Plasmonic Bi NP-accelerated interfacial charge transfer for enhanced solar-driven ciprofloxacin mineralization†
Received
27th September 2021
, Accepted 29th November 2021
First published on 2nd December 2021
Abstract
Highly efficient mineralization of refractory organic pollutants is an admittedly difficult problem for most photocatalytic processes. Herein, a hierarchical type-II heterojunction Bi2MoO6/Bi/BiOBr-x (BMO/Bi/BOB-x) was successfully fabricated via a one-pot solvothermal method. The optimal BMO/Bi/BOB-2 exhibited an ∼80% mineralization ratio of ciprofloxacin under simulated solar irradiation, outperforming most reported photocatalysts. ˙OH was demonstrated to be the dominant reactive species. The ˙OH production capacity of BMO/Bi/BOB-2 increased by 76.2% compared with that of BMO/BOB, highlighting the key role of Bi nanoparticles (Bi NPs). Bi NPs not only induced a strong localized surface plasmon resonance effect to enhance the utilization of visible light, but also acted as an electron mediator to accelerate the separation of e−/h+ pairs. Due to the specific redox potential, the separated h+ and e− could activate H2O and O2 to produce ˙OH synchronously. Overall, this work provided a valuable strategy to design a plasmonic Bi NP-enhanced type-II heterojunction for the efficient treatment of refractory organic wastewater.
Environmental significance
Highly efficient mineralization of refractory organic pollutants is quite challenging for most photocatalytic processes. In this work, a Bi NP-bridged type-II heterojunction Bi2MoO6/BiOBr was synthesized successfully via a one-pot solvothermal method. By regulating the solvothermal temperature, Bi NPs could be introduced in the type-II heterojunction Bi2MoO6/BiOBr. Because of the specific band structures, the Bi NPs could greatly accelerate the interfacial charge transfer. Consequently, the photocatalytic performance was vastly enhanced. Besides, the strong LSPR effect of Bi NPs could tremendously enhance the absorption of visible light. In summary, our work provided a new strategy for the design of a plasmonic Bi NP-enhanced type-II heterojunction for wastewater treatment.
|
1. Introduction
The ever-increasing production, consumption and discharge of antibiotics have attracted widespread attention on account of their potential harm to the ecosystem and human health.1 However, traditional activated sludge treatments exhibit rather low removal efficiency for antibiotics limited by the strong antibacterial activity.2 Therefore, it is imperative to develop efficient and eco-friendly approaches to remove aqueous antibiotics. In recent decades, advanced oxidation processes (AOPs) including chlorination, photocatalysis, ozonation, peroxymonosulfate activation, Fenton and permanganate oxidation have been reported to be efficient in antibiotic degradation.3–8 However, the selectivity of certain reactive radicals (such as ˙SO4−) in AOPs restrains the efficient mineralization of refractory organic pollutants. As a comparison, the non-selective ˙OH is promising to achieve the efficient mineralization by converting organic pollutants into H2O, CO2 and mineral acids.9 Nevertheless, traditional Fenton and Fenton-like processes were usually limited by the low pH requirement and high iron sludge production.10,11 Therefore, it is highly desired to develop a novel and green process to produce ˙OH for efficient mineralization of refractory organic contaminants.
In recent years, photocatalysis has been extensively applied in water purification, due to its mild reaction conditions and low energy cost.12 Apart from the photo-generated holes (h+), reactive oxygen species (ROS), such as ˙OH, ˙O2− and O2, also play important roles in the degradation of organic pollutants.13 As the most important ROS during the mineralization process, ˙OH has been demonstrated to be produced through two main pathways.14 As shown in eqn (1)–(4), h+ could oxidize H2O to produce ˙OH directly and e− could activate O2 to produce ˙OH indirectly. Both processes are determined by the redox ability of the valence band (EVB) and conduction band (ECB). Therefore, designing photocatalysts with suitable band structures is key to efficient ˙OH production.
| Photocatalyst → h+ + e− (hv > Eg) | (1) |
| h+ + H2O → ˙OH + H+E = 2.38 − 0.059 pH | (2) |
| 2e− + 2H+ + O2 → H2O2E = 0.68 − 0.059 pH | (3) |
Due to their low toxicity, high stability and suitable band structures, Bi-based photocatalysts, such as BiOBr, Bi2O2CO3, Bi2MoO6, and BiPO4, have received extensive attention in the removal of refractory organic pollutants.15–18 In particular, BiOBr (BOB) and Bi2MoO6 (BMO) exhibit excellent photocatalytic activities, due to their unique layered structure.19 However, their wide band gap and high photo-generated carrier recombination rate jointly limit the high utilization of visible light.
Generally, constructing heterojunctions and decorating plasmonic noble metals on the surface of semiconductors are effective strategies to promote e−/h+ pair separation and enhance visible light absorption.20–22 By feat of the built-in electric field resulting from band alignment at the heterojunction interface between two semiconductors, the separation efficiency of h+/e− pairs could be greatly improved. It has been reported that many BMO- and BOB-based heterojunctions exhibited excellent photocatalytic efficiency compared with pristine BMO or BOB.23–27
However, decorating plasmonic noble metals on the surface of semiconductors mainly makes use of the local surface plasmon resonance (LSPR) effect of noble metals to widen the visible light absorption range.28 To date, various photocatalysts decorated with plasmonic noble metals have been developed.29–32 However, the high cost of noble metals limited their practical applications. Therefore, the LSPR effect of non-noble metals (Cu, Bi) has gradually attracted much attention.33–35
Recently, a series of plasmonic Bi nanoparticle (Bi NP) decorated semiconductors, such as Bi/TiO2, Bi/Bi2WO6, Bi/g-C3N4, Bi/RGO, Bi/Bi4NO8Cl and Bi/BiOCl, were fabricated with high photocatalytic performance.36–42 Therefore, decoration of plasmonic Bi NPs was proved to be an effective approach to enhance photocatalytic activities. Although both constructing heterojunctions and decorating Bi NPs could enhance the photocatalytic performance, constructing photocatalysts combining both strategies is rare and challenging.
In this work, a novel ternary hierarchical BMO/Bi/BOB-x heterojunction was prepared via a simple solvothermal method. The degradation and mineralization ratios of ciprofloxacin (CIP) were used to evaluate the photocatalytic activity. It showed that the introduction of Bi NPs into BMO/BOB composites could vastly promote the photocatalytic activity towards ciprofloxacin degradation. The role of Bi NPs in the photocatalytic process was explored and the relevant CIP decomposition mechanism was proposed.
2. Experimental section
2.1. Materials
All reagents were used as received without any further purification. Sodium bromide (NaBr), bismuth nitrate pentahydrate (Bi(NO3)3·5H2O), sodium molybdate (Na2MoO4), potassium dichromate(K2Cr2O7), ethylene glycol (EG), and ethyl alcohol (EtOH) were purchased from Sinopharm Chemical Reagent Co., Ltd. (Shanghai, China). Ciprofloxacin (CIP), sulfamethoxazole (SMZ), p-benzoquinone (BQ), ammonium oxalate (AO), tertiary butyl alcohol (TBA), benzoic acid (BA), p-hydroxybenzoic acid (p-HBA), sodium dihydrogen phosphate (NaH2PO4), disodium hydrogen phosphate (Na2HPO4), peroxidase (POD), N-diethyl-1,4-phenylene-diamine sulfate (DPD) and sodium hydroxide (NaOH) were obtained from Aladdin Chemical Co., Ltd. An ultrapure water resistivity of above 18.2 MΩ was used to prepare solutions.
2.2. Synthesis of photocatalysts
Ternary heterojunction BMO/Bi/BOB-x (x represents the different molar ratios of Na2MoO6 and NaBr) was prepared via a one-pot solvothermal method. Generally, 4 mmol Bi(NO3)3·5H2O was dissolved in 40 mL ethylene glycol, named solution A. Certain quantities of NaBr and Na2MoO6 were dissolved in 10 mL ultrapure water, named solution B, otherwise adjusting the solvothermal temperature to 200 °C. The schematic diagram of the synthesis procedures of BMO/Bi/BOB-x is shown in Scheme 1. All the synthesis details of other photocatalysts can be found in Table S1.†
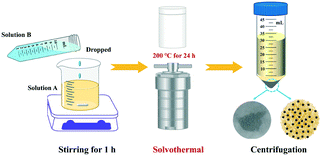 |
| Scheme 1 Schematic diagram of the synthesis procedures of BMO/Bi/BOB-x. | |
2.3. Characterization
BMO/Bi/BOB-2 was selected as the representative BMO/Bi/BOB-x because of its highest photocatalytic performance. X-ray diffraction (XRD) patterns were obtained on an X'TRA X-ray diffractometer (XRD, Switzerland) with Cu Kα1 radiation. A high-resolution transmission electron microscope (HRTEM, JEOL-2100F) working with an accelerating voltage of 200 kV was used for morphology measurements. Scanning electron microscopy (SEM) and elemental analysis were performed on a scanning electron microscope (Hitachi S-4800) and an energy dispersive X-ray spectrometer (EDX). The specific surface area of the materials was measured by the Brunauer–Emmett–Teller method (BET, 3H-2000PS1, China). A thermo spectrometer (ESCALAB 250, UK) with Al Kα as the X-ray source was applied to perform X-ray photoelectron spectroscopy (XPS). UV-vis diffuse reflectance spectra (DRS) were obtained on a UV-vis spectrophotometer (SHIMADZU, Japan) with BaSO4 as the reflectance standard. Photoluminescence (PL) spectra were recorded on a fluorescence spectrophotometer (F-4500, HITACHI, Japan) with an excitation wavelength of 325 nm. Time-resolved photoluminescence (TRPL) measurements were performed on a fluorescence spectrofluorometer (FLS980, Germany). The total organic carbon (TOC) was analyzed using a Shimadzu total organic analyzer. Electrochemical impedance spectra (EIS) and photocurrent plots were obtained on a CHI660E electrochemical workstation. The electrolyte was a 0.1 M Na2SO4 aqueous solution. A Pt plate and saturated calomel electrode were used as the counter electrode and reference electrode, respectively. Electron spin resonance (ESR) measurements were conducted on a Bruker ER200-SRC spectrometer to determine the reactive species in the photocatalytic process.
2.4. Photocatalytic CIP degradation experiment
The photocatalytic performance of the as-prepared photocatalysts was evaluated by the degradation of CIP. Firstly, 20 mg photocatalysts were added into 50 mL CIP aqueous solutions (20 mg L−1) in a quartz glass tube and stirred for 60 min in the dark to achieve the adsorption–desorption equilibrium. And then, a 350 W xenon lamp (Xujiang Electromechanical Plant, China) was selected as the simulated solar light source with an optical power density of ∼180 mW cm−2. The temperature was kept at room temperature by recirculating water. Subsequently, 1.5 mL solution was extracted at given time intervals and filtered with a polytetrafluoroethylene membrane (0.22 μm). The concentration of CIP was determined using a high-performance liquid chromatography instrument (HPLC, Shimadzu, LC2010, Japan) with a symmetric C18 column (5 μm, 4.6 × 250 mm). The mobile phase was 20% acetonitrile and 80% H2O with 0.1% (v/v) formic acid solution at a flow rate of 1.0 mL min−1. The detection wavelength for CIP was set to 279 nm. The intermediate products of CIP degradation were identified using a liquid chromatograph mass spectrometer (LC-MS, Agilent 1290/6460, Triple Quad MS, USA). Besides, the CIP degradation was fitted with a pseudo-first-order kinetic model and the rate constant (k1) was determined by eqn (5). |  | (5) |
where Ct refers to the CIP concentration at a certain time, and C0 refers to the concentration of CIP when the photoreaction began (t = 0). The stability and recyclability of BMO/Bi/BOB-2 were tested by recycling experiments.
Apart from CIP, SMZ, BPA, TC and phenol were also used to assess the photocatalytic performance of BMO/Bi/BOB. The analysis parameters of these pollutants can be seen in Table S2.†
2.5. Free radical quenching experiment
TBA, BQ, AO and K2Cr2O7 were the quenching agents of ˙OH, ˙O2−, h+ and e−, respectively, and other conditions were the same as the photocatalytic CIP degradation experiment. N2 was used to exclude the role of O2.
2.6. Quantitative analysis of ˙OH and H2O2
Quantitative analysis of ˙OH was performed as previously reported.43 Generally, ˙OH reacted with BA and formed p-HBA. The concentration of p-HBA in solution was determined by HPLC. The mobile phase was 40% acetonitrile and 60% 6 mmol L−1 phosphoric acid (v/v) at a flow rate of 1.0 mL min−1. The detection wavelength for p-HBA was set to 256 nm.
Quantitative analysis of H2O2 was performed by the DPD method.44 In brief, 2 mL of sample solution was mixed with 0.4 mL of phosphate buffer. Then, 0.05 mL of DPD and 0.05 mL of POD were added into the mixed solution and stirred for 1 min. The absorbance of the solution was measured at 551 nm using a UV/visible spectrophotometer. The stock solution of DPD was prepared by dissolving 0.05 g DPD in 5 mL of 0.05 M H2SO4. The stock solution of POD was prepared by dissolving 0.005 g POD in 5 mL of ultrapure water. The phosphate buffer solution was prepared by adding 87.7 mL of NaH2PO4 (1 M) and 12.6 mL of Na2HPO4 (1 M) into 99.7 mL water.
3. Results and discussion
3.1. Phase structure and chemical composition
The phase structures of the as-prepared photocatalysts were determined by XRD. All the results are shown in Fig. 1. All the emerging XRD peaks of the as-prepared photocatalysts well agreed with the crystal planes of tetragonal BOB (JCPDS card No. 09-0393), orthorhombic BMO (JCPDS card No. 77-1246) and hexagonal Bi (JCPDS card No. 44-1246). As for pristine BMO and BOB, the experimental results well conformed with the XRD peaks of orthorhombic BMO and tetragonal BOB, which indicated the successful preparation. As for binary Bi/BMO and Bi/BOB, the typical XRD peaks of Bi appeared, which showed the formation of Bi NPs when the solvothermal temperature increased up to 200 °C. The production of Bi NPs could be explained by eqn (6) and (7). Taking advantage of the weak reducibility of ethylene glycol, Bi3+ can be reduced to Bi0 at a suitable temperature.45 Therefore, Bi NPs were randomly dispersed on the surface of BMO/BOB through the in situ reduction method.46 Different from electrostatic interaction, this in situ reduction method could introduce strong covalent interaction in BMO/Bi/BOB. The strong binding force exhibited high transfer kinetics of photogenerated charges.47 | HOCH2CH2OH → CH3CHO + H2O (T > 160 °C) | (6) |
| 2Bi3+ + 6CH3CHO → 3CH3CO – OCCH3 + 2Bi0 + 6H+ | (7) |
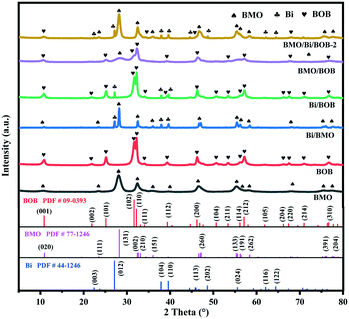 |
| Fig. 1 XRD patterns of the as-prepared photocatalysts. | |
As for binary BMO/BOB, the typical XRD peaks of BOB and BMO could be found obviously, which showed that the BMO/BOB heterojunction was obtained via a one-pot solvothermal method. As for the ternary BMO/Bi/BOB-2 heterojunction, all the typical XRD peaks of BMO, BOB and Bi could be found, which meant the successful preparation.
The elemental composition, the surface chemical state, and the interaction between BMO and BOB in BMO/Bi/BOB-2 were further proved by XPS. As outlined in Fig. S2,† Mo, Br, Bi, and O were clearly detected in the full survey spectrum of BMO/Bi/BOB-2.
As shown in Fig. 2a, the two strong peaks at 233.06 and 236.17 eV for pristine BMO were well consistent with Mo6+ 3d5/2 and Mo6+ 3d3/2.48–50 However, the relevant XPS peaks at 232.84 and 235.97 eV for BMO/BOB were 0.22 and 0.20 eV lower than those for pristine BMO, indicating the increase of electron density around Mo atoms. A wide negative shift of the corresponding binding energies was found for BMO/Bi/BOB-2, implying the further increase of electron density around Mo atoms. The results indicated that the introduction of Bi NPs could further promote the electron transfer between BMO and BOB. Notably, some Mo6+ was also reduced to Mo5+ in BMO/Bi/BOB-2 due to the reducibility of EG.
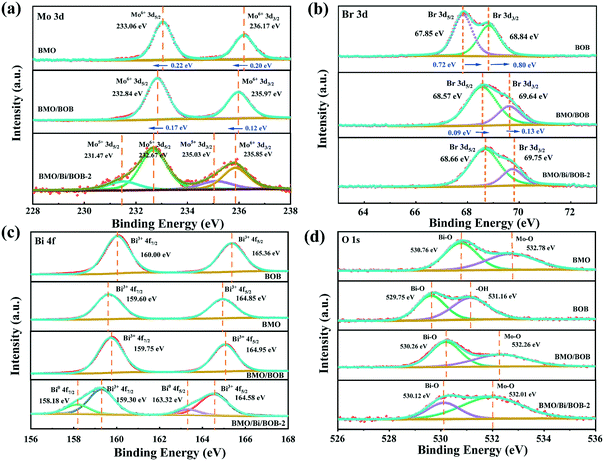 |
| Fig. 2 XPS spectra of (a) Mo 3d, (b) Br 3d, (c) Bi 4f and (d) O 1s of the as-prepared photocatalysts. | |
Adverse results were found in the high-resolution spectra of Br 3d in Fig. 2b. The two strong peaks at 67.85 and 68.84 eV for pure BOB were consistent with Br 3d5/2 and Br 3d3/2.51–53 However, the corresponding XPS peaks for the BMO/BOB composite were at 68.57 and 69.64 eV, exhibiting a positive shift of binding energies (0.72 eV and 0.80 eV) higher than those for pristine BOB, which indicated the decrease of electron density around Br atoms. It is worth noting that the corresponding XPS peaks for BMO/Bi/BOB-2 were at 68.66 and 69.75 eV, being 0.09 and 0.11 eV higher than those for BMO/BOB. The further increase of binding energy indicated the more intimate interaction in BMO/Bi/BOB-2.54
As shown in Fig. 2c, the peaks at 160.00 and 165.36 eV for pristine BOB, along with the peaks at 159.60 and 164.85 eV for pristine BMO were in good agreement with Bi3+ 4f7/2 and Bi3+ 4f5/2, respectively, confirming the existence of Bi3+.55,56 The corresponding XPS peaks for BMO/BOB shifted to 159.75 and 164.95 eV, being between pristine BOB and BMO. However, the corresponding XPS peaks shifted to 159.30 and 164.58 eV for BMO/Bi/BOB-2, which were lower than those for BMO, BOB and BMO/BOB, indicating a strong interaction after inducing Bi NPs. Notably, the obvious XPS peaks (158.18 and 163.32 eV) of Bi0 were also found for BMO/Bi/BOB-2, confirming the production of Bi NPs, which were in good accordance with XRD.57,58 According to the area ratio of the XPS peaks of Bi3+ 4f and Bi0 4f, the optimal load of Bi NPs was estimated to be 10.75%.
Fig. 2d shows the O 1s spectra of BMO, BOB, BMO/BOB and BMO/Bi/BOB-2. The O 1s spectrum of pristine BMO in could be divided into two peaks at 530.76 and 532.78 eV corresponding to Bi–O and Mo–O.59 And the O 1s spectrum of pristine BOB could be divided into two peaks at 529.75 and 531.16 eV. The XPS peaks at 530.26 and 532.26 eV for BMO/BOB were between pristine BMO and BOB, indicating the intimate contact of BMO and BOB. Compared with BMO/BOB, a little negative shift of O 1s in BMO/Bi/BOB-2 was found, which meant that Bi NPs could accelerate the electron transfer process of BMO/BOB.
3.2. Morphology and microstructure of the as-prepared photocatalysts
The typical SEM images of the as-prepared photocatalysts are shown in Fig. 3. As shown in Fig. 3a and b, BOB had a microsphere structure with a diameter of about 2 μm. The surface of the Bi/BOB microspheres became rough, which was attributed to the production of Bi on the surface of BOB. As shown in Fig. 3c and d, BMO and Bi/BMO exhibited obviously hierarchical microsphere structures with a diameter of about 1–2 μm. Both BMO/BOB and BMO/Bi/BOB-2 also exhibited obviously hierarchical microsphere structures, being similar to BMO and Bi/BMO. Differently, the diameters of BMO/BOB and BMO/Bi/BOB-2 were only 0.5–0.7 μm, probably resulting from the cogrowth of BMO and BOB.
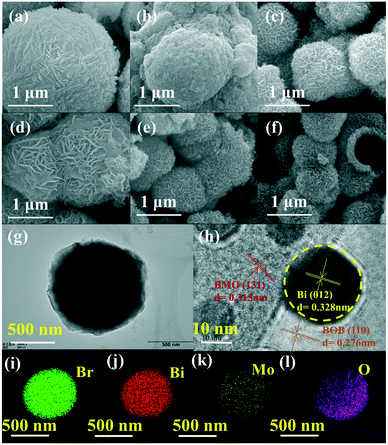 |
| Fig. 3 SEM images of (a) BOB, (b) Bi/BOB, (c) BMO, (d) Bi/BMO, (e) BMO/BOB and (f) BMO/Bi/BOB-2; (g) TEM image and (h) HRTEM image of BMO/Bi/BOB-2; element mappings of (i) Br, (j) Bi, (k) Mo and (l) O of BMO/Bi/BOB-2. | |
The surface morphological details and interface microstructural information were investigated by TEM to confirm the formation of BMO/Bi/BOB-2. The low-resolution TEM image of BMO/Bi/BOB-2 is presented in Fig. 3g. Obviously, BMO/Bi/BOB-2 exhibited a typical microsphere structure, which was also consistent with the results of SEM. The HRTEM image of BMO/Bi/BOB-2 is shown in Fig. 3h. The lattice spacing fringes of small crystallites were investigated to be 0.315, 0.276 and 0.328 nm, corresponding to the (131), (110) and (012) crystallographic planes of orthorhombic BMO, BOB and Bi NPs, respectively.60–62 These findings also confirmed the existence of BMO, BOB and Bi NPs in BMO/Bi/BOB-2, which was also consistent with XRD. Such an intimate contact between BMO, BOB and Bi indicated the formation of the BMO/Bi/BOB-2 heterojunction, which was beneficial to interfacial charge separation during the photocatalytic process. Besides, the EDS mappings of Br, Bi, O and Mo in Fig. 3i–l showed that all four elements were uniformly distributed on the surface of BMO/Bi/BOB-2, confirming the successful preparation.
3.3. Optical properties and energy band structures of the as-prepared photocatalysts
The optical properties of the as-prepared photocatalysts were analyzed by measuring the ultraviolet-visible (UV-vis) diffuse reflectance spectra. As shown in Fig. 4a, BOB and BMO exhibited poor absorption of visible light (λ > 480 nm). However, by the introduction of Bi NPs, both Bi/BMO and Bi/BOB showed obvious absorption of visible light, even near-infrared light. In addition, the ternary BMO/Bi/BOB-2 heterojunction showed the strongest light absorbance for ultraviolet, visible light and near-infrared light. In order to explore the energy-band structure of BMO/Bi/BOB-2, the corresponding bandgap, valence band and conduction band values were further determined. The energy band gap (Eg) of BMO and BOB could be estimated by plotting Tauc's equation.63,64 As shown in Fig. 4b, the Eg of BMO and BOB was estimated to be 2.75 and 2.97 eV, respectively. Moreover, the valence band XPS spectra of BMO and BOB were recorded to measure the valence band maximum (VBM) values. The VB of the two components was obtained by the linear extrapolation method. As seen from Fig. 4c, the VBM values of BMO and BOB were determined to be 2.71 and 2.52 eV, respectively. Thus, the corresponding conduction band minimum (CBM) values could be calculated to be −0.04 and −0.45 eV based on the bandgap values and valence band positions, just as seen in Fig. 4d.
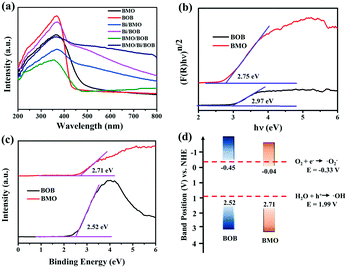 |
| Fig. 4 (a) UV-vis DRS absorption spectra of the as-prepared photocatalysts; (b) plots of (αhν)n/2versus hν of BMO and BOB; (c) valence band spectra and (d) energy band structures of BOB and BMO. | |
3.4. Photocatalytic capacity of BMO/Bi/BOB-2 for CIP degradation
The photocatalytic degradation of CIP (the pH was adjusted to 5.0) with 0.4 g L−1 photocatalysts according to preliminary experiments was carried out under simulated sunlight irradiation. As shown in Fig. 5a and S3,† Bi, BMO and BOB exhibited poor degradation efficiencies towards CIP, and the k values were 0.0058, 0.0179 and 0.0412 min−1, respectively. Compared with pristine Bi, BMO and BOB, Bi/BMO and Bi/BOB exhibited much higher degradation efficiencies, with k values of up to 0.0239 and 0.0477 min−1, respectively. The enhanced photocatalytic activity by introducing Bi NPs could be attributed to the LSPR effect. Besides, BMO/BOB also exhibited much higher CIP degradation efficiency than BMO and BOB. And the k value (0.0576 min−1) of BMO/BOB was 2.2 and 1.4 times higher than those of BMO and BOB, respectively. The enhanced photocatalytic activity by BMO/BOB could be attributed to the efficient separation of photogenerated e−/h+ pairs and the longer lifetime of photo-induced e−/h+ pairs at the interface of the BMO/BOB heterojunction.
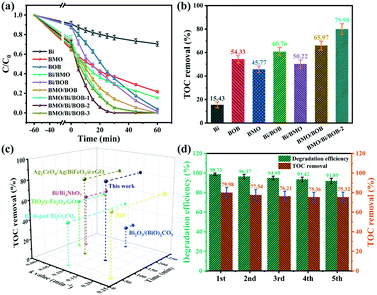 |
| Fig. 5 (a) Photocatalytic CIP degradation by different photocatalysts; (b) the TOC removal of CIP by different photocatalysts; (c) comparison of CIP degradation over other reported photocatalysts;65–70 (d) the repeated photocatalytic experiments of the BMO/Bi/BOB-2 photocatalyst for degradation of CIP. Conditions: [CIP] = 20 mg L−1; [photocatalysts] = 0.40 g L−1; light source: 350 W xenon lamp. | |
Note particularly that the ternary heterojunction BMO/Bi/BOB-2 exhibited the highest CIP degradation efficiency, with the largest k value (0.1502 min−1), which was 25.9, 8.4, 3.6 and 1.6 times higher than those of Bi, BMO, BOB and BMO/BOB, respectively. Consequently, the introduction of Bi NPs could obviously enhance the photocatalytic CIP degradation.
The mineralization ratio of CIP was also determined based on TOC, which can be seen in Fig. 5b. Obviously, BMO/Bi/BOB-2 exhibited the highest TOC removal ratio of 79.98% after 2 h irradiation. As shown in Fig. 5c and Table S7,† compared with other reported photocatalysts, BMO/Bi/BOB-2 showed obvious advantages in both kinetics and mineralization ability.
The reusability and stability of photocatalysts are important factors for the feasibility of their practical applications and cost effectiveness. The reusability and stability of BMO/Bi/BOB-2 were tested in the degradation and mineralization of CIP for 5 consecutive cycles (Fig. 5d). The degradation efficiency of CIP by BMO/Bi/BOB-2 still remained more than 90% after 5 cycles in 30 min, and the corresponding mineralization ratio remained more than 75% in 120 min. As shown in Table S6,† after five successive cycles, the recovery rate of the catalysts remained more than 93%, exhibiting excellent stability. Meanwhile, the leaching of Bi in solution was less than 0.100 mg L−1 after five successive cycles as well, which also indicated the excellent stability. The structural stability of BMO/Bi/BOB-2 after 5 cycles was investigated by XRD in Fig. S6.† There were no obvious changes between the fresh and the recycled one, which indicated the good structure stability of BMO/Bi/BOB-2 in the photocatalytic process. Other environmental factors affecting the photocatalytic efficiency, such as the solution pH, coexisting humic acid and salt ions are discussed in Fig. S8.†
Apart from CIP, other refractory organic pollutants including SMZ, phenol, TC and BPA were also used to assess the photocatalytic activity of BMO/Bi/BOB-2, as shown in Fig. S7.† All of these organic pollutants could be completely degraded in 45 min, and the TOC removal ratios were more than 80% after 120 min of xenon lamp irradiation.
3.5. Mechanism exploration
3.5.1. Reactive species and degradation pathway of CIP.
In order to explore ROS in the degradation of CIP, radical quenching experiments and ESR were performed. As shown in Fig. 6a, there was no obvious degradation of CIP with the addition of IPA, indicating that ˙OH plays an important role. With the addition of AO or by purging N2, the degradation process of CIP was also partly restrained, suggesting that h+ and O2 also took part in the degradation process of CIP. However, with the addition of BQ and Cr(VI), the degradation process of CIP was not restrained obviously, implying that ˙O2− and e− were not the main reactive species.
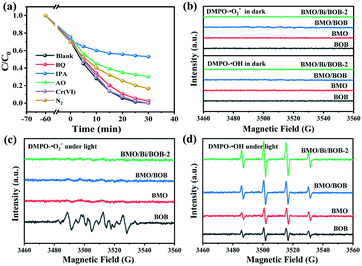 |
| Fig. 6 (a) The photocatalytic degradation of CIP using BMO/Bi/BOB-2 with different scavengers; (b) ESR spectra of DMPO–˙O2− and DMPO–˙OH in the dark; ESR spectra of (c) DMPO–˙O2− and (d) DMPO–˙OH under 30 min irradiation by the 350 W Xenon lamp. | |
To further verify these hypotheses, ESR was also performed. As shown in Fig. 6b, there were no ESR signals for BMO, BOB, BMO/BOB and BMO/Bi/BOB-2 in the dark. In Fig. 6c, an obvious ESR signal of DMPO–˙O2− for BOB was found under xenon lamp irradiation. But for BMO, BMO/BOB and BMO/Bi/BOB-2, there were yet no ESR signals of DMPO–˙O2− appeared under xenon lamp irradiation. These results indicated that only BOB could activate the O2 to produce ˙O2−. Nevertheless, as for DMPO–˙OH in Fig. 6d, there were obvious ESR signals of DMPO–˙OH for BMO, BOB, BMO/BOB and BMO/Bi/BOB-2 under irradiation. Note particularly that the intensity of the ESR signals of ˙OH followed the order BMO/Bi/BOB-2 > BMO/BOB > BMO > BOB, which strongly conformed to the order of photocatalytic performance. The photocatalytic ˙OH production capacities of BMO, BOB, BMO/BOB and BMO/Bi/BOB-2 were also determined. As shown in Fig. S9,† BMO/Bi/BOB-2 exhibited the highest photocatalytic ˙OH and H2O2 production capacities, being much higher than those of BMO, BOB and BMO/BOB. The photocatalytic ˙OH and H2O2 production capacities of BMO/Bi/BOB-2 were greatly enhanced, which indicated that the Bi NPs could vastly boost the separation of e−/h+ pairs.
The production of ˙OH could be explained by the redox ability of the conduction band and valence band. The redox potentials of H2O/˙OH, O2/˙O2− and O2/H2O2 at pH = 5 were 2.09, −0.33 and 0.40 eV, respectively. As for BMO, the ECB and EVB were 2.71 and −0.04 eV, respectively. Therefore, h+ could oxidize H2O to ˙OH, but e− could only activate O2 to H2O2. As for BOB, the ECB and EVB were 2.52 and −0.45 eV, respectively. Therefore, h+ could oxidize H2O to ˙OH and e− could activate O2 to ˙O2− simultaneously. And these results matched well with ESR.
As shown in Fig. S11,† there were two possible photocatalytic mechanisms in BMO/BOB (Z-scheme and type-II). If BMO/BOB is a Z-scheme heterojunction, higher concentrations of ˙OH and ˙O2− would be measured concurrently. However, more ˙OH and no ˙O2− were found in BMO/BOB compared with BMO and BOB, indicating that BMO/BOB was not a Z-scheme heterojunction but a type-II heterojunction. Notably, BMO/Bi/BOB-2 exhibited the highest ˙OH production but no ˙O2− production, indicating that Bi NPs might not change the type-II charge transfer mechanism and enhanced this process in turn. Therefore, BMO/Bi/BOB-2 exhibited the highest separation efficiency of e−/h+ pairs. The photoelectric characterization (ZIS, PC, PL and TRPL) also supported this viewpoint.
The EIS spectra in Fig. 7a showed the Nyquist plots of BMO, BOB, BMO/BOB and BMO/Bi/BOB-2. Compared with BMO and BOB, BMO/BOB exhibited a smaller arc-radius suggesting its lower charge transfer resistance. Furthermore, BMO/Bi/BOB-2 exhibited a smaller arc radius than BMO/BOB, implying that the introduction of Bi NPs could further decrease the charge transfer resistance. Fig. 7b clearly shows that the photocurrent density of all the samples dropped dramatically with the light being turned off, while the photocurrent density of the four photocatalysts increased at different levels under simulated solar light irradiation. The photocurrent intensity among the various samples followed the order BMO/Bi/BOB-2 > BMO/BOB > BOB > BMO, suggesting that BMO/Bi/BOB-2 exhibited excellent separation ability of e−/h+ pairs, which was in accordance with the results of EIS.
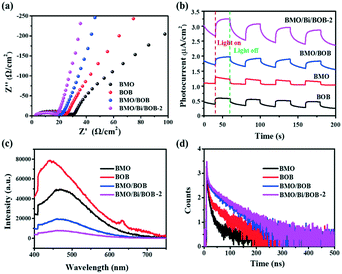 |
| Fig. 7 (a) EIS, (b) photocurrent, (c) PL and (d) TRPL spectra of BMO, BOB, BMO/BOB and BMO/Bi/BOB-2. | |
PL and TRPL were also used to elucidate the separation efficiency and dynamics of photogenerated carriers. As shown in Fig. 7c, BMO/BOB exhibited lower PL intensity than BMO and BOB, indicating the higher separation efficiency of e−/h+ pairs. Moreover, compared with BMO/BOB, the lower PL intensity of BMO/Bi/BOB-2 indicated that the introduction of Bi NPs could further increase the separation efficiency of e−/h+ pairs. TRPL was used to estimate the average lifetime of e−/h+ pairs (the detailed calculation method can be seen in the ESI†). Generally, a longer fluorescence means a higher separation efficiency of e−/h+ pairs. As shown in Fig. 7d and Table S7,† the average lifetime of BMO, BOB, BMO/BOB and BMO/Bi/BOB-2 was 43.62, 53.69, 72.18 and 85.51 ns, respectively, indicating that BMO/Bi/BOB-2 exhibited the highest separation efficiency of e−/h+ pairs. All these photoelectrochemical characterization results were in favor that the introduction of Bi NPs could further increase the separation efficiency of e−/h+ pairs.
To better understand the degradation pathway of CIP with BMO/Bi/BOB-2, the possible intermediates were identified by HPLC-MS at different degradation times (15 min and 30 min). As shown in Fig. S12a† (15 min), the ion peak at m/z = 332.2 was the nondegraded CIP in solution. Meanwhile, the other ion peaks at m/z = 362, 334, 306, 290, 262 and 126 were the degradation intermediates. However, the possible intermediates in Fig. S12b† (30 min) were only identified at the ion peaks at m/z = 362, 290, 262 and 126, and the relative contents were increased by varying degrees. The structural information of CIP and their possible intermediates is summarized in Table S8.† Combining the LC/MS results and previous reports, the degradation of CIP mainly occurred via three routes, ring-opening, removal of functional groups and oxidation reactions.71 The proposed degradation pathways of CIP are given in Fig. 8. Firstly, the piperazine ring in CIP (m/z = 332) was destroyed by ˙OH and formed I1 (m/z = 362). Subsequently, I1 continued to be oxidized to remove a carbonyl group and formed I2 and I2′ (m/z = 334). Then the carbonyl groups in I2 and I2′ were further removed to form I3 (m/z = 306). Afterwards, the amino functional group in I3 was removed and the adjacent C was oxidized to O, forming I4 (m/z = 263). And then, the carboxyl group and cyclopropyl group were destroyed to form I6 (m/z = 126). Finally, I6 was further oxidized to CO2, H2O and small molecule acid.
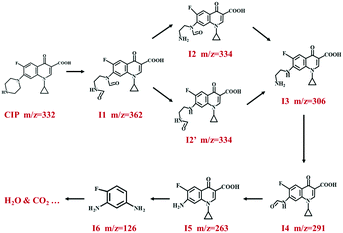 |
| Fig. 8 Photocatalytic degradation pathway of CIP by BMO/Bi/BOB-2. | |
3.5.2. Photocatalytic mechanism of BMO/Bi/BOB-2.
Based on the radical quenching experiments and ESR, BMO/BOB was not a Z-scheme heterojunction but a type-II heterojunction. The built-in electric field at the interface of BMO and BOB could further facilitate the separation process of e−/h+ pairs. The ECB edge of BOB was more negatively charged than that of BMO while the EVB edge of BMO was more positively charged than that of BOB. Hence, when BMO/BOB was exposed to the irradiation of simulated solar light, the photogenerated e− transferred from the ECB of BOB to the ECB of BMO, while the photogenerated h+ transferred from the EVB of BMO to the EVB of BOB. Consequently, h+ accumulated on the surface of BOB while e− accumulated on the surface of BMO. Finally, the accumulated e− may react with O2 to produce H2O2 (resolved into ˙OH finally) and the accumulated h+ could oxidize H2O to yield ˙OH. The ˙OH of dual-path production was subsequently involved in the degradation and mineralization of CIP.
Based on the above discussion, the enhanced photocatalytic mechanism of BMO/Bi/BOB-2 is shown in Fig. 9. As the Fermi energy (Ef = −0.17 eV) of Bi NPs was more positively charged than the ECB of BMO but more negatively charged than the ECB of BMO, Bi NPs could act as an electron mediator to speed up the transfer process of photogenerated e−/h+ pairs. In other words, the photogenerated e− transferred from the ECB of BOB to Bi NPs firstly, and then transferred to the EVB of BOB immediately. Because of the high carrier mobility rate, BMO/Bi/BOB-2 exhibited higher separation efficiency of photogenerated carriers, which could further produce more ˙OH and H2O2. The obtained H2O2 could be further divided into ˙OH by ultraviolet light. Aside from the electron mediator, the hot electron injection effect arising from the LSPR effect of Bi NPs was also an important reason for the enhanced mechanism.72,73 Therefore, compared with BMO/BOB, the introduction of Bi NPs could greatly intensify the dual-path ˙OH production process, resulting in a higher photocatalytic activity.
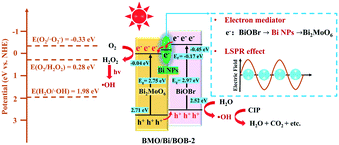 |
| Fig. 9 Schematic diagram for photocatalytic degradation of CIP by BMO/Bi/BOB-2. | |
4. Conclusions
In summary, a novel hierarchical plasmonic BMO/Bi/BOB-x heterojunction was fabricated via a simple one-pot solvothermal method. As for optimal BMO/Bi/BOB-2, the constant k value and the mineralization ratio of CIP reached 0.502 min−1 and 79.98%, respectively, which were 160.7% and 21.1% higher than those of BMO/BOB. The introduction of Bi NPs greatly boosted the photocatalytic process. Bi NPs not only accelerated the separation of photoinduced e−/h+ pairs, but also broadened the absorption region. ˙OH was proved to be the predominant reactive species, which was produced via H2O oxidation and O2 reduction processes simultaneously. The ˙OH production capacity of BMO/Bi/BOB-2 was 76.2% higher than that of BMO/BOB, which resulted in much higher photocatalytic degradation and mineralization ability towards CIP. Moreover, BMO/Bi/BOB-2 exhibited more than 80% mineralization ratios towards extensive organic pollutants under simulated solar irradiation. The pH and coexisting anions performed little effects on the degradation process, which presented the good environmental suitability of BMO/Bi/BOB-2. Besides, BMO/Bi/BOB-2 was reusable in 5 cycles without significant efficiency decay, indicating its good stability during the photocatalytic process. To sum up, this work supported a novel idea for the design and preparation of a plasmonic Bi NP-enhanced ternary heterojunction for the efficient treatment of refractory organic wastewater.
Author contributions
Cailiang Yue: experimental design, data curation, writing – original draft preparation. Changqing Zhu: conceptualization, supervision, writing – review & editing. Wenting Zheng: writing – review & editing, data processing. Jinli Qiu: data processing. Zhiling Du: data processing. Chen Ling: data processing. Fu-Qiang Liu: experimental design, conceptualization, supervision, writing – review & editing.
Conflicts of interest
The authors declare no conflict of interest.
Acknowledgements
This work was supported by the National Outstanding Youth Science Fund Project of National Natural Science Foundation of China (No. 51522805).
References
- C. Hobson, A. N. Chan and G. D. Wright, The Antibiotic Resistome: A Guide for the Discovery of Natural Products as Antimicrobial Agents, Chem. Rev., 2021, 121, 3464–3494 CrossRef CAS PubMed.
- A. Zarei-Baygi, M. Harb, P. Wang, L. B. Stadler and A. L. Smith, Evaluating Antibiotic Resistance Gene Correlations with Antibiotic Exposure Conditions in Anaerobic Membrane Bioreactors, Environ. Sci. Technol., 2019, 53, 3599–3609 CrossRef CAS PubMed.
- R. Yang, H. Li, Y. Wang, Z. Zhu, C. Hu, T. Zhao, W. Wang and B. Liu, A novel flower-like Z-type heterojunction CuS/Bi7O9I3 composite catalyst prepared under mild conditions for degradation of antibiotics and sterilization under visible light, Environ. Sci.: Nano, 2020, 7, 3074–3087 RSC.
- S. Wang, Y. Liu and J. Wang, Peroxymonosulfate Activation by Fe-Co-O-Codoped Graphite Carbon Nitride for Degradation of Sulfamethoxazole, Environ. Sci. Technol., 2020, 54, 10361–10369 CrossRef CAS PubMed.
- J. Wang, X. Quan, S. Chen, H. Yu and Y. Chen, Performing homogeneous catalytic ozonation using heterogeneous Mn2+-bonded oxidized carbon nanotubes by self-driven pH variation induced reversible desorption and adsorption of Mn2+, Environ. Sci.: Nano, 2019, 6, 1932–1940 RSC.
- D. Jia, Y. Zhang, X. Zhang, P. Feng, L. Yang, R. Ning, H. Pan and Y. Miao, Facile fabrication of Bi nanoparticle-decorated g-C3N4 photocatalysts for effective tetracycline hydrochloride degradation: environmental factors, degradation mechanism, pathways and biotoxicity evaluation, Environ. Sci.: Nano, 2021, 8, 415–431 RSC.
- J. Hu, C. Chen, T. Hu, J. Li, H. Lu, Y. Zheng, X. Yang, C. Guo and C. M. Li, Metal-free heterojunction of black phosphorus/oxygen-enriched porous g-C3N4 as an efficient photocatalyst for Fenton-like cascade water purification, J. Mater. Chem. A, 2020, 8, 19484–19492 RSC.
- Y. Zhou, Y. Gao, J. Jiang, Y. M. Shen, S. Y. Pang, Y. Song and Q. Guo, A comparison study of levofloxacin degradation by peroxymonosulfate and permanganate: Kinetics, products and effect of quinone group, J. Hazard. Mater., 2021, 403, 123834 CrossRef CAS PubMed.
- S. Y. Liou and M. C. Dodd, Evaluation of hydroxyl radical and reactive chlorine species generation from the superoxide/hypochlorous acid reaction as the basis for a novel advanced oxidation process, Water Res., 2021, 200, 117142 CrossRef CAS PubMed.
- M. Guo, Z. Xing, T. Zhao, Y. Qiu, B. Tao, Z. Li and W. Zhou, Hollow flower-like polyhedral α-Fe2O3/Defective MoS2/Ag Z-scheme heterojunctions with enhanced photocatalytic-Fenton performance via surface plasmon resonance and photothermal effects, Appl. Catal., B, 2020, 272, 117142 CrossRef.
- A. Serra-Clusellas, L. De Angelis, C. H. Lin, P. Vo, M. Bayati, L. Sumner, Z. Lei, N. B. Amaral, L. M. Bertini, J. Mazza, L. R. Pizzio, J. D. Stripeikis, J. A. Rengifo-Herrera and M. M. Fidalgo de Cortalezzi, Abatement of 2,4-D by H2O2 solar photolysis and solar photo-Fenton-like process with minute Fe(III) concentrations, Water Res., 2018, 144, 572–580 CrossRef CAS PubMed.
- C. Zhu, T. Wei, Y. Wei, L. Wang, M. Lu, Y. Yuan, L. Yin and L. Huang, Unravelling intramolecular charge transfer in donor–acceptor structured g-C3N4 for superior photocatalytic hydrogen evolution, J. Mater. Chem. A, 2021, 9, 1207–1212 RSC.
- Y. Nosaka and A. Y. Nosaka, Generation and Detection of Reactive Oxygen Species in Photocatalysis, Chem. Rev., 2017, 117, 11302–11336 CrossRef CAS PubMed.
- R. Song, H. Chi, Q. Ma, D. Li, X. Wang, W. Gao, H. Wang, X. Wang, Z. Li and C. Li, Highly Efficient Degradation of Persistent Pollutants with 3D Nanocone TiO2-Based Photoelectrocatalysis, J. Am. Chem. Soc., 2021, 143, 13664–13674 CrossRef CAS PubMed.
- J. Xu, W. Meng, Y. Zhang, L. Li and C. Guo, Photocatalytic degradation of tetrabromobisphenol A by mesoporous BiOBr: Efficacy, products and pathway, Appl. Catal., B, 2011, 107, 355–362 CrossRef CAS.
- Z. Wang, H. Wang, Z. Zeng, G. Zeng, P. Xu, R. Xiao, D. Huang, X. Chen, L. He, C. Zhou, Y. Yang, Z. Wang, W. Wang and W. Xiong, Metal-organic frameworks derived Bi2O2CO3/porous carbon nitride: A nanosized Z-scheme systems with enhanced photocatalytic activity, Appl. Catal., B, 2020, 267, 118700 CrossRef CAS.
- L. Zhang, T. Xu, X. Zhao and Y. Zhu, Controllable synthesis of Bi2MoO6 and effect of morphology and variation in local structure on photocatalytic activities, Appl. Catal., B, 2010, 98, 138–146 CrossRef CAS.
- S. P. Sahu, M. Qanbarzadeh, M. Ateia, H. Torkzadeh, A. S. Maroli and E. L. Cates, Rapid Degradation and Mineralization of Perfluorooctanoic Acid by a New Petitjeanite Bi3O(OH)(PO4)2 Microparticle Ultraviolet Photocatalyst, Environ. Sci. Technol. Lett., 2018, 5, 533–538 CrossRef CAS.
- S. Chen, D. Huang, M. Cheng, L. Lei, Y. Chen, C. Zhou, R. Deng and B. Li, Surface and interface engineering of two-dimensional bismuth-based photocatalysts for ambient molecule activation, J. Mater. Chem. A, 2021, 9, 196–233 RSC.
- Z. Xing, J. Hu, M. Ma, H. Lin, Y. An, Z. Liu, Y. Zhang, J. Li and S. Yang, From One to Two: In Situ Construction of an Ultrathin 2D-2D Closely Bonded Heterojunction from a Single-Phase Monolayer Nanosheet, J. Am. Chem. Soc., 2019, 141, 19715–19727 CrossRef CAS PubMed.
- X. Wu, J. N. Hart, X. Wen, L. Wang, Y. Du, S. X. Dou, Y. H. Ng, R. Amal and J. Scott, Improving the Photo-Oxidative Performance of Bi2MoO6 by Harnessing the Synergy between Spatial Charge Separation and Rational Co-Catalyst Deposition, ACS Appl. Mater. Interfaces, 2018, 10, 9342–9352 CrossRef CAS PubMed.
- H. Zhang, T. Itoi, T. Konishi and Y. Izumi, Dual Photocatalytic Roles of Light: Charge Separation at the Band Gap and Heat via Localized Surface Plasmon Resonance To Convert CO2 into CO over Silver-Zirconium Oxide, J. Am. Chem. Soc., 2019, 141, 6292–6301 CrossRef CAS PubMed.
- T. Jia, J. Wu, J. Song, Q. Liu, J. Wang, Y. Qi, P. He, X. Qi, L. Yang and P. Zhao, In situ self-growing 3D hierarchical BiOBr/BiOIO3 Z-scheme heterojunction with rich oxygen vacancies and iodine ions as carriers transfer dual-channels for enhanced photocatalytic activity, Chem. Eng. J., 2020, 396, 125258 CrossRef CAS.
- X. Wu, K. Zhang, G. Zhang and S. Yin, Facile preparation of BiOX (X = Cl, Br, I) nanoparticles and up-conversion phosphors/BiOBr composites for efficient degradation of NO gas: Oxygen vacancy effect and near infrared light responsive mechanism, Chem. Eng. J., 2017, 325, 59–70 CrossRef CAS.
- X. Li, J. Xiong, X. Gao, J. Ma, Z. Chen, B. Kang, J. Liu, H. Li, Z. Feng and J. Huang, Novel BP/BiOBr S-scheme nano-heterojunction for enhanced visible-light photocatalytic tetracycline removal and oxygen evolution activity, J. Hazard. Mater., 2020, 387, 121690 CrossRef CAS PubMed.
- J. Tian, P. Hao, N. Wei, H. Cui and H. Liu, 3D Bi2MoO6 Nanosheet/TiO2 Nanobelt Heterostructure: Enhanced Photocatalytic Activities and Photoelectochemistry Performance, ACS Catal., 2015, 5, 4530–4536 CrossRef CAS.
- H. Li, T. Hu, R. Zhang, J. Liu and W. Hou, Preparation of solid-state Z-scheme Bi2MoO6/MO (M = Cu, Co3/4, or Ni) heterojunctions with internal electric field-improved performance in photocatalysis, Appl. Catal., B, 2016, 188, 313–323 CrossRef CAS.
- Z. Zhang, J. Huang, Y. Fang, M. Zhang, K. Liu and B. Dong, A Nonmetal Plasmonic Z-Scheme Photocatalyst with UV- to NIR-Driven Photocatalytic Protons Reduction, Adv. Mater., 2017, 29, 1606688 CrossRef PubMed.
- K. Wang, J. Li and G. Zhang, Ag-Bridged Z-Scheme 2D/2D Bi5FeTi3O15/g-C3N4 Heterojunction for Enhanced Photocatalysis: Mediator-Induced Interfacial Charge Transfer and Mechanism Insights, ACS Appl. Mater. Interfaces, 2019, 11, 27686–27696 CrossRef CAS PubMed.
- Y. Ren, C. Li, Q. Xu, J. Yan, Y. Li, P. Yuan, H. Xia, C. Niu, X. Yang and Y. Jia, Two-dimensional amorphous heterostructures of Ag/a-WO3− for high-efficiency photocatalytic performance, Appl. Catal., B, 2019, 245, 648–655 CrossRef CAS.
- J. E. Lee, S. Bera, Y. S. Choi and W. I. Lee, Size-dependent plasmonic effects of M and M@SiO2 (M = Au or Ag) deposited on TiO2 in photocatalytic oxidation reactions, Appl. Catal., B, 2017, 214, 15–22 CrossRef CAS.
- X. Li, H. Jiang, C. Ma, Z. Zhu, X. Song, H. Wang, P. Huo and X. Li, Local surface plasma resonance effect enhanced Z-scheme ZnO/Au/g-C3N4 film photocatalyst for reduction of CO2 to CO, Appl. Catal., B, 2021, 283, 119638 CrossRef CAS.
- C. Cheng, W. H. Fang, R. Long and O. V. Prezhdo, Water Splitting with a Single-Atom Cu/TiO2 Photocatalyst: Atomistic Origin of High Efficiency and Proposed Enhancement by Spin Selection, JACS Au, 2021, 1, 550–559 CrossRef CAS PubMed.
- Y. Pang, W. Zang, Z. Kou, L. Zhang, G. Xu, J. Lv, X. Gao, Z. Pan, J. Wang and Y. Wu, Assembling of Bi atoms on TiO2 nanorods boosts photoelectrochemical water splitting of semiconductors, Nanoscale, 2020, 12, 4302–4308 RSC.
- S. Luo, J. Xu, Z. Li, C. Liu, J. Chen, X. Min, M. Fang and Z. Huang, Bismuth oxyiodide coupled with bismuth nanodots for enhanced photocatalytic bisphenol A degradation: synergistic effects and mechanistic insight, Nanoscale, 2017, 9, 15484–15493 RSC.
- W. Fan, C. Li, H. Bai, Y. Zhao, B. Luo, Y. Li, Y. Ge, W. Shi and H. Li, An in situ photoelectroreduction approach to fabricate Bi/BiOCl heterostructure photocathodes: understanding the role of Bi metal for solar water splitting, J. Mater. Chem. A, 2017, 5, 4894–4903 RSC.
- Z. Zhao, W. Zhang, X. Lv, Y. Sun, F. Dong and Y. Zhang, Noble metal-free Bi nanoparticles supported on TiO2 with plasmon-enhanced visible light photocatalytic air purification, Environ. Sci.: Nano, 2016, 3, 1306–1317 RSC.
- J. Wang, H. Liang, C. Zhang, B. Jin and Y. Men, Bi2WO6-x nanosheets with tunable Bi quantum dots and oxygen vacancies for photocatalytic selective oxidation of alcohols, Appl. Catal., B, 2019, 256, 117874 CrossRef CAS.
- F. Dong, Z. Zhao, Y. Sun, Y. Zhang, S. Yan and Z. Wu, An Advanced Semimetal-Organic Bi Spheres-g-C3N4 Nanohybrid with SPR-Enhanced Visible-Light Photocatalytic Performance for NO Purification, Environ. Sci. Technol., 2015, 49, 12432–12440 CrossRef CAS PubMed.
- Z. Wang, S. Yan, Y. Sun, T. Xiong, F. Dong and W. Zhang, Bi metal sphere/graphene oxide nanohybrids with enhanced direct plasmonic photocatalysis, Appl. Catal., B, 2017, 214, 148–157 CrossRef CAS.
- X. Wu, Y. Zhang, K. Wang, S. Zhang, X. Qu, L. Shi and F. Du, In-situ construction of Bi/defective Bi4NbO8Cl for non-noble metal based Mott-Schottky photocatalysts towards organic pollutants removal, J. Hazard. Mater., 2020, 393, 122408 CrossRef CAS PubMed.
- J. Xu, F. Xu, M. Qian, F. Xu, Z. Hong and F. Huang, Conductive Carbon Nitride for Excellent Energy Storage, Adv. Mater., 2017, 29, 1701674 CrossRef PubMed.
- Y. Ren, M. Shi, W. Zhang, D. D. Dionysiou, J. Lu, C. Shan, Y. Zhang, L. Lv and B. Pan, Enhancing the Fenton-like Catalytic Activity of nFe2O3 by MIL-53(Cu) Support: A Mechanistic Investigation, Environ. Sci. Technol., 2020, 54, 5258–5267 CrossRef CAS PubMed.
- S. Wu, H. Yu, S. Chen and X. Quan, Enhanced Photocatalytic H2O2 Production over Carbon Nitride by Doping and Defect Engineering, ACS Catal., 2020, 10, 14380–14389 CrossRef CAS.
- Y. Wang and K. S. Kim, Large-scale polyol synthesis of single-crystal bismuth nanowires and the role of NaOH in the synthesis process, Nanotechnology, 2008, 19, 265303 CrossRef PubMed.
- H. Yu, L. Jiang, H. Wang, B. Huang, X. Yuan, J. Huang, J. Zhang and G. Zeng, Modulation of Bi2MoO6-Based Materials for Photocatalytic Water Splitting and Environmental Application: a Critical Review, Small, 2019, 15, 1901008 CrossRef PubMed.
- H. Wang, X. Yuan, Y. Wu, G. Zeng, W. Tu, C. Sheng, Y. Deng, F. Chen and J. W. Chew, Plasmonic Bi nanoparticles and BiOCl sheets as cocatalyst deposited on perovskite-type ZnSn(OH)6 microparticle with facet-oriented polyhedron for improved visible-light-driven photocatalysis, Appl. Catal., B, 2017, 209, 543–553 CrossRef CAS.
- H. Wang, S. Chen, Z. Wang, Y. Zhou and Z. Wu, A novel hybrid Bi2MoO6-MnO2 catalysts with the superior plasma induced pseudo photocatalytic-catalytic performance for ethyl acetate degradation, Appl. Catal., B, 2019, 254, 339–350 CrossRef CAS.
- G. Zhang, D. Chen, N. Li, Q. Xu, H. Li, J. He and J. Lu, Fabrication of Bi2MoO6/ZnO hierarchical heterostructures with enhanced visible-light photocatalytic activity, Appl. Catal., B, 2019, 250, 313–324 CrossRef CAS.
- Y. Chen, G. Tian, Y. Shi, Y. Xiao and H. Fu, Hierarchical MoS2/Bi2MoO6 composites with synergistic effect for enhanced visible photocatalytic activity, Appl. Catal., B, 2015, 164, 40–47 CrossRef CAS.
- H. Yu, J. Huang, L. Jiang, Y. Shi, K. Yi, W. Zhang, J. Zhang, H. Chen and X. Yuan, Enhanced photocatalytic tetracycline degradation using N-CQDs/OV-BiOBr composites: Unraveling the complementary effects between N-CQDs and oxygen vacancy, Chem. Eng. J., 2020, 402, 126187 CrossRef CAS.
- Z. Shi, Y. Zhang, X. Shen, G. Duoerkun, B. Zhu, L. Zhang, M. Li and Z. Chen, Fabrication of g-C3N4/BiOBr heterojunctions on carbon fibers as weaveable photocatalyst for degrading tetracycline hydrochloride under visible light, Chem. Eng. J., 2020, 386, 124010 CrossRef CAS.
- M. Li, Q. Lu, M. Liu, P. Yin, C. Wu, H. Li, Y. Zhang and S. Yao, Photoinduced Charge Separation via the Double-Electron Transfer Mechanism in Nitrogen Vacancies g-C3N5/BiOBr for the Photoelectrochemical Nitrogen Reduction, ACS Appl. Mater. Interfaces, 2020, 12, 38266–38274 CrossRef CAS PubMed.
- G. Zhang, D. Chen, N. Li, Q. Xu, H. Li, J. He and J. Lu, Construction of Hierarchical Hollow Co9 S8/ZnIn2 S4 Tubular Heterostructures for Highly Efficient Solar Energy Conversion and Environmental Remediation, Angew. Chem., Int. Ed., 2020, 59, 8255–8261 CrossRef CAS PubMed.
- Y. Wu, M. Song, Z. Chai, J. Huang and X. Wang, Facile Construction of Bi2MoO6/Bi/g-C3N4 toward Efficient Photocatalytic Oxidation of Indoor Gaseous Formaldehyde with a Wide Concentration Range under Visible Light Irradiation, ACS Sustainable Chem. Eng., 2020, 8, 7710–7720 CrossRef CAS.
- X. Xu, X. Ding, X. Yang, P. Wang, S. Li, Z. Lu and H. Chen, Oxygen vacancy boosted photocatalytic decomposition of ciprofloxacin over Bi2MoO6: Oxygen vacancy engineering, biotoxicity evaluation and mechanism study, J. Hazard. Mater., 2019, 364, 691–699 CrossRef CAS PubMed.
- Y. Yang, H. Chen, X. Zou, X. L. Shi, W. D. Liu, L. Feng, G. Suo, X. Hou, X. Ye, L. Zhang, C. Sun, H. Li, C. Wang and Z. G. Chen, Flexible Carbon-Fiber/Semimetal Bi Nanosheet Arrays as Separable and Recyclable Plasmonic Photocatalysts and Photoelectrocatalysts, ACS Appl. Mater. Interfaces, 2020, 12, 24845–24854 CrossRef CAS PubMed.
- N. Li, H. Gao, X. Wang, S. Zhao, D. Lv, G. Yang, X. Gao, H. Fan, Y. Gao and L. Ge, Novel indirect Z-scheme g-C3N4/Bi2MoO6/Bi hollow microsphere heterojunctions with SPR-promoted visible absorption and highly enhanced photocatalytic performance, Chin. J. Catal., 2020, 41, 426–434 CrossRef CAS.
- S. Wang, X. Ding, N. Yang, G. Zhan, X. Zhang, G. Dong, L. Zhang and H. Chen, Insight into the effect of bromine on facet-dependent surface oxygen vacancies construction and stabilization of Bi2MoO6 for efficient photocatalytic NO removal, Appl. Catal., B, 2020, 265, 118585 CrossRef CAS.
- H. Li, J. Liu, W. Hou, N. Du, R. Zhang and X. Tao, Synthesis and characterization of g-C3N4/Bi2MoO6 heterojunctions with enhanced visible light photocatalytic activity, Appl. Catal., B, 2014, 160-161, 89–97 CrossRef CAS.
- X. Shi, P. Wang, W. Li, Y. Bai, H. Xie, Y. Zhou and L. Ye, Change in photocatalytic NO removal mechanisms of ultrathin BiOBr/BiOI via NO3− adsorption, Appl. Catal., B, 2019, 243, 322–329 CrossRef CAS.
- D. Chen, J. Fang, S. Lu, G. Zhou, W. Feng, F. Yang, Y. Chen and Z. Fang, Fabrication of Bi modified Bi2S3 pillared g-C3N4 photocatalyst and its efficient photocatalytic reduction and oxidation performances, Appl. Surf. Sci., 2017, 426, 427–436 CrossRef CAS.
- J. Di, C. Chen, C. Zhu, M. Ji, J. Xia, C. Yan, W. Hao, S. Li, H. Li and Z. Liu, Bismuth vacancy mediated single unit cell Bi2WO6 nanosheets for boosting photocatalytic oxygen evolution, Appl. Catal., B, 2018, 238, 119–125 CrossRef CAS.
- Y. Ao, K. Wang, P. Wang, C. Wang and J. Hou, Synthesis of novel 2D-2D p-n heterojunction BiOBr/La2Ti2O7 composite photocatalyst with enhanced photocatalytic performance under both UV and visible light irradiation, Appl. Catal., B, 2016, 194, 157–168 CrossRef CAS.
- A. Kumar, G. Sharma, M. Naushad, T. Ahamad, R. C. Veses and F. J. Stadler, Highly visible active Ag2CrO4/Ag/BiFeO3@RGO nano-junction for photoreduction of CO2 and photocatalytic removal of ciprofloxacin and bromate ions: The triggering effect of Ag and RGO, Chem. Eng. J., 2019, 370, 148–165 CrossRef CAS.
- K. Wang, Y. Li, G. Zhang, J. Li and X. Wu, 0D Bi nanodots/2D Bi3NbO7 nanosheets heterojunctions for efficient visible light photocatalytic degradation of antibiotics: Enhanced molecular oxygen activation and mechanism insight, Appl. Catal., B, 2019, 240, 39–49 CrossRef CAS.
- M. Chen, J. Yao, Y. Huang, H. Gong and W. Chu, Enhanced photocatalytic degradation of ciprofloxacin over Bi2O3/(BiO)2CO3 heterojunctions: Efficiency, kinetics, pathways, mechanisms and toxicity evaluation, Chem. Eng. J., 2018, 334, 453–461 CrossRef CAS.
- F. Wang, X. Yu, M. Ge and S. Wu, One-step synthesis of TiO2/γ-Fe2O3/GO nanocomposites for visible light-driven degradation of ciprofloxacin, Chem. Eng. J., 2020, 384, 123381 CrossRef CAS.
- S. Li, T. Huang, P. Du, W. Liu and J. Hu, Photocatalytic transformation fate and toxicity of ciprofloxacin related to dissociation species: Experimental and theoretical evidences, Water Res., 2020, 185, 116286 CrossRef CAS PubMed.
- X. Hu, H. Zhao, Y. Liang and R. Chen, Energy level mediation of (BiO)2CO3 via Br doping for efficient molecular oxygen activation and ciprofloxacin photodegradation, Appl. Catal., B, 2019, 258, 117966 CrossRef CAS.
- X. Hu, X. Hu, Q. Peng, L. Zhou, X. Tan, L. Jiang, C. Tang, H. Wang, S. Liu, Y. Wang and Z. Ning, Mechanisms underlying the photocatalytic degradation pathway of ciprofloxacin with heterogeneous TiO2, Chem. Eng. J., 2020, 380, 122366 CrossRef CAS.
- T. Tatsuma, H. Nishi and T. Ishida, Plasmon-induced charge separation: chemistry and wide applications, Chem. Sci., 2017, 8, 3325–3337 RSC.
- S. Kim, J. M. Kim, J. E. Park and J. M. Nam, Nonnoble-Metal-Based Plasmonic Nanomaterials: Recent Advances and Future Perspectives, Adv. Mater., 2018, 30, 1704528 CrossRef PubMed.
Footnote |
† Electronic supplementary information (ESI) available. See DOI: 10.1039/d1en00896j |
|
This journal is © The Royal Society of Chemistry 2022 |