorption spectra of the wood, WA and Co–CN. The wood has poor light absorption performance. Due to the removal of light-absorbing lignin, WA exhibits lower light absorption performance than the wood. Meanwhile, Co–CN shows strong light absorption in the whole wavelength range (Fig. 5a). To further verify the photothermal conversion capability of Co–CN–WA, we recorded the temperature changes on the upper surface of the wood, WA and Co–CN–WA evaporator under 1 sunlight irradiation. As shown in Fig. 5b, the surface temperature of the wood increases from 26.6 to 61.2 °C after 15 min and the surface temperature of WA increases from 29.9 °C to 53.8 °C. In contrast, the surface temperature of Co–CN–WA rises rapidly from 30.4 °C to 80 °C within 1 min, then continues to rise and stabilizes at around 99.6 °C, showing the excellent photothermal conversion ability.
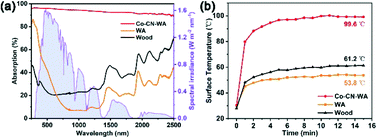 |
| Fig. 5 (a) Light absorbance of natural wood, WA and Co–CN–WA in the 250–2500 nm wavelength range. The purple shaded part shows the normalized spectral solar irradiance density of air mass 1.5 global (AM 1.5 G) tilt solar spectrum. (b) The surface temperatures of the wood, WA and Co–CN–WA at different times under 1 sunlight irradiation. | |
To test the evaporation performance of Co–CN–WA, we conducted seawater evaporation experiments under 1 sunlight irradiation using a solar simulator to simulate the real conditions to the greatest extent. Fig. 6 records the changes in surface temperature, mass change, and evaporation rate of blank seawater, WA and Co–CN–WA over time within 1 h, and the evaporation efficiency is calculated at the same time. Because the thermal conductivity of WA under wet conditions is higher than that under dry conditions (Fig. S2†), the heat loss of the top surface of the evaporator under wet conditions is faster than that under dry conditions, resulting in the lower temperature of the top surface of the evaporator during the evaporation process in seawater. As shown in Fig. 6a, the surface temperatures of seawater, WA, and Co–CN–WA reach the equilibrium temperatures of 31.4, 32.8, and 41.5 °C, respectively, after evaporation for 15 min. The thermal infrared images of the evaporation process are shown in Fig. 6b. The mass change curves show that the mass loss per unit area of the seawater with the Co–CN–WA evaporator is much higher than that of the seawater with WA and blank seawater (Fig. 6c). The evaporation rate of the Co–CN–WA evaporator (1.52 kg m−2 h−1) is much higher than that of seawater (0.50 kg m−2 h−1) and WA (1.01 kg m−2 h−1) when the evaporation reaches equilibrium, which is about 3 times that of seawater and 1.5 times that of WA (Fig. 6d). The evaporation efficiency is calculated by eliminating the inherent evaporation under dark conditions. As shown in Fig. 6e, the evaporation efficiency of seawater and WA is 28.65% and 60.02%, respectively, while the evaporation efficiency of the Co–CN–WA evaporator is 92.42%, which is much higher than that of blank seawater and WA. We used the same method to make a Zn–ZIF-L based evaporator (CN–WA), and performed the evaporation experiment under 1 sunlight. For the structure of Zn–ZIF-L, Zn atoms are volatilized at high temperature and only the porous carbon framework remains.43 The evaporation rate of CN–WA being lower than that of Co–CN–WA proves the role of cobalt nanoparticles in photothermal conversion (Fig. S5†).
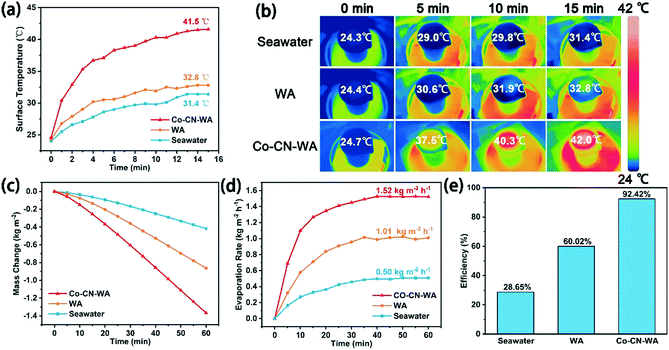 |
| Fig. 6 (a) The surface temperatures of Co–CN–WA, WA and blank seawater evaporation system at different times under 1 sunlight. (b) Thermal infrared images of Co–CN–WA, WA and blank seawater evaporation system at different times under 1 sunlight. (c) Water quality losses of Co–CN–WA, WA and blank seawater evaporation system at different times under 1 sunlight. (d) Changes of evaporation rate of Co–CN–WA, WA and blank seawater evaporation system under1 sunlight. (e) Average efficiency of Co–CN–WA, WA and blank seawater evaporation system under1 sunlight. | |
We summarized the evaporation performance of solar-driven interface evaporators reported in recent years, and found that the Co–CN–WA evaporator has excellent evaporation performance comparable to that previously reported (Fig. 7a).33,36,41,47–52 The high-efficiency evaporation of the Co–CN–WA evaporator is mainly owing to the excellent photothermal conversion ability of the photothermal layer and the thermal insulation performance of the supporting layer. These features can confine the heat to the evaporation interface, significantly reduce heat loss, and improve the evaporation efficiency accordingly.
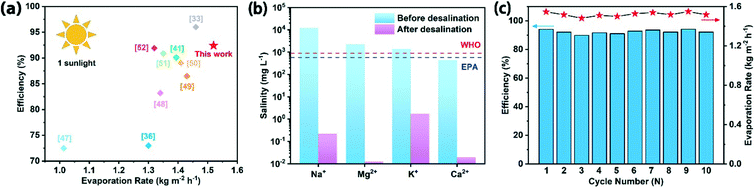 |
| Fig. 7 (a) Comparison of evaporation rates and efficiencies between the Co–CN–WA and previous reports when under 1 sunlight. (b) Concentrations of Na+, Mg2+, K+, and Ca2+ in seawater and clean water collected after evaporation with Co–CN–WA. (c) The evaporation rate of the Co–CN–WA evaporator for 10 cycles of testing under 1 sunlight. | |
An inductively coupled plasma mass spectrometer was used to determine the concentration of Na+, K+, Mg2+ and Ca2+ ions in water before and after desalination using the Co–CN–WA evaporator. After desalination, the concentrations of Na+, Mg2+, K+, and Ca2+ ions decrease from 11
856.29, 2233.22, 1404.25 and 445.08 mg L−1 to 0.22, 0.01, 1.73 and 0.02 mg L−1, respectively, with the removal rates being higher than 99.99%. Furthermore, after desalination, the concentration of these ions is lower than the drinking water standards jointly established by the WHO and US-EPA (Fig. 7b).
The stable operation of the evaporator is an important factor in practical applications. After 10 cycles of evaporation experiments, the Co–CN–WA evaporator can still maintain a high evaporation rate (Fig. 7c). In order to further verify the stability of the Co–CN–WA evaporator, the evaporation performance of the Co–CN–WA evaporator was measured after immersing it in seawater for a week. As shown in Fig. S6,† neither the evaporation rate (1.51 kg m−2 h−1) nor efficiency (91.57%) of the Co–CN–WA evaporator decreases significantly, indicating that Co–CN–WA has good stability in seawater. Thermogravimetric analysis shows that the temperature at which the Co–CN–WA evaporator begins to degrade is 230 °C, which promises structural stability at the working temperature (Fig. 8a). The solution stability of the evaporator in seawater comes from the alkali resistance of cellulose,23 which is the main component of WA derived from wood.
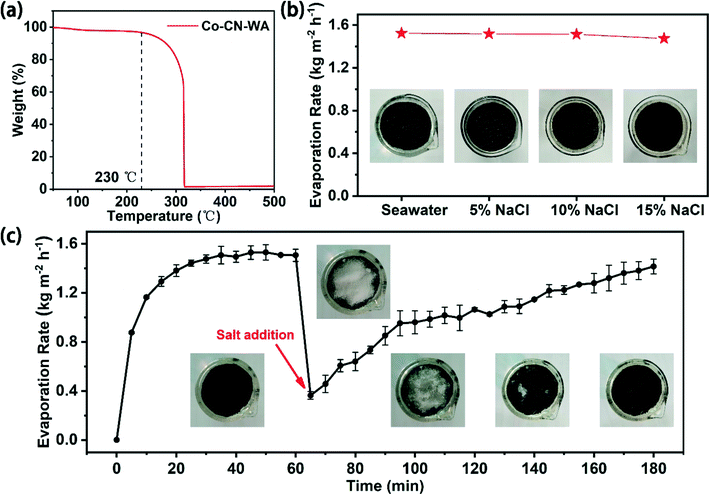 |
| Fig. 8 (a) TGA curve of Co–CN–WA measured from 50 °C to 500 °C with a heating speed of 10 °C min−1. (b) The evaporation rate of Co–CN–WA in seawater, 5 wt%, 10 wt% and 15 wt% NaCl solution under 1 sunlight. (c) The evaporation rate of Co–CN–WA in seawater under 1 sunlight; the illustrations are the photos of the NaCl solid on the surface of the Co–CN–WA evaporator. | |
Salt tolerance and automatic salt discharge performance
In practical applications, the salt deposition resistance and durability of the evaporator are two key conditions. To verify the resistance to salt deposition of the Co–CN–WA evaporator, we tested the evaporation rate of Co–CN–WA in seawater, 5 wt%, 10 wt% and 15 wt% NaCl solution under 1 sunlight, respectively. The result shows that when the evaporation reaches stability, the evaporation rate of the Co–CN–WA evaporator reaches 1.52, 1.52, 1.51 and 1.49 kg m−2 h−1 respectively, and remains at a stable level. It is notable that during the evaporation process, there are no salt deposits on the surface of the evaporator (Fig. 8b). In addition, we placed 1.0 g NaCl solid on the surface of the Co–CN–WA evaporator after evaporation for 1 h, and continued to evaporate under 1 sunlight. The evaporation rate and the change of the NaCl solid on the surface of the evaporator over time were recorded. As shown in Fig. 8c, before placing the NaCl solid, the evaporation rate of Co–CN–WA gradually increases to an equilibrium state. After placing the NaCl solid, the evaporation rate drops rapidly to a very slow state since the evaporation interface is covered with salt particles. But with time increasing, the NaCl solid gradually dissolves to expose the evaporation interface, and the evaporation rate increases. Surprisingly, after evaporating for 2 h, the NaCl solid on the surface of the evaporator disappears completely, and the evaporation rate returns to the highest value. During the evaporation process, the macroporous channels of WA pump water to the evaporation interface continuously, to realize the rapid replenishment of water on the evaporation interface. In addition, the pits on the wall of the macroporous channels can realize water transmission, diffusion and convection along the horizontal direction, and transport high concentration brine in narrow conduits to nearby large channels, increasing the capacity for salt exchange with the bulk water. These features effectively avoid the deposition of salt crystals on the evaporation interface.38
Outdoor evaporation performance
In order to verify the evaporation performance of the Co–CN–WA evaporator under natural conditions, we conducted an outdoor evaporation experiment on the roof of a building in Hainan University (13 July 2021, sunny, from 9:00 AM to 6:00 PM). The changes in solar radiation intensity in this area, evaporator surface temperature and evaporation rate within a day were recorded. As shown in Fig. 9, the solar radiation intensity varies in the range of 400–1000 W m−2. The surface temperature and evaporation rate of the Co–CN–WA evaporator have the same changing trend as the radiation intensity. The evaporation rate can reach 1.6 kg m−2 h−1 at the time when the evaporation is strongest in a day, which is higher than the results measured in the laboratory. This difference is caused by the occasional high solar radiation intensity (>1000 W m−2) and the extra heat provided to the evaporator by thermal radiation from the ground during the day. According to calculation, the average daily water output of the Co–CN–WA evaporator reaches 1.41 kg m−2 h−1 under natural conditions in the daytime. This result reveals that the Co–CN–WA evaporator has the potential for practical application.
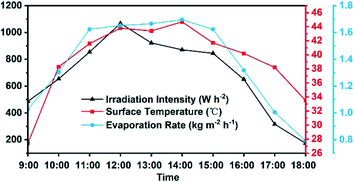 |
| Fig. 9 Outdoor evaporation performance (13 July 2021, sunny). | |
Experimental
Materials
Natural balsa wood was purchased from Alibaba Zhuhai, Guangdong. Seawater was taken from Haikou City, Hainan Province, China (South China Sea). Sodium hydroxide (NaOH, ≥96%, Guangdong Canton Test Agent Technology), sodium sulfite (Na2SO3, ≥97%, Guangdong Canton Test Agent Technology), hydrogen peroxide (H2O2, 30%, Guangzhou Yilian Chemical Industry), and deionized water were used for processing balsa wood. Cobaltous nitrate hexahydrate (Co(NO3)2·6H2O, 99%, Shanghai Macklin Biochemical Co., Ltd) and 2-methylimidazole (98%, Shanghai Macklin Biochemical Co., Ltd) were used for preparing Co-MOF (ZIF-L). Sodium alginate (CP, Sinopharm Chemical Reagent Co., Ltd) was used as a binder.
Preparation
The preparation process is shown in Fig. 1. Typically, an 18 mm thick cylindrical piece was cut from balsa wood along the growth direction. The cylindrical block was soaked in a mixed solution of NaOH (2.5 mol L−1) and Na2SO3 (0.4 mol L−1) and heated at 150 °C for 12 h. After that, the sample was washed with hot deionized water (80 °C) to remove the attached chemicals. Then, the ginger sample was soaked in a solution of H2O2 (1.5 mol L−1) and heated at 120 °C for 1.5 h to remove residual lignin. WA was obtained by freeze-drying in a desiccator for 48 h.
0.552 g Co(NO3)2·6H2O and 1.303 g 2-methylimidazole were respectively dissolved in 40 mL deionized water. The two solutions were mixed together at room temperature and stirred magnetically for 90 min. The obtained precipitates were collected by centrifugation, and then washed with deionized water three times. Then, the precipitates were dried at 60 °C overnight to obtain purple powder (ZIF-L). ZIF-L was annealed under Ar at 450 °C for 2 h at a ramp rate of 2 °C min−1 to obtain black powder (Co–CN). Co–CN and sodium alginate were dispersed in deionized water with a mass ratio of 5
:
1, and magnetically stirred for 6 h to obtain a homogeneous suspension. Finally, the obtained suspension was spread on the surface of WA evenly and allowed for gelation for 2 h. In this process, sodium alginate combined Co–CN and WA tightly to obtain stable Co–CN–WA.
Characterization
The morphology investigation was performed with a scanning electron microscope (SEM, PHENOM SCIENTIFIC ProX G5, Netherlands) and a transmission electron microscope (TEM, FEI Tecnai G2 F30, USA). The surface properties of the wood, WA and Co–CN–WA were analyzed using a Fourier transform infrared spectrometer (FT-IR, Nicolet 6700 IR, USA). The wetting dynamic evolution of water droplets was captured by an optical contact angle measuring instrument (Biolin Theta Flex, Sweden). The powder X-ray diffraction (PXRD) patterns of the samples were measured with a Miniflex 600 diffractometer (Rigaku, Japan). The elements present and the chemical state of the elements on the surface of the sample were measured using an X-ray photoelectron spectrometer (XPS, Thermo escalab 250Xi). A UV-visible-NIR spectrophotometer (PerkinElmer Lambda 750S, USA) was used to measure the absorption with an integrating sphere in the wavelength range of 250–2500 nm. The temperature distribution of the evaporator surface was observed with a forward-looking infrared camera (FLIR ONE PRO, USA). The thermogravimetric behavior of the prepared samples was investigated using a TG-DTA8122 micro-analyzer (Rigaku, Japan) from 50 to 500 °C with a heating rate of 10 °C min−1 in an atmospheric environment. The ion concentrations of the seawater and desalted water were evaluated with an inductively coupled plasma mass spectrometer (ICP-MS, Agilent ICPOES 730, USA).
Evaporation performance test
The experiment was carried out under a solar simulator (Perfectlight CHF-XM-500 W, China), with an optical filter for the standard AM-1.5 spectrum. An optical power meter (NBeT FZ400, China) was used to make sure that the optical density simulates sunlight. A piece of the Co–CN–WA evaporator with a diameter of 2.9 cm was placed in the center of the beaker with the photothermal layer floating above the water. An electronic balance (SARTORIUS GL124-1SCN, Germany) was used to monitor the mass loss of brine during the evaporation process. The solar-to-vapor conversion efficiency (η) was calculated using the following equation (eqn (1)).53 |  | (1) |
where η (%) is the evaporation efficiency, ṁ (kg m−2 h−1) is the evaporation rate, I (1.0 kW m−2) is the intensity of the normal direct solar irradiation, and hLV (J g−1) is the thermal enthalpy of transformation between the liquid and vapor phase and sensible heat.
The Co–CN–WA evaporator was placed in seawater, and 10 cycles of evaporation experiments were carried out under 1 sunlight generated by the solar simulator, to test the recycling performance of the evaporator. Each experiment lasted for 1 h, and the evaporation rate and efficiency during the evaporation process were recorded. In addition, the Co–CN–WA evaporator was immersed in seawater for a week, and evaporation experiments were carried out under 1 sunlight to test the stability of the evaporator in seawater. The evaporation rate and efficiency during the evaporation process were recorded.
Salt deposition resistance test
The Co–CN–WA evaporator was placed in natural seawater, 5 wt%, 10 wt% and 15 wt% NaCl solution, respectively, and simulated evaporation experiments were carried out under 1 sunlight generated by the solar simulator. The evaporation rate was recorded during the evaporation process. In addition, we placed the Co–CN–WA evaporator in seawater with 1.0 g of NaCl solid on the evaporator surface to test the automatic salt discharge performance. The changes of NaCl crystals on the surface and the evaporation rate during the evaporation process were recorded.
Experiments were typically conducted at an ambient temperature between 23 and 24 °C and humidity between 55% and 63%. Each group of experiments was tested in parallel for more than three times, and the average value was calculated.
Conclusions
A solar interface salt-tolerant evaporator was successfully prepared by loading the carbonized cobalt-based ZIF-L on the surface of wood aerogel using sodium alginate as a binder, which can realize continuous, stable and efficient solar seawater desalination. The wood aerogel as the supporting layer has excellent hydrophilicity, porous structure and low thermal conductivity, which is beneficial to high-efficiency water transport. Co–CN as the photothermal layer has excellent light absorption performance and photothermal conversion ability, and sodium alginate is used to strengthen the adhesion of the photothermal layer on the surface of the aerogel. The Co–CN–WA evaporator shows a high evaporation rate of 1.52 kg m−2 h−1 and a high evaporation efficiency of 92.42% under 1 sunlight irradiation. What's more, because of the strong diffusion and convection in the macroporous channels and pits, salt deposition is effectively prevented during the evaporation process and the stability is ensured. This evaporator derived from wood and a Co-based metal–organic framework is expected to play a role in solar clean water production, sewage purification and other fields.
Conflicts of interest
The authors declare no competing financial interest.
Acknowledgements
This work was supported by the Natural Science Foundation of Hainan Province (2019RC005), the National Natural Science Foundation of China (22061014), Hainan University start-up fund (KYQD(ZR)1806), and the Open Funds of the State Key Laboratory of Rare Earth Resource Utilization (RERU2021001 and RERU2021020).
Notes and references
- N. Xu, J. Li, Y. Wang, C. Fang, X. Li, Y. Wang, L. Zhou, B. Zhu, Z. Wu, S. Zhu and J. Zhu, A water lily–inspired hierarchical design for stableand efficient solar evaporation of high-salinity brine, Sci. Adv., 2019, 5, eaaw7013 CrossRef CAS.
- M. Rodell, J. S. Famiglietti, D. N. Wiese, J. T. Reager, H. K. Beaudoing, F. W. Landerer and M. H. Lo, Emerging trends in global freshwater availability, Nature, 2018, 557, 651–659 CrossRef CAS.
- S. Wu, H. Chen, H. Wang, X. Chen, H. Yang and S. B. Darling, Solar-driven evaporators for water treatment: challenges and opportunities, Environ. Sci.: Water Res. Technol., 2020, 7, 24–39 RSC.
- M. Elimelech and W. A. Phillip, The Future of Seawater Desalination: Energy, Technology, and the Environment, Science, 2011, 333, 712–717 CrossRef CAS.
- I. C. Karagiannis and P. G. Soldatos, Water desalination cost literature: review and assessment, Desalination, 2008, 223, 448–456 CrossRef CAS.
- Z. M. Binger and A. Achilli, Forward osmosis and pressure retarded osmosis process modeling for integration with seawater reverse osmosis desalination, Desalination, 2020, 491, 114583 CrossRef CAS.
- R. Verbeke, V. Gómez and I. F. J. Vankelecom, Chlorine-resistance of reverse osmosis (RO) polyamide membranes, Prog. Polym. Sci., 2017, 72, 1–15 CrossRef CAS.
- Z. Wang, Z. Wang, S. Lin, H. Jin, S. Gao, Y. Zhu and J. Jin, Nanoparticle-templated nanofiltration membranes for ultrahigh performance desalination, Nat. Commun., 2018, 9, 2004 CrossRef.
- Y. Han, Z. Xu and C. Gao, Ultrathin Graphene Nanofiltration Membrane for Water Purification, Adv. Funct. Mater., 2013, 23, 3693–3700 CrossRef CAS.
- A. A. Uliana, N. T. Bui, J. Kamcev, M. K. Taylor, J. J. Urban and J. R. Long, Ion-capture electrodialysis using multifunctional adsorptive membranes, Science, 2021, 372, 296–299 CrossRef CAS.
- M. A. Al-Obaidi, G. Filippini, F. Manenti and I. M. Mujtaba, Cost evaluation and optimisation of hybrid multi effect distillation and reverse osmosis system for seawater desalination, Desalination, 2019, 456, 136–149 CrossRef CAS.
- C. Xiao, W. Liang, L. Chen, J. He, F. Liu, H. Sun, Z. Zhu and A. Li, Janus Poly(ionic liquid) Monolithic Photothermal Materials with Superior Salt-Rejection for Efficient Solar Steam Generation, ACS Appl. Energy Mater., 2019, 2, 8862–8870 CrossRef CAS.
- V. D. Dao and H. S. Choi, Carbon-Based Sunlight Absorbers in Solar-Driven Steam Generation Devices, Glob. Chall., 2018, 2, 1700094 CrossRef PubMed.
- X. Zhou, F. Zhao, Y. Guo, B. Rosenberger and G. Yu, Architecting highly hydratable polymer networks to tune the water state for solar water purification, Sci. Adv., 2019, 5, eaaw5484 CrossRef CAS.
- F. Zhao, X. Zhou, Y. Shi, X. Qian, M. Alexander, X. Zhao, S. Mendez, R. Yang, L. Qu and G. Yu, Highly efficient solar vapour generation via hierarchically nanostructured gels, Nat. Nanotechnol., 2018, 13, 489–495 CrossRef CAS.
- X. Bai, Y. Li, F. Zhang, Y. Xu, S. Wang and G. Fu, Mass production of superhydrophilic sponges for efficient and stable solar-driven highly corrosive water evaporation, Environ. Sci.: Water Res. Technol., 2019, 5, 2041–2047 RSC.
- L. Zhu, M. Gao, C. K. N. Peh and G. W. Ho, Recent progress in solar-driven interfacial water evaporation: Advanced designs and applications, Nano Energy, 2019, 57, 507–518 CrossRef CAS.
- K. Xu, C. Wang, Z. Li, X. Yan, X. Mu, M. Ma and P. Zhang, Architecting a Janus biomass carbon/sponge evaporator with salt-rejection and ease of floatation for sustainable solar-driven desalination, Environ. Sci.: Water Res. Technol., 2021, 7, 879–885 RSC.
- H. Yin, H. Xie, J. Liu, X. Zou and J. Liu, Graphene tube shaped photothermal layer for efficient solar-driven interfacial evaporation, Desalination, 2021, 511, 115116 CrossRef CAS.
- M. Gao, C. K. Peh, H. T. Phan, L. Zhu and G. W. Ho, Solar Absorber Gel: Localized Macro-Nano Heat Channeling for Efficient Plasmonic Au Nanoflowers Photothermic Vaporization and Triboelectric Generation, Adv. Energy Mater., 2018, 8, 1800711 CrossRef.
- C. Xing, D. Huang, S. Chen, Q. Huang, C. Zhou, Z. Peng, J. Li, X. Zhu, Y. Liu, Z. Liu, H. Chen, J. Zhao, J. Li, L. Liu, F. Cheng, D. Fan and H. Zhang, Engineering Lateral Heterojunction of Selenium-Coated Tellurium Nanomaterials toward Highly Efficient Solar Desalination, Adv. Sci., 2019, 6, 1900531 CrossRef CAS PubMed.
- Y. Xia, Q. Hou, H. Jubaer, Y. Li, Y. Kang, S. Yuan, H. Liu, M. W. Woo, L. Zhang, L. Gao, H. Wang and X. Zhang, Spatially isolating salt crystallisation from water evaporation for continuous solar steam generation and salt harvesting, Energy Environ. Sci., 2019, 12, 1840–1847 RSC.
- D. You, W. Yang, Y. Zhao, H. Yu, Y. Ma, D. Lin, Q. Pan and S. Song, Salt-tolerant and low-cost flame-treated aerogel for continuously efficient solar steam generation, Sol. Energy, 2021, 227, 303–311 CrossRef CAS.
- D. Zhang, M. Zhang, S. Chen, Q. Liang, N. Sheng, Z. Han, Y. Cai and H. Wang, Scalable, self-cleaning and self-floating bi-layered bacterial cellulose biofoam for efficient solar evaporator with photocatalytic purification, Desalination, 2021, 500, 114899 CrossRef CAS.
- L. Zhao, J. Tian, Y. Liu, L. Xu, Y. Wang, X. Fei and Y. Li, A novel floatable composite hydrogel for solar evaporation enhancement, Environ. Sci.: Water Res. Technol., 2020, 6, 221–230 RSC.
- X. Han, L. V. Besteiro, C. S. L. Koh, H. K. Lee, I. Y. Phang, G. C. Phan-Quang, J. Y. Ng, H. Y. F. Sim, C. L. Lay, A. Govorov and X. Y. Ling, Intensifying Heat Using MOF-Isolated Graphene for Solar-Driven Seawater Desalination at 98% Solar-to-Thermal Efficiency, Adv. Funct. Mater., 2021, 31, 2008904 CrossRef CAS.
- Y. Liu, Z. Liu, Q. Huang, X. Liang, X. Zhou, H. Fu, Q. Wu, J. Zhang and W. Xie, A high-absorption and self-driven salt-resistant black gold nanoparticle-deposited sponge for highly efficient, salt-free, and long-term durable solar desalination, J. Mater. Chem. A, 2019, 7, 2581–2588 RSC.
- M. Zhu, Y. Li, F. Chen, X. Zhu, J. Dai, Y. Li, Z. Yang, X. Yan, J. Song, Y. Wang, E. Hitz, W. Luo, M. Lu, B. Yang and L. Hu, Plasmonic Wood for High-Efficiency Solar Steam Generation, Adv. Energy Mater., 2018, 8, 1701028 CrossRef.
- T. Li, H. Liu, X. Zhao, G. Chen, J. Dai, G. Pastel, C. Jia, C. Chen, E. Hitz, D. Siddhartha, R. Yang and L. Hu, Scalable and Highly Efficient Mesoporous Wood-Based Solar Steam Generation Device: Localized Heat, Rapid Water Transport, Adv. Funct. Mater., 2018, 28, 1707134 CrossRef.
- J. Zhou, Z. Sun, M. Chen, J. Wang, W. Qiao, D. Long and L. Ling, Macroscopic and Mechanically Robust Hollow Carbon Spheres with Superior Oil Adsorption and Light-to-Heat Evaporation Properties, Adv. Funct. Mater., 2016, 26, 5368–5375 CrossRef CAS.
- G. Zhu, J. Xu, W. Zhao and F. Huang, Constructing Black Titania with Unique Nanocage Structure for Solar Desalination, ACS Appl. Mater. Interfaces, 2016, 8, 31716–31721 CrossRef CAS.
- J. Wang, Y. Li, L. Deng, N. Wei, Y. Weng, S. Dong, D. Qi, J. Qiu, X. Chen and T. Wu, High-Performance Photothermal Conversion of Narrow-Bandgap Ti2O3 Nanoparticles, Adv. Mater., 2017, 29, 1603730 CrossRef PubMed.
- N. Ma, Q. Fu, Y. Hong, X. Hao, X. Wang, J. Ju and J. Sun, Processing Natural Wood into an Efficient and Durable Solar Steam Generation Device, ACS Appl. Mater. Interfaces, 2020, 12, 18165–18173 CrossRef CAS PubMed.
- X. Fan, Y. Yang, X. Shi, Y. Liu, H. Li, J. Liang and Y. Chen, A MXene-Based Hierarchical Design Enabling Highly Efficient and Stable Solar-Water Desalination with Good Salt Resistance, Adv. Funct. Mater., 2020, 30, 2007110 CrossRef CAS.
- M. U. Farid, J. A. Kharraz and A. K. An, Plasmonic Titanium Nitride Nano-enabled Membranes with High Structural Stability for Efficient Photothermal Desalination, ACS Appl. Mater. Interfaces, 2021, 13, 3805–3815 CrossRef CAS PubMed.
- L. Song, X.-F. Zhang, Z. Wang, T. Zheng and J. Yao, Fe3O4/polyvinyl alcohol decorated delignified wood evaporator for continuous solar steam generation, Desalination, 2021, 507, 115024 CrossRef CAS.
- X. Song, H. Song, N. Xu, H. Yang, L. Zhou, L. Yu, J. Zhu, J. Xu and K. Chen, Omnidirectional and effective salt-rejecting absorber with rationally designed nanoarchitecture for efficient and durable solar vapour generation, J. Mater. Chem. A, 2018, 6, 22976–22986 RSC.
- S. He, C. Chen, Y. Kuang, R. Mi, Y. Liu, Y. Pei, W. Kong, W. Gan, H. Xie, E. Hitz, C. Jia, X. Chen, A. Gong, J. Liao, J. Li, Z. J. Ren, B. Yang, S. Das and L. Hu, Nature-inspired salt resistant bimodal porous solar evaporator for efficient and stable water desalination, Energy Environ. Sci., 2019, 12, 1558–1567 RSC.
- X. Chen, X. Zhu, S. He, L. Hu and Z. J. Ren, Advanced Nanowood Materials for the Water–Energy Nexus, Adv. Mater., 2021, 33, 2001240 CrossRef CAS.
- C. Chen, Y. Wang, Q. Wu, Z. Wan, D. Li and Y. Jin, Highly strong and flexible composite hydrogel reinforced by aligned wood cellulose skeleton via alkali treatment for muscle-like sensors, Chem. Eng. J., 2020, 400, 125876 CrossRef CAS.
- Q. Zhang, L. Li, B. Jiang, H. Zhang, N. He, S. Yang, D. Tang and Y. Song, Flexible and Mildew-Resistant Wood-Derived Aerogel for Stable and Efficient Solar Desalination, ACS Appl. Mater. Interfaces, 2020, 12, 28179–28187 CrossRef CAS.
- K. Tu, B. Puertolas, M. Adobes-Vidal, Y. Wang, J. Sun, J. Traber, I. Burgert, J. Perez-Ramirez and T. Keplinger, Green Synthesis of Hierarchical Metal–Organic Framework/Wood Functional Composites with Superior Mechanical Properties, Adv. Sci., 2020, 7, 1902897 CrossRef CAS.
- R. Wang, J. Yang, X. Chen, Y. Zhao, W. Zhao, G. Qian, S. Li, Y. Xiao, H. Chen, Y. Ye, G. Zhou and F. Pan, Highly Dispersed Cobalt Clusters in Nitrogen-Doped Porous Carbon Enable Multiple Effects for High-Performance Li–S Battery, Adv. Energy Mater., 2020, 10, 1903550 CrossRef CAS.
- D. Fang, Y. Wang, C. Qian, X. Liu, X. Wang, S. Chen and S. Zhang, Synergistic Regulation of Polysulfides Conversion and Deposition by MOF-Derived Hierarchically Ordered Carbonaceous Composite for High-Energy Lithium–Sulfur Batteries, Adv. Funct. Mater., 2019, 29, 1900875 CrossRef.
- Q. Wang, R. Zou, W. Xia, J. Ma, B. Qiu, A. Mahmood, R. Zhao, Y. Yang, D. Xia and Q. Xu, Facile Synthesis of Ultrasmall CoS2 Nanoparticles within Thin N-Doped Porous Carbon Shell for High Performance Lithium-Ion Batteries, Small, 2015, 11, 2511–2517 CrossRef CAS PubMed.
- Q. Zhang, X. Zhao, X. Miao, W. Yang, C. Wang and Q. Pan, ZIF-L-Co@carbon fiber paper composite derived Co/Co3O4@C electrocatalyst for ORR in alkali/acidic media and overall seawater splitting, Int. J. Hydrogen Energy, 2020, 45, 33028–33036 CrossRef CAS.
- Z. Wang, Y. Yan, X. Shen, C. Jin, Q. Sun and H. Li, A wood–polypyrrole composite as a photothermal conversion device for solar evaporation enhancement, J. Mater. Chem. A, 2019, 7, 20706–20712 RSC.
- M. Kim, K. Yang, Y. S. Kim, J. C. Won, P. Kang, Y. H. Kim and B. G. Kim, Laser-induced photothermal generation of flexible and salt-resistant monolithic bilayer membranes for efficient solar desalination, Carbon, 2020, 164, 349–356 CrossRef CAS.
- H. Li, H. Wen, J. Li, J. Huang, D. Wang and B. Z. Tang, Doping AIE Photothermal Molecule into All-Fiber Aerogel with Self-Pumping Water Function for Efficiency Solar Steam Generation, ACS Appl. Mater. Interfaces, 2020, 12, 26033–26040 CrossRef CAS.
- F. Wang, Y. Su, Y. Li, D. Wei, H. Sun, Z. Zhu, W. Liang and A. Li, Salt-Resistant Photothermal Materials Based on Monolithic Porous Ionic Polymers for Efficient Solar Steam Generation, ACS Appl. Energy Mater., 2020, 3, 8746–8754 CrossRef CAS.
- W. Chao, X. Sun, Y. Li, G. Cao, R. Wang, C. Wang and S.-H. Ho, Enhanced Directional Seawater Desalination Using a Structure-Guided Wood Aerogel, ACS Appl. Mater. Interfaces, 2020, 12, 22387–22397 CrossRef CAS PubMed.
- B. Shao, Y. Wang, X. Wu, Y. Lu, X. Yang, G. Y. Chen, G. Owens and H. Xu, Stackable nickel–cobalt@polydopamine nanosheet based photothermal sponges for highly efficient solar steam generation, J. Mater. Chem. A, 2020, 8, 11665–11673 RSC.
- Q. Jiang, L. Tian, K. K. Liu, S. Tadepalli, R. Raliya, P. Biswas, R. R. Naik and S. Singamaneni, Bilayered Biofoam for Highly Efficient Solar Steam Generation, Adv. Mater., 2016, 28, 9400–9407 CrossRef CAS PubMed.
Footnote |
† Electronic supplementary information (ESI) available. See DOI: 10.1039/d1ew00593f |
|
This journal is © The Royal Society of Chemistry 2022 |