Occurrence, human exposure, and risk of microplastics in the indoor environment
Received
19th July 2021
, Accepted 22nd November 2021
First published on 24th November 2021
Abstract
Microplastics (MPs) are a group of emerging contaminants that have attracted increasing scientific and societal attention over the past decade due to their ubiquitous detection in all environmental compartments. So far, most studies on MPs focus on characterizing their occurrence, fate, and impact in the aquatic environment. Therefore, very little is known about the magnitude, patterns, and associated risks of human exposure to MPs, particularly indoors. This is a significant research gap given that people spend most of their time (up to 90%) indoors, which is exacerbated over the past year by COVID-19 lockdown measures. Critical evaluation of the existing literature revealed the presence of MPs at higher concentrations in indoor air and dust (from homes and offices) compared to outdoors. This was attributed to several factors including: indoor MPs sources (e.g. furniture, textiles), increased deposition of atmospheric MPs indoors, and less atmospheric mixing and dilution compared to outdoor air. Current understanding is that indoor human exposure to MPs occurs via a combination of inhalation, ingestion, and dermal contact. Dietary intake was considered the major pathway of human exposure to MPs until recent studies revealed potential high exposure via inhalation. Moreover, exposure via inadvertent dust ingestion and dermal contact cannot be neglected, particularly for young children. This is alarming due to the potential toxic implications of MPs exposure. Early toxicological evidence indicates that small MPs (<20 µm) can cause oxidative stress and inflammation, while particles <5 µm can be engulfed by cells and translocated to accumulate in different organs. Also, there is increasing concern over potential leaching of toxic chemicals used as plastic additives (e.g. plasticizers and flame retardants) upon exposure to MPs due to their large surface area. However, MPs exposure and risk assessment in humans is still in its infancy and more research is necessary to provide the knowledge base required for regulations to protect human health and environment against MPs.
Environmental significance
So far, most studies on Microplastics (MPs) focus on characterizing their occurrence, fate, and impact in the aquatic environment. Therefore, very little is known about the magnitude, patterns, and associated risks of human exposure to MPs, particularly indoors. This is a significant research gap given that people spend up to 90% indoors, which is exacerbated over the past year by COVID-19 lockdown measures. Critical evaluation of existing literature revealed the presence of MPs at higher concentrations in indoor air and dust compared to outdoors. Dietary intake was considered the major pathway of human exposure to MPs until recent studies revealed potential high exposure via inhalation. Moreover, exposure via inadvertent dust ingestion and dermal contact cannot be neglected, particularly for young children. This is alarming due to the potential toxic implications of MPs exposure. However, MPs exposure and risk assessment in humans is still in its infancy and more research is necessary to provide the knowledge base required for regulations to protect human health and environment.
|
1. Introduction
Plastic contamination is considered a global threat to human and environmental health. The substantial increase in the worldwide annual production of plastics from 2 million tonnes in 1950 to over 300 million tonnes in 2018 has led to high levels of environmental plastic pollution.1 This is mainly due to the ability of plastics to accumulate in various environmental media owing to their resistance to chemical and/or biological degradation.2 Several types of polymers have been identified as the main sources of plastic pollution in different environmental compartments, including: polypropylene (PP), polyethylene (PE), polyethylene terephthalate (PET), polystyrene (PS), polyurethane (PUR), polyvinyl chloride (PVC), and polycarbonate (PC).3 While larger plastic waste items present an imminent visible risk to the environment and biota, there is increasing concern over the environmental and health impacts of smaller plastic fragments. Based on size, plastics can be classified into five major categories: “nanoplastic” (<0.03 cm), “microplastic” (<0.5 cm), “mesoplastic” (0.5–5 cm), “macroplastic” (5–50 cm), and “megaplastic” (>50 cm).4,5 Thompson (2006) was the first author to use the term “Microplastics (MPs)” for plastic particles such as fragments, pellets and fibres.6 MPs is inclusive of all types of plastic polymers in different shapes with a longest dimension < 5 mm, including nanoplastics, which are <0.1 mm.7 To further distinguish MPs from nanoplastics; Frias and Nash (2019) defined MPs as “synthetic solid particles or polymeric matrices, with regular or irregular shape and with size ranging from 1 µm to 5 mm, of either primary or secondary manufacturing origin, which are insoluble in water”.8 Accordingly, MPs can have various shapes, such as, pellets, films, foam, fragments, and microbeads. Also, MPs can be classified into “primary” and “secondary” based on their origin. Primary MPs are those manufactured or released to the environment in the “micro” size. This includes microbeads used in cosmetics, microfibres shed from clothing and other textiles upon washing, as well as micronized particles emitted from the wear of rubber tyres.9 Secondary MPs are produced from the breakdown of larger plastics in the environment as a result of natural weathering processes including photo-, chemical-, and biological degradation.10
MPs have become emerging pollutants of increasing global concern due to their ubiquitous detection in several environmental compartments including air, water, soil, sediment, and biota.5,11–13 However, most studies on MPs so far are centering on characterizing their occurrence, fate, and impact in the aquatic environment. Studies that have focused on MPs in terrestrial outdoor and indoor environments are limited compared to those in the marine/freshwater environment.14,15 Therefore, very little is known about the magnitude, patterns, and associated risks of human exposure to MPs in the terrestrial environment, particularly indoors.
Plastics were long considered inert materials due to the covalent-bonded polymer structure that renders them of high molecular weight, size, and hydrophobicity; thereby unavailable for absorption and readily excreted unchanged. However, recent studies revealed that MPs (particularly those < 1 mm) have the potential to cause adverse health effects to living organisms by: obstruction, inflammation, and/or accumulation in organs after translocation.14,16–18 Moreover, there is further concern over the potential release of hazardous plastic chemical additives to the environment and biota upon exposure to MPs containing such additives. These chemicals are added to plastic polymers at varying concentrations (up to 70% by mass) to impart some desired physical (e.g. colour, strength) or performance properties (e.g. flame-retardancy, flexibility).19 However, several classes of these plastic additives can cause serious adverse health effects (e.g. phthalates, bisphenols, organophosphates, biocides), as well as persistence and bioaccumulative properties (e.g. brominated flame retardants, chlorinated paraffins). This potential risk is aggravated by the high surface area to mass ratios of MPs, which may facilitate the leaching of these chemicals out of the plastic polymer upon human exposure to these particles.5,13,16
Against this backdrop, the present study aims to: (a) provide a critical review of the state-of-knowledge on the occurrence and concentrations of MPs in the indoor environment; (b) evaluate the existing literature on human exposure to MPs indoors via different exposure pathways; (c) investigate the potential risk(s) arising from such exposure; and finally (d) identify major research gaps and provide recommendations for future research to assess the risk of human exposure to MPs in the indoor environment.
2 Microplastics in the indoor environment
The indoor environment is important to modern human life due to the large proportion of time (up to 90% or more) that people spend indoors (e.g., homes, offices, transport etc.).20 Subsequently, indoor environmental quality has substantial implications for human health and wellbeing. This is further exacerbated by the various lockdown measures taken by several countries around the world to limit the spread of COVID-19, which may contribute to elevated exposure to emerging contaminants, such as MPs, indoors.
The following sections will summarise the current state-of-knowledge on the concentrations of MPs in environmental media relevant to indoor exposure. This includes indoor environment media (e.g. dust and air), as well as other exposure media relevant for indoor exposure (e.g. food and drinking water).
2.1 Settled dust
Indoor dust is considered an important medium for human exposure to several pollutants such as, construction materials, painting products, microorganisms, heavy metals such as lead, and several organic chemical pollutants (e.g. flame retardants and plasticisers).21–23 The occurrence of MPs at various concentrations, shapes, and sizes in indoor dust has been recently reported. A study on three different indoor sites in Paris including two private apartments and one office at the University of Paris-Est-Creteil pointed out that the number of MPs fibres in dust sampled from vacuum cleaner bags ranged from 1 to 60 fibres per m3. These concentrations exceeded those detected in outdoor dust (0.3 to 1.5 fibres per m3), indicating higher potential for human exposure to MPs via indoor than outdoor dust.24 In a worldwide study on MPs in house dust from 12 different countries including China, Colombia, Greece, Japan, Kuwait, and Saudi Arabia. MPs were detected in all 286 dust samples, at concentrations of 38–120
000 µg g−1 (median: 5900 µg g−1).25
A recent study investigated the presence of MPs in settled indoor dust collected from two offices, two schools, and two apartments in Surabaya, Indonesia. MP numbers measured in the two offices were: 334 particles on weekdays, 242 particles on a weekend (office 1); and 351 particles on weekdays, and 252 particles at the weekend (office 2). In schools they were: 290 particles on weekdays, and 239 particles at the weekend (school 1); and 321 particles on weekday, and 257 particles at the weekend (school 2), while in the apartments there were: 133 particles on weekdays, and 127 particles at the weekend (apartment 1); and 108 particles on weekdays, and 95 particles at the weekend (apartment 2). Despite the small sample size, it was evident that the number of MPs collected at each location during weekdays exceeded that found at weekends. This was attributed to the greater occupancy levels of the studied indoor environments on weekdays, which contribute to increasing MPs numbers through increased human activity.26
Liu et al. (2019) investigated indoor and outdoor dust samples from 39 different cities of China to determine two types of MPs, viz. polyethylene terephthalate (PET) and polycarbonate (PC). The results found PET MPs in all samples at high concentrations of 1550–120
000 mg kg−1 (indoor) and 212–9020 mg kg−1 (outdoor). PC MPs were detected in 70% of the samples, with median concentrations of 4.6 mg kg−1 (indoor) and 2.0 mg kg−1 (outdoor).27
Peng et al., (2020) developed a method to measure nylon MPs (polyamide, PA6, and PA66) in different environmental media including: indoor dust, sludge, marine sediment, freshwater sediment, and fishery sediment in China. The results revealed that PA6 and PA66 MPs in indoor dust were present at 0.431–86.3 and 3.10–92.9 mg kg−1, respectively. The authors also observed that the total concentrations of PA6 and PA66 MPs in indoor dust exceeded those in the other environmental matrices studied such as sludge, marine sediment, and freshwater sediment.28
2.2 Air
Generally, there are limited data on the levels and types of MPs in air compared to other environmental media.25,27,29 The presence of MPs in indoor air was attributed to emission and distribution from various sources including, furniture, municipal litter, and construction materials.30 Likewise, Webster et al., (2009) identified several sources of MPs to indoor air such as: textiles (mats, clothing, curtains, mattresses), toys, rubber, kitchen items (plates, cups, utensils, bowls, bottles), electrical cables, electronics, indoor paint, and cleaning agents.31
Dris et al. (2017) measured atmospheric MPs concentrations at three different indoor sites including two private apartments and one office in Paris. The results showed the concentrations of indoor air fibres in the studied sites ranged from 4.0–59.4 fibres per m3. The highest concentrations were observed in the office (4.0–59.4 fibres per m3), followed by apartment A (2.5–18.2 fibres per m3) and finally apartment B (1.1–16.3 fibres per m3). The variation was attributed to differences in emission of MPs fibres from indoor sources like furniture and carpets as a result of daily usage and/or cleaning activities. Concentrations of MPs in indoor air from all 3 sites exceeded those measured in outdoor air (0.3–1.5 fibres per m3). This was explained by the existence of indoor sources of the MPs fibres detected, as well as greater atmospheric dilution of MPs outdoors compared to indoors.32 Moreover, the rate of MPs deposition from air (also known as atmospheric fall out) in different indoor sites was reported to range from 1586 to 11
130 particles per m2 per day.32,33 These rates are substantially higher than those reported outdoors for a European metropolitan region (137–512 particles per m2 per day)34 and a remote conservation area in the United States (132 particles per m2 per day).35
Zhang et al., (2020c) investigated concentrations of MPs in indoor air at three different indoor microenvironments, namely: dormitory room, office, and a lecture building at East China Normal University. The results showed the highest average MPs abundance to be in the dormitory (9.9 × 103 MPs per m2 per day), followed by the office (1.8 × 103 MPs per m2 per day) then the corridor (1.5 × 103 MPs per m2 per day). The measured MPs existed mostly in the form of fibres, which had similar polymer compositions to the textile products used in the studied microenvironments. This led to the conclusion that textile quantity in an indoor microenvironment is one of the main factors affecting MPs abundance in indoor air, whereas high airflow turbulence can increase abrasion of textile fibres and MPs migration in indoor environments.36
Another investigation of airborne MPs both indoors and outdoors in coastal California, showed higher concentrations of MPs in indoor air (3.3 ± 2.9 fibres and 12.6 ± 8.0 fragments per m3) than in outdoor air (0.6 ± 0.6 fibres and 5.6 ± 3.2 fragments per m3). Also, the results showed that indoor MP fragments (58.6 ± 55 µm) were smaller in size than outdoor MP fragments (104.8 ± 64.9 µm). The authors concluded that the risk of inhalation exposure to MPs indoors is higher than outdoors due to increased abundance and smaller size of MPs in indoor air.37
A recent investigation of indoor air MPs from 20 houses in Hull, UK revealed an average household MPs concentration of 1414 MPs per m2 per day over a 6 month monitoring period. MP fibres (5–250 µm) were the most abundant shape of MPs (90%), while PET accounted for 62% of the identified MPs. Moreover, results suggested substantially higher human exposure to MPs (1–45 times) via inhalation indoors than outdoors.38
2.3 Drinking water
MPs were detected at varying concentrations in both tap water and bottled water.39,40Table 1 summarizes the current knowledge on the presence of MPs in drinking water. One of the potential reasons for presence of MPs in tap water is the possible erosion and/or degradation of the plastic components of distribution networks (e.g., pipes, tubing) leading to fragments of MPs entering drinking water.41 Also, using plastic bottles and caps as packaging for drinking water and potential degradation through the processes of manufacture, distribution, marketing, and repetitive use might be an important source of MPs in drinking water.42
Table 1 MPs in drinking waterd
Sample number and description |
Concentrations MPs per L |
Detection frequency (%) |
Polymer types |
Size |
Shape |
Reference |
Average |
Range |
Only particles ≥100 µm were verified with FTIR.
Water treatment plant.
Only fibres counted.
(PP): polypropylene, (PVC): polyvinyl chloride, (PA): polyamide (nylon), (PE): polyethylene, (PET): polyethylene terephthalate, (PS): polystyrene, (PTT): poly trim ethylene terephthalate, (PPS): polyphenylene sulfide, (PAA): polyacrylic acid, (PMPS): poly (methyl phenyl siloxane), (PI): poly (isoprene), N/A: not reported.
|
95 |
65 plastic bottles |
140 |
N/A |
100% |
PET 28.4%, PE 24.2%, PP 18.1%, PA 7.2%, PVC 4.4% |
6.5 to ≥50 µm |
Fibres and fragments |
Kankanige and Babel, (2020)108 |
30 glass-bottles |
52 |
7 |
Tap water |
0.7 |
0.3–1.6 |
100% |
Rayon, PET, PE, PS, polyester, PAA, PMPS, PI |
10–5000 µm |
Fibres and fragments |
Zhang et al., (2020b)109 |
38 |
Tap water |
440 |
0–1247 |
95% |
PE 26.8%, PP 24.4%, co PE–PP 22.0%, PPS 7.3%, PS 6.5%, PET 3.3% |
3–4453 µm |
Fragments, fibres, and spheres |
Tong et al., (2020)110 |
15 |
Tap water |
0.0007 |
0–0.0007 |
42% |
Polyester 62%, PVC 14%, PA and epoxy resin 9%, PE 6% |
>20 µm |
Fragments |
Mintenig et al., (2019)41 |
20 |
10 still water |
5.42 × 107 |
3.16 × 107 to 1.1 × 108 |
100% |
N/A |
0.5–10 µm |
N/A |
Zuccarello et al., (2019)111 |
10 sparkling water |
32 |
12 reusable plastic bottles |
4889 |
N/A |
100% |
PET, PP, PE |
>5 µm |
N/A |
Oßmann et al., (2018)134 |
10 single use plastic bottles |
2649 |
10 glass bottles |
6292 |
259 |
253 plastic bottles |
10.4a |
0–14 |
93% |
PP 54%, nylon 16% |
>100 µm |
Fragments, fibres, pellets and films |
Mason et al., (2018)40 |
6 glass bottles |
36 tap water from 3 WTPsb |
12 WTP1 |
443 |
N/A |
100% |
PET 44%, PP |
<10 µm |
Fragments, fibres, and spheres |
Pivokonsky et al., (2018)112 |
12 WTP2 |
338 |
PET 62%, PP |
12 WTP3 |
628 |
PET 26%, PP, PE 24% |
17 |
9 houses |
<0.58 |
N/A |
24% |
PP 50%, PS 25%, PET 25% |
>100 µm |
Fragments |
Strand et al., (2018)113 |
3 offices |
5 public institutions |
38 from mineral water |
15 reusable plastic bottles |
118 |
28–241 |
100% |
PET 84%, PP 7%, PE 5%, PA 2% |
50–500 µm |
Fragments |
Schymanski et al., (2018)48 |
11 single use plastic bottles |
14 |
2–44 |
3 beverage cartons |
11 |
5–20 |
9 glass bottles |
50 |
4–156 |
1 |
1 mineral water brand |
1 in the samplec |
N/A |
100% |
PET |
N/A |
Fibres |
Wiesheu et al., (2016)114 |
2.4 Food
Fish and seafood are considered major sources of MPs in the human diet because of the relatively early detection of different types of MPs in various types of fish, shrimp, shellfish, and bivalves.43–45,130 In addition, recent studies have reported the presence of MPs in different types of food and beverages such as sugar, honey, milk, table salt, soft drinks, and beer.46,47Table 2 summarises current knowledge on the presence of MPs in food and beverages. The extensive use of plastic materials in food packaging may be a reason for the presence of MPs in packaged food.48,49 The extent of MP release from food wraps/packages to packaged food remains unknown, although it is thought high based on the findings that one plastic tea bag can release millions of MPs into one cup of the hot beverage.49 Moreover, the potential for ingesting MPs depositing from the atmosphere onto the surface of food items cannot be neglected as it has been estimated to lead to exposure in the range 1.4–6.8 × 104 MPs per person per year.33
Table 2 MPs in food and beveragesa
Food type |
Sample number |
MPs average content |
Polymer types |
Size |
Shape |
Reference |
(PP): polypropylene, (PA): polyamide (nylon), (PE): polyethylene, (PET): polyethylene terephthalate, (PS): polystyrene, (PEST): polyester, (CP): cellophane, (HDPE): high-density polyethylene, (LDPE): high-density polyethylene, (PAAm): polyacrylamide, (PU): polyurethane, (PVC): polyvinyl chloride, (PS): polystyrene, (PAN): polyacrylonitrile, N/A: not reported.
|
Fish |
100 |
2.2 ± 0.89 MPs per individual |
PET 55%, PP 33%, PEST 6%, PU 2% |
<500 µm |
Fibres, films, fragments, foams, and granules |
Ghosh et al., (2021)115 |
Fish |
44 |
11.4 MPs items per fish (0.015 items per g wet weight) |
N/A |
<500 µm |
Fibres, fragments, and beads |
Taghizadeh Rahmat Abadi et al., (2021)116 |
Fish |
111 |
2.29 MPs per fish |
N/A |
0.5–4.75 mm |
Fibres, fragments, film, and beads |
Zakeri et al., (2020)117 |
Bivalves |
30 |
1.26 ± 0.39 to 1.56 ± 0.33 MPs per individual |
PP, PA, PE, PS, PET, PVC, PAN |
0.1–16.4 mm |
Fibres, fragments, and films |
Fang et al., (2019)118 |
Red sea fishes |
178 |
26 MPs per fish |
PP 42%, PET 42%, PVC 8%, PS 4%, PAN 4% |
2.39 ± 0.28 mm |
Fibres, fragments, and films |
Baalkhuyur et al., (2018)119 |
Canned sardines and sprats |
20 |
6 particles per item |
PP 33.3%, PET 33.3%, PE 16.6%, PVC 16.6% |
190–3800 µm |
Fragments, filaments, and films |
Karami et al., (2018)120 |
Table salts |
39 |
0–1674 particles per kg in sea salts |
PP, PE, PS, PET, PVC,PA |
100–5000 µm |
Fragments, fibres, sheets, and spherules |
Kim et al., (2018)121 |
0–148 particles per kg in rock salt |
28–462 particles per kg in lake salt |
Mussels (Mytilus edulis) |
162 |
0.7–2.9 MPs items per g of tissue (wet weight) |
PP, PEST, PET |
0.005–5 mm |
Fibres, fragments, spheres, and flakes |
Li et al., (2018)122 |
Molluscs |
123 |
0.2–21.0 MPs particles per g of soft tissue (wet weight) |
PE, PET, PA |
10–5000 µm |
Fragments, films, and pellets |
Naji et al., (2018)123 |
Mussels |
20 |
6.2–7.2 MPs items per g of tissue |
N/A |
1150–2290 µm |
Filaments |
Renzi et al., (2018)124 |
Sea salt |
6 Italian brands |
1.57–8.23 items per g (Italy) |
N/A |
4–2100 µm (Italian salts) |
Fibres, films, fragments, granules, and foams |
Renzi and Blašković, (2018)125 |
5 Croatian brands |
27.13–31.68 items per g (Croatia) |
15–4628 µm (Croatian salts) |
Fish |
212 |
1.56 ± 0.5 MPs per fish |
N/A |
0.38–3.1 mm |
Fibres, spheres, films, and fragments |
Bellas et al., (2016)126 |
Fish |
16 |
1–7 particles per fish |
PE 40%, PA 22%, PP 13%, PS 10%, PET 5%, PU 5%, PEST 5%. |
|
N/A |
Rummel et al., (2016)43 |
Fish |
64 |
2.3 pieces on average and up to 15 pieces per individual |
PE 52%, PP 43.3% |
150–1000 µm |
Fragments and beads |
Tanaka and Takada, (2016)127 |
Bivalves |
9 |
2.1–10.5 particles per g |
PE, PET, PA |
5–5000 µm |
Fibres, fragments, and pellets |
Li et al., (2015)128 |
Shrimp |
165 |
0.68 ± 0.55 MPs per g wet weight |
N/A |
|
Fibres, film, spherule, and fragment |
Devriese et al., (2015)44 |
Fish |
76 |
0–21 particles per individual fish |
N/A |
>500 µm |
Fragments, foam, and film |
Rochman et al., (2015)129 |
Blue mussels (Mytilus edulis) |
36 |
0.36 ± 0.07 items per g |
N/A |
5–25 µm |
N/A |
Van Cauwenberghe et al., (2015a)130 |
Table salt |
15 |
550–681 particles per kg in sea salts |
PET, PE, CP |
|
Fragments and fibres |
Yang et al., (2015)131 |
43–364 particles per kg in lake salts |
7–204 particles per kg in rock/well salts |
Blue mussel (Mytilus edulis) |
30 |
2.6–5.1 fibres/10 g of mussel |
N/A |
200–1500 µm |
Fibres |
De Witte et al., (2014)67 |
Honey |
19 |
166 ± 147 fibres per kg |
N/A |
10–20 µm |
Fragments and fibres |
Liebezeit and Liebezeit, (2013)132 |
9 ± 9 fragments per kg |
Sugar |
5 |
217 ± 123 fibres per kg |
N/A |
10–20 µm |
Fragments and fibres |
Liebezeit and Liebezeit, (2013)132 |
32 ± 7 fragments per kg |
Beer |
24 |
0.025 fibres per mL |
N/A |
10–20 µm |
Fragments, fibres, and granules |
Liebezeit and Liebezeit, (2014)133 |
0.033 fragments per mL |
0.017 granules per mL |
Milk |
10 |
137 fibres per L |
HDPE, LDPE 44%, PAAm 30%, PP 26% |
|
Fragments and fibres |
Diaz-Basantes et al., (2020)47 |
204 fragments per L |
Tea |
4 |
∼11.6 MPs per cup of the beverage |
PA, PET |
25 µm |
N/A |
Hernandez et al., (2019)49 |
3 Human exposure to MPs
Currently, little is known about human exposure to MPs, especially indoors. This may be attributed to several reasons including the lack of standardized methods for quantitative analysis of MPs in different matrices relevant to human exposure (e.g. indoor dust, air, diet, water and personal care products). This is compounded by the reporting of results as number of MPs in the majority of the few available studies, while most exposure assessment models require the exposure doses in mass units. Nevertheless, current understanding is that human exposure to MPs occurs via a combination of inhalation (air), ingestion (food, drinks, dust), and dermal contact (dust, fabrics, cosmetics).5,50Fig. 1 provides a schematic representation of the different pathways of human exposure to MPs and the risks associated with such exposure.
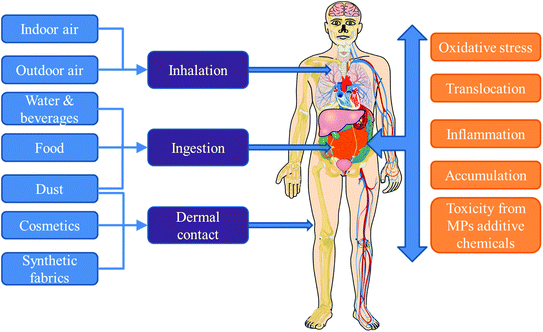 |
| Fig. 1 Pathways of human exposure to MPs and associated risks. | |
3.1 Inhalation
Inhalation has recently emerged as an important route of human exposure to MPs; due to their consistent detection at considerable concentrations in both indoor and outdoor air.36,51 Cox et al. (2019) highlighted the significance of the inhalation pathway through a meta-analysis of 26 studies on human exposure to MPs with a particular focus on the American population. Using an average concentration of 9.8 MPs per m3, results revealed adult inhalation exposure of 170 and 132 MPs per day for males and females, respectively, while children were exposed to 110 and 97 MPs per day. These estimates constituted ∼50% or more of the total daily exposure via all routes in the studied population groups indicating inhalation to be the major pathway of human exposure to MPs.46 A more recent study of MPs in Australian homes reported a mean inhalation intake of 0.2 mg per kg bw per year (equivalent to 12
891 MPs fibres per year) with the highest intake in young children at 0.31 mg per kg bw per year.52 Domenech and Marcos estimated a relatively low global human daily inhalation intake of 5.9 MPs per day based on average global airborne MPs concentration of 0.685 particles/m3 and breathing rate of 8.64 m3 per day.53 In earlier studies, individual MPs inhalation exposure was estimated to be between 26 and 132 MPs per day,54 while Vianello et al. reported mean inhalation of 272 MPs per day for average male adults doing light activity.55 Interestingly, a recent study highlighted the increased risk of MPs inhalation due to wearing different types of face masks during the COVID-19 pandemic. Fibres and spheres were the most abundant MP types detected, while activated carbon masks and N95 masks produced the highest and lowest levels of MPs inhalation, respectively.56
It is worth mentioning that meaningful comparison between the few available MPs inhalation exposure studies is difficult due to the different sampling, analysis and exposure assessment techniques. Such variation extends to the units for reporting concentrations of airborne MPs. Some studies report concentrations as number of MPs per m2 (measured atmospheric fallout area), while others report number of MPs per m3 (volume of air samples).57 Therefore, development of standardised methods for analysis of MPs in air is crucial for accurate and comparable exposure assessment results.
Currently, very little is known about inhalation exposure to MPs via resuspension of settled indoor dust. Several studies have provided experimental evidence on the contribution of resuspended house dust to inhalable airborne particulate matter. Resuspension of indoor dust occurs when the previously settled particles are detached from surfaces (e.g. carpets, floor) to become re-entrained into indoor air by human activities such as walking, crawling and vacuuming.58–61 Interestingly, toddlers were reported to experience higher inhalation exposure to contaminants in resuspended dust particles via crawling, compared to adult exposure via walking.58 Several studies have assessed the inhalation intake of various resuspended particulate-phase contaminants in indoor dust (e.g. PM10, PM2.5, bacteria).62–64 Resuspension of house dust was found to be equivalent to a PM2.5 source strength ranging from 0.03 to 0.5 mg min−1. Moreover, resuspension from walking on carpets was reported as the most significant contributor to both PM2.5 and PM10 exposure in the personal cloud (i.e. personal breathing zone) of retirement centre inhabitants.60,65 While MPs particles are expected to behave similarly, there exists no data on inhalation exposure to MPs via resuspension of settled indoor dust. This represents a significant knowledge gap suggesting that current reports of human inhalation exposure to MPs may be underestimated, particularly in toddlers.
3.2 Ingestion
Until recently, ingestion was widely considered as the main route of human exposure to MPs.66 This was led by a few early studies that identified MPs in various food items, particularly fish and seafood.67,68 Moreover, evidence has now emerged of potential human exposure to MPs via inadvertent ingestion of indoor dust.25
3.2.1 Diet.
Several studies have identified MPs at varying concentrations in different food items including fish, seafood, table salt, sugar, honey, milk, and beer (Table 2). This will inevitably lead to human exposure to these MPs via diet. However, few studies have estimated such dietary exposure. Cox et al., (2019) estimated the dietary consumption of MPs by American male adults, female adults, male children, and female children to be 142, 113, 126, and 106 particles per day, respectively.46 Another study estimated European adult exposure to MPs via consumption of table salt to range between 37 to 100 MPs per person per year.69 Elsewhere, the measured concentrations of MPs in fish and shellfish samples from Iran could contribute to an average daily adult exposure of 5 particles per day.70
The current state-of-knowledge on dietary exposure to MPs is far from comprehensive due to the lack of knowledge on MPs in major food groups (e.g. meat, vegetables, oil, dairy products etc.). This research gap was highlighted in a report by SAPEA (Science Advice for Policy by European Academies), which called for more comprehensive dietary studies to better characterise the risk associated with human dietary exposure to MPs.13 Another pertinent factor that requires further investigation is the release of MPs from plastic packaging to food and beverages which may result in elevated exposure. A study of hot beverages raised concern by reporting that brewing one plastic teabag at 95 °C releases approximately 11.6 billion MPs into a single cup of the beverage.49 Although the methods and techniques applied in this study leading to such high estimate of MPs was questioned,71 the potential release of MPs from plastic teabags has been confirmed. Another alarming study showed that polypropylene infant feeding bottles may release MPs resulting in concentrations as high as 16 million particles per litre due to repeated sterilisation and exposure to high-temperature water. The resulting exposure estimates ranged from 14
600–4
550
000 particles per capita per day, which demonstrated that infant exposure to MPs may be higher than previously recognised.72 A recent study provided evidence of the contamination of meat products with MPs from polystyrene packaging trays at concentrations of 4–18.7 MPs per kg of packaged meat. These particles were difficult to remove by rinsing and are probably cooked before consumption.73 Similarly, low concentrations of MPs (1–14 MPs per litre) were detected in branded milk packages from Mexico, likely as a result of leaching from the thermoplastic sulfone membrane filters used during the manufacturing process.74 Moreover, mechanical stress was identified as a factor that may influence human exposure to MPs via bottled drinks. Winkler et al. reported the frequent opening/closing of plastic bottles increases the number of MPs released into the bottled water via degradation; thereby increasing the chances of ingesting MPs.42
Collectively, these studies provide clear evidence on human exposure to MPs via diet in different countries and in different age groups from nursing infants to adults. There is even evidence on prenatal exposure to MPs via the placenta.75 However, more comprehensive, large scale studies are required for further understanding of the magnitude, profiles and associated risk of human dietary exposure to MPs.
3.2.2 Dust.
The role of indoor dust as an important matrix for human exposure to various groups of hazardous chemicals has been extensively highlighted over the past 2 decades. This has spurred some recent studies to investigate potential human exposure to MPs via unintentional ingestion of indoor dust. A study on polyethylene terephthalate (PET) and polycarbonate (PC) MPs in both indoor and outdoor dust samples from 39 cities in China reported the estimated daily intake (EDI) for different age groups to fall between 6500–89
700 ng per kg bw per day. Children were the highest exposed age group with a mean EDI of 17
300 ng per kg bw per day, while the mean EDIs of PET MPs in teenagers and adults were 7270 and 6500 ng per kg bw per day, respectively. Comparison between indoor and outdoor dust through EDI of PET MPs in all the studied age groups revealed higher exposure (almost double) via indoor dust.51 The ubiquity of human exposure to MPs via indoor dust, combined with higher intake and subsequent risk in younger age groups was confirmed by another study of MPs in house dust from 12 different countries. High concentrations of MPs (38–120
000 µg g−1) were detected; resulting in median EDI values of 4000–150
000 ng per kg bw per day for infants. Adults were less exposed with median EDI values of 360–12
000 ng per kg bw per day, which was mainly attributed to a combination of higher dust ingestion rate and lower body weight in infants.25 Similar findings were reported by a recent study on MPs in indoor dust from 32 Australian homes with estimated mean ingestion rates of 6.1 mg per kg bw per year (EDI = 16
712 ng per kg bw per day) for children and 0.5 mg per kg bw per year (EDI = 1370 ng per kg bw per day) for adults.52
Despite the small number of studies, it can be concluded that human exposure to MPs via ingestion of indoor dust and the potential risk of such exposure cannot be neglected, especially for children. More research is needed to understand the spatial and temporal variability of such exposure and the impact of individuals' time-activity patterns (e.g. the proportion of time spent in homes, offices, outdoors, cars, schools, and other types of microenvironments) on the overall daily exposure to MPs via dust ingestion in different age groups.
3.3 Dermal contact
To date, there are no available studies assessing human dermal exposure to MPs and its associated risks. However, with the ubiquitous occurrence of MPs in indoor dust, atmospheric deposition from both indoor and outdoor air, as well as wide application of microbeads in cosmetics and continuous degradation of microfibres from textiles; it is reasonable to consider dermal contact as a pathway of human exposure to MPs.53 Microplastic beads (microbeads, generally < 1 mm in diameter) have been widely applied in dermal exfoliation and cleansing products, as well as in toothpaste and denture fillings.76 Few studies have attempted estimation of per capita consumption of microbeads through the use of specific personal care products. A study on UK facial scrubs from different brands revealed a MPs content of 10–100 g L−1 resulting in per capita consumption of 40.5–215 mg per day.77 Gouin et al. estimated an average consumption of 2.4 mg MPs per day per capita for the US population through the use of liquid soap.78
While these few studies fall short of providing a comprehensive understanding on human dermal exposure to MPs, they provide evidence that the dermal route cannot be ignored. Although human skin can act as an efficient barrier against penetration of large particles and studies suggest that only particles <100 nm (i.e. nanoplastics) can directly cross the dermal barrier,50 there are other possible routes for transdermal penetration of larger particles through hair follicles, sweat gland, or open skin injuries.79 Moreover, skin damage as a result of inflammation and oxidative stress has been associated with dermal exposure to MPs.80 Therefore, more research is required to assess human dermal exposure to MPs via contact with cosmetics, settled dust particles, fabric fibres etc., as well as evaluating the significance of this exposure pathway and the associated health risk.
4 Microplastics risk to human health
Toxicological understanding of the potential impacts of MPs exposure on human health is still in its infancy.13 This may be attributed in part to the lack of accurate, sensitive and standardized analytical methods for determination of MPs in different human tissues (e.g. blood, milk) and exposure-relevant matrices (e.g. food, air). On the other hand, very little is known about toxicological endpoints of MPs and how they relate to environmentally-relevant human exposure doses.81 It is also not clear if the sizes, shapes, and chemical composition of the wide variety of environmental MPs available for human exposure via different pathways will impact on their toxicity in humans.82 Nevertheless, human internal exposure to MPs has been recently confirmed through the detection of MPs in human stool83 and placenta.75 This has spurred more research into the potential toxic implications of human exposure to MPs. Current understanding is that adverse health effects from MPs may be associated with particle toxicity and/or toxicity from associated chemicals (e.g. plasticisers, flame retardants, colourants etc.).84,85
4.1 MPs particle toxicity
Evidence on the toxicological impacts of airborne MPs in human is sparse. It is widely thought that MPs fibres <20 µm are predominant in air.13,86 Therefore, entry of such MPs into the airway is possible, although not as yet quantified. Larger MPs might be deposited in airways or trapped by the lung lining fluid and cleared out. However, smaller MPs may be engulfed by macrophages and epithelial cells.87 Accumulation of MPs fibres in human pulmonary tissue has been previously reported.88 Such accumulation of inhaled MPs in human airways may cause interstitial lung inflammatory and/or immune responses, and even cancer, although no large scale epidemiological associations or toxicological endpoints have been estimated for these adverse effects in humans.89 Moreover, small MPs (<2.5–5 µm) were reported to be capable of entering the systemic circulation through endocytosis and can be translocated to mesenteric lymph nodes, blood circulation, liver, and spleen.90 Increased permeability of the epithelial membrane due to inflammation was suggested as a plausible mechanism for this translocation of small MPs.91 This was supported by the observed increased haemolysis and subsequent release of histamine, a pro-inflammatory chemical, from human cell lines exposed to polypropylene MPs (20–200 µm).92 Another potential toxicological impact may be caused by inhalation of MPs carrying microbial colonisation. Pathogenic Vibrio spp. colonies were identified on a number of MPs particles (e.g. polyethylene, polypropylene and polystyrene) from North/Baltic sea.93 In addition to the risk of pathogenic species infections, inhaled MPs with microbial colonisation could cause a shift in the microbial community structure in the lung.13
Similar to inhaled MPs, current understanding is that intestinal absorption of ingested MPs is generally low with 90% or more of ingested particles excreted in faeces, depending on the particle size and shape.94 While an early study showed the mucus layer in the gut to present an effective barrier against the diffusion of 500 nm latex microbeads,95 more recent studies in human cell lines and lab animals indicate that MPs <10 µm can cross the gastrointestinal barrier and be translocated via the blood circulation to other organs (e.g. liver and kidney).96
Controlled laboratory studies in human cell lines and laboratory animals have revealed several potential toxic implications as a result of MPs exposure. Cytotoxicity of MPs on cerebral and epithelial human cell lines was attributed to oxidative stress caused by increased reactive oxygen species to high concentrations.97 This should be interpreted carefully though, because in other human cell lines (Caco-2), no significant cytotoxic effects were observed upon exposure to polystyrene MPs, even at high exposure doses.98,99 Other studies investigating the toxicity of MPs as particulate matter in air and water have reported on a range of potential toxicological impacts in animal models including immunosuppression and links to autoimmune diseases such as lupus erythematosus and autoimmune rheumatic disease.91 Other studies suggested possible neurotoxic effects due to increased activity of acetylcholinesterase enzyme in the brain and altered serum neurotransmitters levels in fish and mice.100,101 Exposure to MPs has been linked to obesity based on the increased food intake in mice as a response to higher energy demands or lower absorption efficiency associated with an observed decrease in liver weight and size.101 Prata (2018) suggested that chronic exposure to airborne MPs might be linked with cancer due to DNA damage caused by chronic inflammation and interstitial irritation.54
It is worth noting that extrapolating results from these controlled laboratory animal studies to humans should be done carefully and interpreted with caution due to inter-species variability, more complex and sophisticated immune and nervous systems, as well as the differences in MPs exposure levels and pathways in humans.
4.2 Toxicity from associated chemicals
A wide range of chemical additives are often incorporated into plastic polymers during manufacture at typical concentrations around 20% by weight (but can be up to 70% w/w) to impart specific properties or enhance the physical characteristics/appearance of the final product.19
Most of these additive chemicals (Table 3), particularly those used as flame retardants and/or plasticisers, have been well-documented to cause adverse health effects in humans including: endocrine disruption, reproductive toxicity, neurotoxicity, hepatotoxicity, and cancer.16,81,86 In the case of MPs, the concern over exposure to these toxic chemical additives is exacerbated by the small particle size and large surface area of MPs. This is likely to allow more surface area for these additive chemicals to leach out from the plastic polymer to human body fluids (e.g. sweat, gastric, intestinal and lung fluids) upon exposure (i.e. higher bioaccessibility).91 While the plethora of evidence on the potential adverse effects associated with exposure to MPs additive chemicals is concerning, very little is known about the leaching potential of these different chemical groups from various polymer types and consequently their potential toxicological impacts in humans.
Table 3 Common chemical groups used as plastic additives
Category |
Group |
Examples |
Functional additives |
Plasticizers |
Phthalates, bisphenols, organophosphate esters |
Flame retardants |
Brominated flame retardants, organophosphate flame retardants, chlorinated paraffins |
Stabilizers |
Nonylphenols, cadmium and lead compounds |
Biocides |
Triclosan, triclocarban |
Anti-static agents |
Quaternary ammonium compounds, alkylsulfonates, and alkylphosphates |
Slip agents |
Metallic stearates, fatty acid amides and waxes |
Colourants |
Soluble pigments |
Azo dyes, anthraquinones |
Organic pigments |
Cobalt diacetate |
Inorganic pigment |
Cadmium, zinc and chromium salts |
Reinforcements |
Fibres |
Carbon fibres, glass fibres |
Fillers |
|
Zinc oxide, talk, clay, calcium carbonate |
Another toxicological concern over MPs and associated chemicals is the so called “Trojan Horse effect”, which refers to the potential for accumulation of hazardous environmental chemical pollutants on the surface of MPs (aided by their large surface area) and this will lead to an increase in the chemical toxicity within the organism as the chemicals are then released when the plastic is ingested and or inhaled.102,103 While recent studies have demonstrated the potential for increased toxicity of polycyclic aromatic hydrocarbons (PAHs) in zebrafish102 and heavy metals in Daphnia,104 the Trojan Horse theory still needs verification in humans and higher trophic level animals. It is also possible that adsorption of chemical pollutants to MPs can reduce their bioaccessibility in organisms, through a reduced concentration in biological fluids, if the chemical co-pollutant is strongly bound to the MP and not desorbed easily, as reported in Northern Fulmars.105
5 Conclusion and future outlook
The extensive research on the identification and characterisation of MPs over the past decade has established their ubiquitous distribution in all environmental matrices. While the majority of this research was focused in the aquatic environment, few recent studies have raised concern over the existence of MPs in the indoor environment at concentrations relevant to human exposure. Current understanding is that human exposure to MPs in the indoor environment can occur through a combination of inhalation, ingestion, and dermal contact. The detection of MPs in indoor air and atmospheric deposition samples indicate inhalation as a significant exposure route, while the presence of MPs in indoor dust, food items, and beverages renders exposure via ingestion inevitable. Moreover, the use of MPs in cosmetics and personal care products, as well their release via the degradation of microfibres from domestic fabrics means the dermal exposure pathway cannot be neglected. This is alarming due to the fact that people spend up to 90% or more of their time indoors, recently exacerbated by COVID-19 pandemic lockdown measures. Critical evaluation of existing literature in the present review has revealed the paucity of information on human exposure to MPs indoors and the risk associated with it. The increasing public concern over MPs pollution and its potential detrimental effects on human health and the environment is escalating the pressure on regulators and policy makers to take decisive actions to mitigate such harmful effects. The knowledge base required for such informed decisions to protect public health against MPs pollution is still lacking. Significant research gaps exist in the current knowledge on both the sources and magnitude of human exposure MPs, as well as the relative contribution of different exposure pathways to human body burdens of MPs. The few studies available on the indoor environment mainly originate from developed countries, while very little is known about human exposure to MPs in low and middle income countries (LMIC) and the impact of different climates (e.g. less time spent indoors in tropical and subtropical regions) and cultures on such exposure (e.g. consumption of plastic packaged takeaway food and beverages). Therefore, the following topics are recommended for prioritisation in MPs research:
(a) There is an urgent need for validated, standardised methods for quantifying concentrations of MPs in indoor air and dust samples. It is also essential to reach a consensus on the units for reporting these concentrations on a mass basis suitable for exposure assessment models. Of particular importance is the harmonisation of units used for reporting airborne concentrations of MPs. The discrepancy between studies reporting concentrations as MPs per m2 (measured atmospheric fallout area) and those reporting MPs per m3 (volume of air samples) in the existing literature is hindering comparability between various studies. Moreover, the use of atmospheric fallout data (MPs per unit area) to assess human inhalational exposure is challenging because existing exposure models are based on inhalation rates in volume (m3) of air.
(b) Despite the few studies reporting MPs concentrations in human exposure-relevant media (e.g. air, dust and diet), very little is known about the magnitude of this exposure under real-life scenarios and for different age/sex groups. Therefore, comprehensive human exposure assessment studies are crucially required to estimate individual- and population-level exposure to MPs via total diet studies, market basket studies, dermal contact scenarios, and/or time-activity patterns (i.e. time spent in homes, offices, schools, nurseries, cars, outdoor exercise etc.) for different age groups in various countries.
(c) There is paucity of data on some sources and pathways that may contribute substantially to human exposure to MPs. More research is required to assess the resuspension of MPs particles from settled indoor dust to the personal cloud of adults and toddlers; thereby assessing its contribution to human inhalation exposure of MPs. Studies should also address the factors influencing this exposure source, such as: personal activity (e.g. walking adult, crawling toddler), indoor features (e.g. carpeted vs. wooden floors), rate of vacuuming, temperature and ventilation.
(d) While few recent studies assessed human exposure to MPs via food items (mainly seafood), very little is known on the contribution of food packaging and food-contact materials on human intake of MPs. Future studies should investigate the contribution of packaging (e.g. plastic boxes, films, covers, plates and utensils) on MPs concentrations in food and subsequent intake by different age groups in different cultures. Similarly, more research is required to understand the contribution of plastic bottles and caps to MPs exposure via drinking and the impact of beverage composition (e.g. probiotic drinks, multivitamin drinks) and temperature (e.g. hot coffee vs. cold juice) on the leaching of MPs from the bottle and consequent human exposure via drinking these beverages.
(e) Risk is assessed based on knowledge of both exposure and hazard. Recent studies have proposed risk assessment approaches for MPS in humans including probabilistic lifetime exposure models106 and multi-compartment exposure frameworks.107 However, more information on the toxicological implications and endpoints of MPs in humans (i.e. hazard) is essential to assess the risk arising from their exposure. In particular, more research is required on the particle-induced toxicity of MPs in human at exposure-relevant concentrations and MPs particle size ranges. Similarly, studies on the potential leaching and bioavailability of toxic chemical additives from human ingested/inhaled MPs are urgently required. These studies should preferably be conducted in human cell-lines and tissues, or pertinent mammalian laboratory animals due to the large inter-species variation that precludes meaningful extrapolation of toxicological endpoints from species like zebrafish and daphnia to humans.
Conflicts of interest
There are no conflicts to declare.
Acknowledgements
Hassan Khalid Ageel acknowledges gratefully the provision of his PhD studentship from the Kingdom of Saudi Arabia Ministry of Education.
References
- R. C. Hale, M. E. Seeley, M. J. La Guardia, L. Mai and E. Y. Zeng, A Global Perspective on Microplastics, J. Geophys. Res.: Oceans, 2020, 125(1), e2018JC014719, DOI:10.1029/2018JC014719.
- D. K. A. Barnes, F. Galgani, R. C. Thompson and M. Barlaz, Accumulation and fragmentation of plastic debris in global environments, Philos. Trans. R. Soc., B, 2009, 364(1526), 1985–1998 CrossRef CAS PubMed.
- W. C. Li, H. F. Tse and L. Fok, Plastic waste in the marine environment: A review of sources, occurrence and effects, Sci. Total Environ., 2016, 566–567, 333–349 CrossRef CAS.
- L. Lebreton, B. Slat, F. Ferrari, B. Sainte-Rose, J. Aitken and R. Marthouse,
et al., Evidence that the Great Pacific Garbage Patch is rapidly accumulating plastic, Sci. Rep., 2018, 8(1), 1–15 CAS.
- P. Wu, J. Huang, Y. Zheng, Y. Yang, Y. Zhang and F. He,
et al., Environmental occurrences, fate, and impacts of microplastics, Ecotoxicol. Environ. Saf., 2019, 184, 109612 CrossRef CAS.
- R. C. Thompson, Plastic debris in the marine environment: consequences and solutions, Mar. Nat. Conserv. Eur., 2006, 2006, 107–116 Search PubMed.
- O. S. Alimi, J. Farner Budarz, L. M. Hernandez and N. Tufenkji, Microplastics and Nanoplastics in Aquatic Environments: Aggregation, Deposition, and Enhanced Contaminant Transport, Environ. Sci. Technol., 2018, 52(4), 1704–1724 CrossRef CAS PubMed.
- J. P. G. L. Frias and R. Nash, Microplastics: Finding a consensus on the definition, Mar. Pollut. Bull., 2019, 138, 145–147 CrossRef CAS.
- M. A. Browne, P. Crump, S. J. Niven, E. Teuten, A. Tonkin and T. Galloway,
et al., Accumulation of microplastic on shorelines worldwide: Sources and sinks, Environ. Sci. Technol., 2011, 45(21), 9175–9179 CrossRef CAS.
- A. L. Andrady, Microplastics in the marine environment, Mar. Pollut. Bull., 2011, 62(8), 1596–1605 CrossRef CAS PubMed.
- P. Yao, B. Zhou, Y. H. Lu, Y. Yin, Y. Q. Zong and M.-T. Chen,
et al., A review of microplastics in sediments: Spatial and temporal occurrences, biological effects, and analytic methods, Quat. Int., 2019, 519, 274–281 CrossRef.
- K. Duis and A. Coors, Microplastics in the aquatic and terrestrial environment: sources (with a specific focus on personal care products), fate and effects, Environ. Sci. Eur., 2016, 28(1), 1–25 CrossRef CAS PubMed.
-
SAPEA, A Scientific Perspective on Microplastics in Nature and Society, Science Advice for Policy by European Academies, 2019, p. 176 Search PubMed.
- S. L. Wright, R. C. Thompson and T. S. Galloway, The physical impacts of microplastics on marine organisms: a review, Environ. Pollut., 2013, 178, 483–492 CrossRef CAS.
- S. C. Gall and R. C. Thompson, The impact of debris on marine life, Mar. Pollut. Bull., 2015, 92(1–2), 170–179 CrossRef CAS.
- B. Jiang, A. E. Kauffman, L. Li, W. McFee, B. Cai and J. Weinstein,
et al., Health impacts of environmental contamination of micro- and nanoplastics: a review, Environ. Health Prev. Med., 2020, 25(1), 29, DOI:10.1186/s12199-020-00870-9.
- X. Chang, Y. Xue, J. Li, L. Zou and M. Tang, Potential health impact of environmental micro- and nanoplastics pollution, J. Appl. Toxicol., 2020, 40(1), 4–15 CrossRef CAS.
- J. Wang, Z. Tan, J. Peng, Q. Qiu and M. Li, The behaviors of microplastics in the marine environment, Mar. Environ. Res., 2016, 113, 7–17 CrossRef CAS.
- J. N. Hahladakis, C. A. Velis, R. Weber, E. Iacovidou and P. Purnell, An overview of chemical additives present in plastics: Migration, release, fate and environmental impact during their use, disposal and recycling, J. Hazard. Mater., 2018, 344, 179–199 CrossRef CAS.
- N. E. Klepeis, W. C. Nelson, W. R. Ott, J. P. Robinson, A. M. Tsang and P. Switzer,
et al., The National Human Activity Pattern Survey (NHAPS): A resource for assessing exposure to environmental pollutants, J. Exposure Anal. Environ. Epidemiol., 2001, 11(3), 231–252 CrossRef CAS.
- F. Mercier, P. Glorennec, O. Thomas and B. L. Bot, Organic contamination of settled house dust, a review for exposure assessment purposes, Environ. Sci. Technol., 2011, 45(16), 6716–6727 CrossRef CAS.
- Y. Shan, W. Wu, W. Fan, T. Haahtela and G. Zhang, House dust microbiome and human health risks, Int. Microbiol., 2019 Sep, 22(3), 297–304 Search PubMed.
- S. Y. Tan, S. M. Praveena, E. Z. Abidin and M. S. Cheema, A review of heavy metals in indoor dust and its human health-risk implications, Rev. Environ. Health, 2016 Dec, 31(4), 447–456 Search PubMed.
-
R. Dris, J. Gasperi and B. Tassin, Sources and Fate of Microplastics in Urban Areas: A Focus on Paris Megacity, in Freshwater Microplastics, 2017, pp. 79–93 Search PubMed.
- J. Zhang, L. Wang and K. Kannan, Microplastics in house dust from 12 countries and associated human exposure, Environ. Int., 2020, 134, 105314 CrossRef CAS PubMed.
- I. Bahrina, A. D. Syafei, R. Satoto, J. J. Jiang, N. R. Nurasrin and A. F. Assomadi,
et al., An Occupant-Based Overview of Microplastics in Indoor Environments in the City of Surabaya, Indonesia, J. Ecol. Eng., 2020, 21(8), 236–242 CrossRef.
- K. Liu, X. Wang, N. Wei, Z. Song and D. Li, Accurate quantification and transport estimation of suspended atmospheric microplastics in megacities: Implications for human health, Environ. Int., 2019, 132, 105127 CrossRef CAS.
- C. Peng, X. Tang, X. Gong, Y. Dai, H. Sun and L. Wang, Development and Application of a Mass Spectrometry Method for Quantifying Nylon Microplastics in Environment, Anal. Chem., 2020, 92, 13930–13935 CrossRef CAS.
- S. Sridharan, M. Kumar, L. Singh, N. S. Bolan and M. Saha, Microplastics as an emerging source of particulate air pollution: A critical review, J. Hazard. Mater., 2021, 418, 126245 CrossRef CAS , https://www.sciencedirect.com/science/article/pii/S0304389421012097.
- R. Dris, J. Gasperi, M. Saad, C. Mirande and B. Tassin, Synthetic fibers in atmospheric fallout: A source of microplastics in the environment?, Mar. Pollut. Bull., 2016, 104(1–2), 290–293 CrossRef CAS.
- T. F. Webster, S. Harrad, J. R. Millette, R. D. Holbrook, J. M. Davis and H. M. Stapleton,
et al., Identifying transfer mechanisms and sources of decabromodiphenyl ether (BDE 209) in indoor environments using environmental forensic microscopy, Environ. Sci. Technol., 2009, 43(9), 3067–3072 CrossRef CAS PubMed.
- R. Dris, J. Gasperi, C. Mirande, C. Mandin, M. Guerrouache and V. Langlois,
et al., A first overview of textile fibers, including microplastics, in indoor and outdoor environments, Environ. Pollut., 2017, 221, 453–458 CrossRef CAS PubMed.
- A. I. Catarino, V. Macchia, W. G. Sanderson, R. C. Thompson and T. B. Henry, Low levels of microplastics (MP) in wild mussels indicate that MP ingestion by humans is minimal compared to exposure via household fibres fallout during a meal, Environ. Pollut., 2018, 237, 675–684 CrossRef CAS.
- M. Klein and E. K. Fischer, Microplastic abundance in atmospheric deposition within the Metropolitan area of Hamburg, Germany, Sci. Total Environ., 2019, 685, 96–103 CrossRef CAS.
- J. Brahney, M. Hallerud, E. Heim, M. Hahnenberger and S. Sukumaran, Plastic rain in protected areas of the United States, Science, 2020, 368(6496), 1257–1260 CrossRef CAS PubMed.
- Q. Zhang, Y. Zhao, F. Du, H. Cai, G. Wang and H. Shi, Microplastic Fallout in Different Indoor Environments, Environ. Sci. Technol., 2020, 54(11), 6530–6539 CrossRef CAS.
- E. Gaston, M. Woo, C. Steele, S. Sukumaran and S. Anderson, Microplastics Differ Between Indoor and Outdoor Air Masses: Insights from Multiple Microscopy Methodologies, Appl. Spectrosc., 2020, 74(9), 1079–1098 CrossRef CAS PubMed.
- L. C. Jenner, L. R. Sadofsky, E. Danopoulos and J. M. Rotchell, Household indoor microplastics within the Humber region (United Kingdom): Quantification and chemical characterisation of particles present, Atmos. Environ., 2021, 259, 118512 CrossRef CAS , https://www.sciencedirect.com/science/article/pii/S1352231021003332.
- M. Kosuth, S. A. Mason and E. V. Wattenberg, Anthropogenic contamination of tap water, beer, and sea salt, PLoS One, 2018, 13(4), 1–18 CrossRef PubMed.
- S. A. Mason, V. G. Welch and J. Neratko, Synthetic Polymer Contamination in Bottled Water, Front. Chem., 2018, 6, 407 CrossRef PubMed.
- S. M. Mintenig, M. G. J. Löder, S. Primpke and G. Gerdts, Low numbers of microplastics detected in drinking water from ground water sources, Sci. Total Environ., 2019, 648, 631–635 CrossRef CAS.
- A. Winkler, N. Santo, M. A. Ortenzi, E. Bolzoni, R. Bacchetta and P. Tremolada, Does mechanical stress cause microplastic release from plastic water bottles?, Water Res., 2019, 166, 115082 CrossRef CAS PubMed.
- C. D. Rummel, M. G. J. Löder, N. F. Fricke, T. Lang, E. M. Griebeler and M. Janke,
et al., Plastic ingestion by pelagic and demersal fish from the North Sea and Baltic Sea, Mar. Pollut. Bull., 2016, 102(1), 134–141 CrossRef CAS.
- L. I. Devriese, M. D. van der Meulen, T. Maes, K. Bekaert, I. Paul-Pont and L. Frère,
et al., Microplastic contamination in brown shrimp (Crangon crangon, Linnaeus 1758) from coastal waters of the Southern North Sea and Channel area, Mar. Pollut. Bull., 2015, 98(1–2), 179–187 CrossRef CAS.
- L. Van Cauwenberghe, L. Devriese, F. Galgani, J. Robbens and C. R. Janssen, Microplastics in sediments: A review of techniques, occurrence and effects, Mar. Environ. Res., 2015, 111, 5–17 CrossRef CAS PubMed.
- K. D. Cox, G. A. Covernton, H. L. Davies, J. F. Dower, F. Juanes and S. E. Dudas, Human Consumption of Microplastics, Environ. Sci. Technol., 2019, 53(12), 7068–7074, DOI:10.1021/acs.est.9b01517.
- M. F. Diaz-Basantes, J. A. Conesa and A. Fullana, Microplastics in honey, beer, milk and refreshments in Ecuador as emerging contaminants, Sustainability, 2020, 12(14), 5514 CrossRef.
- D. Schymanski, C. Goldbeck, H. U. Humpf and P. Fürst, Analysis of microplastics in water by micro-Raman spectroscopy: Release of plastic particles from different packaging into mineral water, Water Res., 2018, 129, 154–162 CrossRef CAS PubMed.
- L. M. Hernandez, E. G. Xu, H. C. E. Larsson, R. Tahara, V. B. Maisuria and N. Tufenkji, Plastic Teabags Release Billions of Microparticles and Nanoparticles into Tea, Environ. Sci. Technol., 2019, 53(21), 12300–12310, DOI:10.1021/acs.est.9b02540.
- M. Revel, A. Châtel and C. Mouneyrac, Micro(nano)plastics: A threat to human health?, Curr. Opin. Environ. Sci. Health, 2018, 1, 17–23 CrossRef.
- C. Liu, J. Li, Y. Zhang, L. Wang, J. Deng and Y. Gao,
et al., Widespread distribution of PET and PC microplastics in dust in urban China and their estimated human exposure, Environ. Int., 2019, 128, 116–124 CrossRef CAS PubMed.
- N. S. Soltani, M. P. Taylor and S. P. Wilson, Quantification and exposure assessment of microplastics in Australian indoor house dust, Environ. Pollut., 2021, 283, 117064 CrossRef CAS PubMed , https://linkinghub.elsevier.com/retrieve/pii/S0269749121006461.
- J. Domenech and R. Marcos, Pathways of human exposure to microplastics, and estimation of the total burden, Curr. Opin. Food Sci., 2021, 39, 144–151 CrossRef.
- J. C. Prata, Airborne microplastics: Consequences to human health?, Environ. Pollut., 2018, 234, 115–126 CrossRef CAS.
- A. Vianello, R. L. Jensen, L. Liu and J. Vollertsen, Simulating human exposure to indoor airborne microplastics using a Breathing Thermal Manikin, Sci. Rep., 2019, 9(1), 8670, DOI:10.1038/s41598-019-45054-w.
- L. Li, X. Zhao, Z. Li and K. Song, COVID-19: Performance study of microplastic inhalation risk posed by wearing masks, J. Hazard. Mater., 2021, 411, 124955 CrossRef CAS.
- Y. Huang, X. Qing, W. Wang, G. Han and J. Wang, Mini-review on current studies of airborne microplastics: Analytical methods, occurrence, sources, fate and potential risk to human beings, TrAC, Trends Anal. Chem., 2020, 125, 115821 CrossRef CAS.
- H. K. Hyytiäinen, B. Jayaprakash, P. V. Kirjavainen, S. E. Saari, R. Holopainen and J. Keskinen,
et al., Crawling-induced floor dust resuspension affects the microbiota of the infant breathing zone, Microbiome, 2018, 6(1), 25, DOI:10.1186/s40168-018-0405-8.
- R. D. Lewis, K. H. Ong, B. Emo, J. Kennedy, J. Kesavan and M. Elliot, Resuspension of house dust and allergens during walking and vacuum cleaning, J. Occup. Environ. Hyg., 2018, 15(3), 235–245 CrossRef CAS PubMed.
- P. E. Rasmussen, C. Levesque, M. Chénier and H. D. Gardner, Contribution of metals in resuspended dust to indoor and personal inhalation exposures: Relationships between PM10 and settled dust, Build. Environ., 2018, 143, 513–522 CrossRef , https://www.sciencedirect.com/science/article/pii/S0360132318304529.
- B. Wang, Z. Tang, Y. Li, N. Cai and X. Hu, Experiments and simulations of human walking-induced particulate matter resuspension in indoor environments, J. Cleaner Prod., 2021, 295, 126488 CrossRef CAS.
- S. D. Mitro, R. E. Dodson, V. Singla, G. Adarnkiewicz, A. F. Elmi and M. K. Tilly,
et al., Consumer Product Chemicals in Indoor Dust: A Quantitative Meta-analysis of US Studies, Environ. Sci. Technol., 2016, 50(19), 10661–10672 CrossRef CAS PubMed.
- L. D. Knibbs, C. He, C. Duchaine and L. Morawska, Vacuum Cleaner Emissions as a Source of Indoor Exposure to Airborne Particles and Bacteria, Environ. Sci. Technol., 2012, 46(1), 534–542 CrossRef CAS.
- T. Wu, M. Taubel, R. Holopainen, A.-K. Viitanen, S. Vainiotalo and T. Tuomi,
et al., Infant and Adult Inhalation Exposure to Resuspended Biological Particulate Matter, Environ. Sci. Technol., 2018, 52(1), 237–247 CrossRef CAS PubMed.
- C. E. Rodes, P. A. Lawless, G. F. Evans, L. S. Sheldon, R. W. Williams and A. F. Vette,
et al., The relationships between personal PM exposures for elderly populations and indoor and outdoor concentrations for three retirement center scenarios, J. Exposure Anal. Environ. Epidemiol., 2001, 11(2), 103–115 CrossRef CAS.
-
T. S. Galloway, Micro- and Nano-plastics and Human Health, in Marine Anthropogenic Litter, 2015, pp. 343–66 Search PubMed.
- B. De Witte, L. Devriese, K. Bekaert, S. Hoffman, G. Vandermeersch and K. Cooreman,
et al., Quality assessment of the blue mussel (Mytilus edulis): Comparison between commercial and wild types, Mar. Pollut. Bull., 2014, 85(1), 146–155 CrossRef CAS PubMed , https://www.sciencedirect.com/science/article/pii/S0025326X14003671.
- L. Van Cauwenberghe and C. R. Janssen, Microplastics in bivalves cultured for human consumption, Environ. Pollut., 2014, 193, 65–70 CrossRef CAS.
- A. Karami, A. Golieskardi, C. Keong Choo, V. Larat, T. S. Galloway and B. Salamatinia, The presence of microplastics in commercial salts from different countries, Sci. Rep., 2017, 7, 1–11 CrossRef CAS.
- S. Abbasi, N. Soltani, B. Keshavarzi, F. Moore, A. Turner and M. Hassanaghaei, Microplastics in different tissues of fish and prawn from the Musa Estuary, Persian Gulf, Chemosphere, 2018, 205, 80–87 CrossRef CAS PubMed.
- K. Busse, I. Ebner, H.-U. Humpf, N. Ivleva, A. Kaeppler and B. E. Oßmann,
et al., Comment on “Plastic Teabags Release Billions of Microparticles and Nanoparticles into Tea, Environ. Sci. Technol., 2020, 54(21), 14134–14135, DOI:10.1021/acs.est.0c03182.
- D. Li, Y. Shi, L. Yang, L. Xiao, D. K. Kehoe and Y. K. Gun'ko,
et al., Microplastic release from the degradation of polypropylene feeding bottles during infant formula preparation, Nat. Food, 2020, 1(11), 746–754, DOI:10.1038/s43016-020-00171-y.
- M. Kedzierski, B. Lechat, O. Sire, G. Le Maguer, V. Le Tilly and S. Bruzaud, Microplastic contamination of packaged meat: Occurrence and associated risks, Food Packag. Shelf Life, 2020, 24, 100489 CrossRef , https://www.sciencedirect.com/science/article/pii/S2214289419306738.
- G. Kutralam-Muniasamy, F. Pérez-Guevara, I. Elizalde-Martínez and V. C. Shruti, Branded milks – Are they immune from microplastics contamination?, Sci. Total Environ., 2020, 714, 136823 CrossRef CAS PubMed , https://www.sciencedirect.com/science/article/pii/S0048969720303338.
- A. Ragusa, A. Svelato, C. Santacroce, P. Catalano, V. Notarstefano and O. Carnevali,
et al., Plasticenta: First evidence of microplastics in human placenta, Environ. Int., 2021, 146, 106274 CrossRef CAS PubMed , https://www.sciencedirect.com/science/article/pii/S0160412020322297.
- L. Anagnosti, A. Varvaresou, P. Pavlou, E. Protopapa and V. Carayanni, Worldwide actions against plastic pollution from microbeads and microplastics in cosmetics focusing on European policies. Has the issue been handled effectively?, Mar. Pollut. Bull., 2021, 162, 111883 CrossRef CAS PubMed , https://www.sciencedirect.com/science/article/pii/S0025326X20310018.
- I. E. Napper, A. Bakir, S. J. Rowland and R. C. Thompson, Characterisation, quantity and sorptive properties of microplastics extracted from cosmetics, Mar. Pollut. Bull., 2015, 99(1), 178–185 CrossRef CAS , https://www.sciencedirect.com/science/article/pii/S0025326X1500449X.
- T. Gouin, N. Roche, R. Lohmann and G. Hodges, A Thermodynamic Approach for Assessing the Environmental Exposure of Chemicals Absorbed to Microplastic, Environ. Sci. Technol., 2011, 45(4), 1466–1472, DOI:10.1021/es1032025.
- M. Schneider, F. Stracke, S. Hansen and U. F. Schaefer, Nanoparticles and their interactions with the dermal barrier, Derm.-Endocrinol., 2009, 1(4), 197–206 CrossRef CAS PubMed.
- S. L. Wright and F. J. Kelly, Plastic and Human Health: A Micro Issue?, Environ. Sci. Technol., 2017, 51(12), 6634–6647 CrossRef CAS PubMed.
- L. Rubio, R. Marcos and A. Hernández, Potential adverse health effects of ingested micro- and nanoplastics on humans. Lessons learned from in vivo and in vitro mammalian models, J. Toxicol. Environ. Health, Part B, 2020, 23(2), 51–68, DOI:10.1080/10937404.2019.1700598.
- F. Amereh, M. Babaei, A. Eslami, S. Fazelipour and M. Rafiee, The emerging risk of exposure to nano(micro)plastics on endocrine disturbance and reproductive toxicity: From a hypothetical scenario to a global public health challenge, Environ. Pollut., 2020, 261, 114158 CrossRef CAS , https://www.sciencedirect.com/science/article/pii/S0269749119352066.
- P. Schwabl, S. Koppel, P. Konigshofer, T. Bucsics, M. Trauner and T. Reiberger,
et al., Detection of various microplastics in human stool: A prospective case series, Ann. Intern. Med., 2019, 171(7), 453–457 CrossRef PubMed.
- Q. Shi, J. Tang, R. Liu and L. Wang, Toxicity in vitro reveals potential impacts of microplastics and nanoplastics on human health: A review, Crit. Rev. Environ. Sci. Technol., 2021, 1–33, DOI:10.1080/10643389.2021.1951528.
- A. D. Vethaak and J. Legler, Microplastics and human health, Science, 2021, 371(6530), 672–674 CrossRef CAS PubMed.
- A. Rahman, A. Sarkar, O. P. Yadav, G. Achari and J. Slobodnik, Potential human health risks due to environmental exposure to nano- and microplastics and knowledge gaps: A scoping review, Sci. Total Environ., 2021, 757, 143872 CrossRef CAS , https://www.sciencedirect.com/science/article/pii/S0048969720374039.
- M. Geiser, B. Rothen-Rutishauser, N. Kapp, S. Schürch, W. Kreyling and H. Schulz,
et al., Ultrafine particles cross cellular membranes by nonphagocytic mechanisms in lungs and in cultured cells, Environ. Health Perspect., 2005 Nov, 113(11), 1555–1560 Search PubMed.
- J. L. Pauly, S. J. Stegmeier, H. A. Allaart, R. T. Cheney, P. J. Zhang and A. G. Mayer,
et al., Inhaled cellulosic and plastic fibers found in human lung tissue, Cancer Epidemiol., Biomarkers Prev., 1998, 7(5), 419–428 CAS.
- W. Huang, B. Song, J. Liang, Q. Niu, G. Zeng and M. Shen,
et al., Microplastics and associated contaminants in the aquatic environment: A review on their ecotoxicological effects, trophic transfer, and potential impacts to human health, J. Hazard. Mater., 2021, 405, 124187 CrossRef CAS PubMed , https://www.sciencedirect.com/science/article/pii/S0304389420321774.
- R. M. Urban, J. J. Jacobs, M. J. Tomlinson, J. Gavrilovic, J. Black and M. Peoc’h, Dissemination of wear particles to the liver, spleen, and abdominal lymph nodes of patients with hip or knee replacement, J. Bone Jt. Surg., Am. Vol., 2000, 82(4), 457–476 CrossRef CAS.
- J. C. Prata, J. P. da Costa, I. Lopes, A. C. Duarte and T. Rocha-Santos, Environmental exposure to microplastics: An overview on possible human health effects, Sci. Total Environ., 2020, 702, 134455 CrossRef CAS PubMed , https://www.sciencedirect.com/science/article/pii/S0048969719344468.
- J. Hwang, D. Choi, S. Han, J. Choi and J. Hong, An assessment of the toxicity of polypropylene microplastics in human derived cells, Sci. Total Environ., 2019, 684, 657–669 CrossRef CAS PubMed , https://www.sciencedirect.com/science/article/pii/S0048969719320832.
- I. V. Kirstein, S. Kirmizi, A. Wichels, A. Garin-Fernandez, R. Erler and M. Löder,
et al., Dangerous hitchhikers? Evidence for potentially pathogenic Vibrio spp. on microplastic particles, Mar. Environ. Res., 2016, 120, 1–8 CrossRef CAS.
- M. Smith, D. C. Love, C. M. Rochman and R. A. Neff, Microplastics in Seafood and the Implications for Human Health, Curr. Environ. Health Rep., 2018, 5(3), 375–386 CrossRef CAS PubMed.
- B. H. Bajka, N. M. Rigby, K. L. Cross, A. Macierzanka and A. R. Mackie, The influence of small intestinal mucus structure on particle transport ex vivo, Colloids Surf., B, 2015, 135, 73–80 CrossRef CAS PubMed , https://www.sciencedirect.com/science/article/pii/S0927776515300722.
- A. Banerjee and W. L. Shelver, Micro- and nanoplastic induced cellular toxicity in mammals: A review, Sci. Total Environ., 2021, 755, 142518 CrossRef CAS , https://www.sciencedirect.com/science/article/pii/S0048969720360472.
- G. F. Schirinzi, I. Pérez-Pomeda, J. Sanchís, C. Rossini, M. Farré and D. Barceló, Cytotoxic effects of commonly used nanomaterials and microplastics on cerebral and epithelial human cells, Environ. Res., 2017, 159, 579–587 CrossRef CAS.
- V. Stock, L. Böhmert, E. Lisicki, R. Block, J. Cara-Carmona and L. K. Pack,
et al., Uptake and effects of orally ingested polystyrene microplastic particles in vitro and in vivo, Arch. Toxicol., 2019 Jul, 93(7), 1817–1833 Search PubMed.
- M. Hesler, L. Aengenheister, B. Ellinger, R. Drexel, S. Straskraba and C. Jost,
et al., Multi-endpoint toxicological assessment of polystyrene nano- and microparticles in different biological models in vitro, Toxicol. In Vitro, 2019, 61, 104610 CrossRef CAS , https://www.sciencedirect.com/science/article/pii/S0887233319302607.
- L. G. A. Barboza, L. R. Vieira and L. Guilhermino, Single and combined effects of microplastics and mercury on juveniles of the European seabass (Dicentrarchus labrax): Changes in behavioural responses and reduction of swimming velocity and resistance time, Environ. Pollut., 2018, 236, 1014–1019 CrossRef CAS , https://www.sciencedirect.com/science/article/pii/S0269749117337995.
- Y. Deng, Y. Zhang, B. Lemos and H. Ren, Tissue accumulation of microplastics in mice and biomarker responses suggest widespread health risks of exposure, Sci. Rep., 2017, 7, 1–10 CrossRef PubMed.
- R. Trevisan, D. Uzochukwu and R. T. Di Giulio, PAH Sorption to Nanoplastics and the Trojan Horse Effect as Drivers of Mitochondrial Toxicity and PAH Localization in Zebrafish, Front. Environ. Sci., 2020, 8, 78 CrossRef PubMed , https://www.frontiersin.org/article/10.3389/fenvs.2020.00078.
- A. F. R. M. Ramsperger, V. K. B. Narayana, W. Gross, J. Mohanraj, M. Thelakkat and A. Greiner,
et al., Environmental exposure enhances the internalization of microplastic particles into cells, Sci. Adv., 2020, 6(50), 1211 CrossRef PubMed , https://advances.sciencemag.org/content/6/50/eabd1211.
- F. A. Monikh, M. G. Vijver, Z. L. Guo, P. Zhang, G. K. Darbha and W. Peijnenburg, Metal sorption onto nanoscale plastic debris and trojan horse effects in Daphnia magna: Role of dissolved organic matter, Water Res., 2020, 186, 116410 CrossRef.
- D. Herzke, T. Anker-Nilssen, T. H. Nost, A. Gotsch, S. Christensen-Dalsgaard and M. Langset,
et al., Negligible Impact of Ingested Microplastics on Tissue Concentrations of Persistent Organic Pollutants in Northern Fulmars off Coastal Norway, Environ. Sci. Technol., 2016, 50(4), 1924–1933 CrossRef CAS PubMed.
- N. Hazimah Mohamed Nor, M. Kooi, J. Diepens N and A. Koelmans A, Lifetime Accumulation of Microplastic in Children and Adults, Environ. Sci. Technol., 2021, 55(8), 5084–5096 CrossRef.
- S. Noventa, M. S. P. Boyles, A. Seifert, S. Belluco, A. S. Jiménez and H. J. Johnston,
et al., Paradigms to assess the human health risks of nano- and microplastics, Microplast. Nanoplast., 2021, 1(1), 9, DOI:10.1186/s43591-021-00011-1.
- D. Kankanige and S. Babel, Smaller-sized micro-plastics (MPs) contamination in single-use PET-bottled water in Thailand, Sci. Total Environ., 2020, 717, 137232 CrossRef CAS.
- M. Zhang, J. Li, H. Ding, J. Ding, F. Jiang and N. X. Ding,
et al., Distribution Characteristics and Influencing Factors of Microplastics in Urban Tap Water and Water Sources in Qingdao, China, Anal. Lett., 2020, 53(8), 1312–1327 CrossRef CAS.
- H. Tong, Q. Jiang, X. Hu and X. Zhong, Occurrence and identification of microplastics in tap water from China, Chemosphere, 2020, 252, 126493 CrossRef CAS.
- P. Zuccarello, M. Ferrante, A. Cristaldi, C. Copat, A. Grasso and D. Sangregorio,
et al., Exposure to microplastics (<10 µm) associated to plastic bottles mineral water consumption: The first quantitative study, Water Res., 2019, 157, 365–371 CrossRef CAS.
- M. Pivokonsky, L. Cermakova, K. Novotna, P. Peer, T. Cajthaml and V. Janda, Occurrence of microplastics in raw and treated drinking water, Sci. Total Environ., 2018, 643, 1644–1651 CrossRef CAS PubMed.
-
J. Strand, L. Feld, F. Murphy, A. Mackevica and N. B. Hartmann, Analysis of Microplastic Particles in Danish Drinking Water, DCE-Danish Centre for Environment and Energy, 2018, p. 34 Search PubMed.
- A. C. Wiesheu, P. M. Anger, T. Baumann, R. Niessner and N. P. Ivleva, Raman microspectroscopic analysis of fibers in beverages, Anal. Methods, 2016, 8(28), 5722–5725 RSC.
- G. C. Ghosh, S. M. Akter, R. M. Islam, A. Habib, T. K. Chakraborty and S. Zaman,
et al., Microplastics contamination in commercial marine fish from the Bay of Bengal, Reg. Stud. Mar. Sci., 2021, 44, 101728 CrossRef.
- Z. Taghizadeh Rahmat Abadi, B. Abtahi, H. P. Grossart and S. Khodabandeh, Microplastic content of Kutum fish, Rutilus frisii kutum in the southern Caspian Sea, Sci. Total Environ., 2021, 752, 141542 CrossRef CAS.
- M. Zakeri, A. Naji, A. Akbarzadeh and S. Uddin, Microplastic ingestion in important commercial fish in the southern Caspian Sea, Mar. Pollut. Bull., 2020, 160, 111598 CrossRef CAS PubMed.
- C. Fang, R. Zheng, H. Chen, F. Hong, L. Lin and H. Lin,
et al., Comparison of microplastic contamination in fish and bivalves from two major cities in Fujian province, China and the implications for human health, Aquaculture, 2019, 512, 734322 CrossRef CAS.
- F. M. Baalkhuyur, E. J. A. B. Dohaish, M. E. A. Elhalwagy, N. M. Alikunhi, A. M. AlSuwailem and A. Røstad,
et al., Microplastic in the gastrointestinal tract of fishes along the Saudi Arabian Red Sea coast, Mar. Pollut. Bull., 2018, 131, 407–415 CrossRef CAS PubMed.
- A. Karami, A. Golieskardi, C. K. Choo, V. Larat, S. Karbalaei and B. Salamatinia, Microplastic and mesoplastic contamination in canned sardines and sprats, Sci. Total Environ., 2018, 612, 1380–1386 CrossRef CAS.
- J. S. Kim, H. J. Lee, S. K. Kim and H. J. Kim, Global Pattern of Microplastics (MPs) in Commercial Food-Grade Salts: Sea Salt as an Indicator of Seawater MP Pollution, Environ. Sci. Technol., 2018, 52(21), 12819–12828 CrossRef CAS.
- J. Li, C. Green, A. Reynolds, H. Shi and J. M. Rotchell, Microplastics in mussels sampled from coastal waters and supermarkets in the United Kingdom, Environ. Pollut., 2018, 241, 35–44 CrossRef CAS PubMed.
- A. Naji, M. Nuri and A. D. Vethaak, Microplastics contamination in molluscs from the northern part of the Persian Gulf, Environ. Pollut., 2018, 235, 113–120 CrossRef CAS PubMed.
- M. Renzi, C. Guerranti and A. Blašković, Microplastic contents from maricultured and natural mussels, Mar. Pollut. Bull., 2018, 131, 248–251 CrossRef CAS.
- M. Renzi and A. Blašković, Litter & microplastics features in table salts from marine origin: Italian versus Croatian brands, Mar. Pollut. Bull., 2018, 135, 62–68 CrossRef CAS.
- J. Bellas, J. Martínez-Armental, A. Martínez-Cámara, V. Besada and C. Martínez-Gómez, Ingestion of microplastics by demersal fish from the Spanish Atlantic and Mediterranean coasts, Mar. Pollut. Bull., 2016, 109(1), 55–60 CrossRef CAS.
- K. Tanaka and H. Takada, Microplastic fragments and microbeads in digestive tracts of planktivorous fish from urban coastal waters, Sci. Rep., 2016, 6, 1–8 CrossRef.
- J. Li, D. Yang, L. Li, K. Jabeen and H. Shi, Microplastics in commercial bivalves from China, Environ. Pollut., 2015, 207, 190–195 CrossRef CAS PubMed.
- C. M. Rochman, A. Tahir, S. L. Williams, D. V. Baxa, R. Lam and J. T. Miller,
et al., Anthropogenic debris in seafood: Plastic debris and fibers from textiles in fish and bivalves sold for human consumption, Sci. Rep., 2015, 5, 1–10 Search PubMed.
- L. Van Cauwenberghe, M. Claessens, M. B. Vandegehuchte and C. R. Janssen, Microplastics are taken up by mussels (Mytilus edulis) and lugworms (Arenicola marina) living in natural habitats, Environ. Pollut., 2015, 199, 10–17 CrossRef CAS PubMed.
- D. Yang, H. Shi, L. Li, J. Li, K. Jabeen and P. Kolandhasamy, Microplastic Pollution in Table Salts from China, Environ. Sci. Technol., 2015, 49(22), 13622–13627 CrossRef CAS PubMed.
- G. Liebezeit and E. Liebezeit, Food Additives & Contaminants : Part A Non-pollen particulates in honey and sugar, Food Addit. Contam., Part A, 2013, 30(12), 2136–2140 CrossRef CAS.
- G. Liebezeit and E. Liebezeit, Synthetic particles as contaminants in German beers, Food Addit. Contam., Part A: Chem., Anal., Control, Exposure Risk Assess., 2014, 31(9), 1574–1578 CrossRef CAS.
- B. E. Oßmann, G. Sarau, H. Holtmannspötter, M. Pischetsrieder, S. H. Christiansen and W. Dicke, Small-sized microplastics and pigmented particles in bottled mineral water, Water Res., 2018, 141, 307–316 CrossRef.
|
This journal is © The Royal Society of Chemistry 2022 |