Polycyclic aromatic hydrocarbon occurrence in forest soils in response to fires: a summary across sites†
Received
7th September 2021
, Accepted 29th November 2021
First published on 30th November 2021
Abstract
Forest fires are important sources of polycyclic aromatic hydrocarbons (PAHs) in soils. However, factors controlling PAH production in soils subjected to fires in different sites are poorly understood. Here, we analyzed 143 sets of previously published data to evaluate the concentrations and composition profiles of PAHs in ash and soils associated with forest fires and to assess the impacts of soil depth, fire intensity, post-fire duration, and vegetation type on their occurrence. Compared to unburned soils, the total PAH concentrations increased by 205% (95% confidential interval of 152–269%; n = 136) in soils associated with fires. This increase surpassed that of PAH toxic equivalents (73%) because fires produce dominantly low-ring PAHs with relatively low toxicity. PAH concentrations in fire-impacted sites increased by 684%, 258%, and 155% in the ash, 0–5 cm soil depth interval, and >5 cm soil depth interval, respectively. The increases in PAH concentrations associated with mild-intensity fires (412%) exceeded those associated with moderate-intensity (163%) and high-intensity (168%) fires, which is possibly due to pyromineralization or volatilization of organic matters at high burning temperatures. These increases were highest within a month after the fire (280%), gradually decreasing over time, and showed no significant difference compared to the reference sites after 24 months. The concentration increases exhibit no major difference between various vegetation types (broad-leaved forest vs. coniferous forest vs. shrub). Assessments reveal that exposure to post-fire soil PAHs involves no serious human health risk. However, potential adverse effects of soil PAHs on other organisms (e.g., microbes and plants) and ecosystems should be further examined. The present study highlights the strong impacts of soil depth, fire intensity, and post-fire duration, and the relatively weak impact of the vegetation type on PAH concentrations in soils associated with fires in different areas.
Environmental significance
Regional climate warming and drought are increasing wildfire frequency and intensity in many regions worldwide. While wildfire is a well-recognized source of polycyclic aromatic hydrocarbons (PAHs), critical factors driving soil PAH occurrence have not yet been comprehensively analyzed. We conducted the first meta-analysis of PAH occurrence from previously reported wildfire cases. It allowed us to quantitatively identify the critical impacts of the soil depth, fire intensity, and post-fire duration, and the relatively weak impact of the vegetation type on elevating PAH levels in forest soils across various geographical locations. This study is significant in improving our understanding of potential wildfire impacts on environmental quality.
|
1. Introduction
Forest fires are considered among the most alarming and worrisome global ecological disasters because of their fierce nature, extensive devastation, difficulties in disposing the associated debris and rescuing trapped living things, related massive economic losses, and their significant impact on the climate.1–4 Owing to global warming, the frequency and intensity of forest fires have been increasing in recent decades in many regions.5,6 For example, between 1973 and 2012, fire records in western United States reveal that large-scale forest fire occurrence increased by approximately 40% per decade, and the area affected increased on an average by >390%.7 Many fires, such as those in Chile, Portugal, USA, and Australia in 2017, 2017, 2018, and 2019–2020, respectively, drew attention internationally.4 Forest fires alter the communities and diversity of plants, animals, and microbes, thereby inducing changes in the cycling of water, carbon, and pollutants.8–13 Owing to the increasing frequency of forest fires in recent decades, their impacts on environmental quality and human health are now of significant interest.
Forest soil represents a major long-term reservoir for persistent pollutants including polycyclic aromatic hydrocarbons (PAHs).14,15 The heat associated with forest fires combusts biomass to produce PAHs through reactions, such as dehydration, decarboxylation, and condensation, which eventually increase the amounts of PAHs in forest soils.13 Because of their carcinogenic, mutagenic, and teratogenic toxicity as well as ecotoxicity, quantifying the impacts of forest fires on the occurrence of PAHs in soils is crucial for risk assessments for human health and ecosystem health.15,16 However, previous studies have focused on changes in soil PAH concentrations linked to one or two fire events.1,17–19 Considering the differences in the characteristics of fire events and the dependence on the local climate, findings from these studies are sometimes inconsistent. For example, Chen et al.16 indicated that PAHs in ash produced by low-intensity fires were 1.7 times (on average) higher than those associated with high-intensity fires, and this is linked with a high degree of organic matter mineralization. In contrast, Kim et al.20 reported that PAHs produced by high-intensity fires were 2.8 times (on average) higher than those linked to low-intensity fires. In addition, the soil depth and vegetation type can also impact the occurrence of PAHs in soils affected by fires. Olivella et al.,18 for example, showed that the concentration of PAHs (19 ng g−1) in pine forest ash was more than 10 times higher than that of oak forest (1.3 ng g−1) ash, a month after a fire event. According to Campos et al.,1 the PAH concentrations in the ash and topsoil collected from a pine forest immediately after burning were correspondingly 52% and 64% higher than those from a eucalyptus forest. However, the effects of fire on soil PAH concentrations are transient because, according to several studies, PAH concentrations decrease dramatically within a year after such incidents.1,20–23 The rapid drop in PAH concentrations in the ash and soil within the first year after the fire is attributed to erosion by overland flows and selective leaching.23 However, the influences of factors, such as the soil depth, fire intensity, post-fire duration, and vegetation type, on the concentration and composition profiles of PAHs in soils after fire incidents involving many sites remain poorly investigated. Owing to the absence of meta-analysis of the impacts of fire on soil PAH occurrence and risks, understanding of factors driving the post-fire environmental behaviors and consequences of PAHs in fire-prone regions is limited.
In the present study, a meta-analysis of 143 sets of data from 51 areas with reported post-fire PAH concentrations in soils was performed. Changes in the concentrations, composition profiles, and potential health risks of PAHs after fires were evaluated and the impacts of the soil depth, fire intensity, post-fire duration, and vegetation type on the responses of soil PAH concentrations to fires were elucidated. We hypothesized that (1) after burning, the total PAH concentrations and toxic equivalents (TEQs) in soils increased depending on the soil layer, fire intensity, post-fire duration, and vegetation type; and (2) environmental health risks associated with PAHs in soils after burning are significant. The present study serves as a reference for evaluating the effects of forest fires on the environmental quality of soils within the context of global climate change.
2. Materials and methods
2.1 Data collection
In the present study, articles and dissertations on PAHs associated with forest fires for the period from 2000 to 2020 were retrieved from the Web of Science (Thomson Reuters, New York, NY, USA) and Google Scholar (Google Inc., Mountain View, CA, USA) using the following search terms: (i) wildfire OR forest fire OR grassland fire OR wildland fire; (ii) polycyclic aromatic hydrocarbons OR polynuclear aromatic hydrocarbons OR PAHs; and (iii) soil. The concentrations (ng g−1, dry weight) of 16 PAHs regulated by the U.S. Environmental Protection Agency (US EPA) and the total PAH concentrations were obtained for unburned soils (references) and ash/burned soils from 14 published experimental studies. The references were either soils collected before fires or soils from nearby unaffected areas. The data were sorted into the following categories: the (1) soil depth (ash, 0–5 cm (topsoil), and >5 cm (subsurface soil)); (2) fire intensity (mild, moderate, and high); (3) post-fire duration (<1 month, 1–6 months, 6–12 months, 12–24 months, and >24 months); and (4) vegetation type (broad-leaved forest, coniferous forest, and shrubs). When the description of the fire intensity was available in the papers, we categorized the burnt sites into mild-, moderate- and high-intensity groups. The fire intensity levels in these papers were mainly determined from the comprehensive fire information such as the height of canopy burnt, the consumption of litter, and the color of ash. Information on all samples and the associated data from various articles are presented in the ESI,† Data. xls. The locations of selected study sites, the fire intensity, and the effect sizes of fire on the PAH concentrations are plotted in Fig. S1.†
2.2 Data analysis
The 16 US EPA priority PAHs selected included naphthalene (Nap), acenaphthylene (Acy), acenaphthene (Ace), fluorene (Flu), phenanthrene (Phe), anthracene (Ant), fluoranthene (Flt), pyrene (Pyr), benzo[a]anthracene (BaA), chrysene (Chr), benzo[b]fluoranthene (BbF), benzo[k]fluoranthene (BkF), benzo[a]pyrene (BaP), dibenzo[a,h]anthracene (DahA), indeno[1,2,3-c,d]pyrene (IcdP), and benzo[g,h,i]perylene (BghiP). The logarithmic response ratio was used to describe the effect size in data analysis,24 and the formula for calculating the natural log response ratio (ln
RR) of PAH concentrations associated with fire is expressed as follows: | 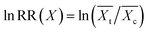 | (1) |
where
is the average PAH concentration in the reference soil (either the surface soil collected before the fire or surface soil from a nearby unaffected area) and
represents the average PAH concentration in the burned soil or ash. The TEQs of PAHs in the reference and burned samples were estimated through a weighted summation of the BaP TEQs of the 16 PAHs. To assess the carcinogenic risk of soil PAHs,25 the TEQ is calculated as follows: | 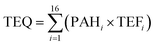 | (2) |
where PAHi is the concentration of a PAH and TEFi is the corresponding toxic equivalence factor.26
To calculate changes in TEQs of the PAHs in the soil, the following expression was utilized:
|  | (3) |
where

is the average TEQ of PAHs in the reference soil and

is the average TEQ of PAHs in the burned soil. If ln
![[thin space (1/6-em)]](https://www.rsc.org/images/entities/char_2009.gif)
RR is >0, it means that the PAH concentration or TEQ in the burned soil sample is higher than that of the reference. Conversely, if ln
![[thin space (1/6-em)]](https://www.rsc.org/images/entities/char_2009.gif)
RR is <0, it implies that the PAH concentration or TEQ in the burned soil sample is lower than that of the reference. The levels of statistical significance of various data sets were examined simultaneously. Effects were considered significant if the 95% confidence intervals (CIs) did not overlap with zero.
27,28 In addition, the between-group difference (
e.g., between sampling depths) in ln
![[thin space (1/6-em)]](https://www.rsc.org/images/entities/char_2009.gif)
RR was considered significant when
P <0.05 based on the examination on the within-group and between-group heterogeneities.
24 A significant difference was assigned to pairs of datasets without overlap in their confidence intervals, whereas those with overlapping confidence intervals were attributed to no significant difference.
29,30
The Ant/(Ant + Phe) and Flt/(Flt + Pyr) diagnostic ratios were calculated as follows:
| 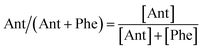 | (4) |
| 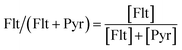 | (5) |
where [Ant], [Phe], [Flt], and [Pyr] are the concentrations of Ant, Phe, Flt, and Pyr, respectively, in the soil. These diagnostic ratios are commonly employed to differentiate between pyrogenic (combustion-related; Ant/(Ant + Phe) >0.1; Flt/(Flt + Pyr) >0.5) and petrogenic (non-combustion-related; Ant/(Ant + Phe) <0.1; Flt/(Flt + Pyr) <0.5) sources.
31,32 Considering that the areas in the present study are natural forests involving limited anthropogenic input, the PAH data of burned soils enabled us to assess the reliability of the diagnostic criteria for the pyrogenic origin of PAHs.
The hazard index (HI) and the incremental lifetime cancer risk (ILCR) described in the 2002 US EPA exposure factors handbook were employed to estimate the possible non-carcinogenic and carcinogenic health effects of PAHs in soils after fires, respectively. Humans are mainly exposed to these compounds in soils through the following pathways: inadvertent ingestion, dermal contact, and inhalation.33–36 Chronic daily intake (CDI, ng g−1 day−1) values of PAHs from soil through each avenue were estimated from the following expressions:
|  | (6) |
|  | (7) |
| 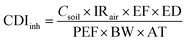 | (8) |
where
Csoil is the concentration (for HI calculation) or toxic equivalent (for ILCR calculation) of PAHs (ng g
−1), IR
soil is the ingestion rate from the soil (mg d
−1), EF denotes the exposure frequency (d a
−1), ED represents the exposure duration (a), CF is a conversion factor (1 × 10
−6 kg mg
−1), BW represents the average body weight (kg), AT is the average lifespan (d), SA stands for the surface area of the skin in contact with the soil (cm
2), AF
soil is the adherence factor of the skin to the soil (mg cm
−2), ABS is the dermal soil absorption factor (dimensionless), IR
air denotes the inhalation rate (m
3 d
−1), and PEF represents the particle emission factor (m
3 kg
−1). Based on the Risk Assessment Guidance of the US EPA and other publications, data for these parameters are presented in Table S1.
†
For non-carcinogenic PAHs, we compared the CDI value with the corresponding reference dose (RfD, kg day mg−1, Table S2†) to obtain the hazard quotient value (HQ) for each pathway using the following expression:
|  | (9) |
An HQ of 1 implies that the PAHs are harmful to humans, and the risk increases as the HQ increases. Conversely, an HQ <1 indicates that the PAHs have no evident non-carcinogenic effects on humans. The hazard index (HI), which represents the sum of the HQs, was used to assess the overall potential carcinogenic risk for humans linked to the three pathways, and this is expressed as follows:
| HI = HQing + HQderm + HQinh | (10) |
Therefore, at an HI >1, the hazard to human health requires consideration.37
The ILCR was used to express the lifetime probability of cancer incidence in a population exposed to pollutants. Although exposure to pollution may be low, the risk of cancer remains, if exposed for an extended duration. Thus, for carcinogenic PAHs, we estimated the ILCR using the product of the CDI and the corresponding carcinogenic slope factor (CSF, kg day mg−1), and this is expressed as follows:
The CDI was obtained by converting the concentration of PAH using the toxicity equivalent of BaP. The CSFs for ingestion, dermal contact, and inhalation exposure pathways of BaP were 7.3, 25, and 3.85 kg day mg−1, respectively.33 At an ILCR <10−6, the carcinogenic risk is negligible, while 10−6 to 10−4 represents a tolerable ILCR range. An ILCR >10−4 indicates a carcinogenic risk to humans.
3. Results and discussion
Following fire incidents, the PAH concentrations in the soils increased by an average of 205% (ln
RR = 1.11; n = 136; Fig. 1), while the TEQs increased by an average of 73% (ln
RR = 0.55; n = 136) compared with those of the unaffected soils (Fig. S2†). The ln
RR of the TEQs of the PAHs exhibit similar patterns to the ln
RR of the PAH concentrations, which explains their positive correlation (r2 = 0.47, P <0.0001). However, the concentration response is relatively higher than the toxicity response (Fig. 2, slope <1).
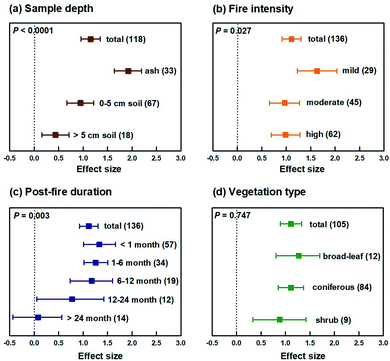 |
| Fig. 1 Changes in the PAH concentrations at different (a) sample depths, (b) fire intensities, (c) post-fire durations, and (d) vegetation types. Effect sizes are given as mean weighted natural log response ratios (ln RR) and error bars display the 95% confidence intervals; an effect size of 0 indicates no change relative to the reference, while values >0 or <0 indicate an increase or decrease, respectively. Numbers in parentheses represent the number of observations (N) for each parameter. P <0.05 indicates significant between-group heterogeneity. | |
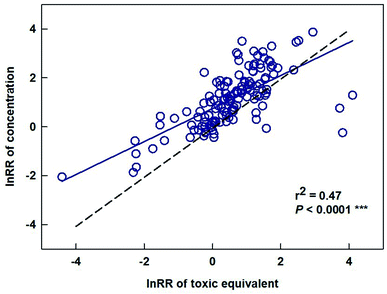 |
| Fig. 2 Plot showing the relationship between the response ratio (ln RR) of the toxic equivalents (TEQs) and the response ratio of the total PAH concentrations after burning. Out of the 136 data points, 111 are above the dashed line representing the 1 : 1 diagonal. | |
3.1 Factors controlling the post-fire soil PAH concentrations
3.1.1 Sample depth.
Based on the depth, the PAH concentrations are highest in the ash layer (584% increase; ln
RR = 1.92; n = 33), followed by the 0–5 cm soil layer (158% increase; ln
RR = 0.95; n = 67), and then the >5 cm soil layer (55% increase; ln
RR = 0.44; n = 18; Fig. 1a). Previous studies reporting elevated PAH concentrations in ash and surface soils after forest fires are common, whereas such studies are scarce for deeper soil layers. Our meta-analysis results confirm that PAH concentrations are highest in wildfire ash after a fire and the increase in concentration declines with increasing soil depth. These findings are consistent with those of previous studies,1,38 with ash being a direct product of the burning of vegetation and litter.1 Notably, the increase in PAH concentrations in soils deeper than 5 cm after burning was on an average less than half of that for soils between 0 and 5 cm. In fact, in some studies, the post-fire increases in PAH concentrations in the subsurface soils (5–15 cm) were insignificant.23
The relatively low PAH concentrations in subsurface soils could be attributed to at least two reasons. First, the relatively low temperature in subsurface soils could hardly support the in situ PAH generation. Generally, PAHs are formed from organic matter through incomplete combustion under high-temperature (>350 °C) conditions39 However, because of the low heat conductivity of surface soils, soils at depth experience limited heat from fires.40 In the case of a mild-intensity fire, the temperature of the surface soil seldom exceeds 100 °C, while the soil at depths greater than 5 cm hardly reaches 50 °C.13,41 The subsurface soil temperature associated with a high-intensity fire is comparable to that linked to a mild-intensity fire.42 According to previous studies, the surface soil reached 475 °C during a high-intensity fire, while the temperature at 5 cm was just 40 °C.43 Therefore, PAHs in soils at depth after a fire may be unrelated to in situ generations. Second, the hydrophobic layer at the soil surface can often form after fires, which will reduce the leaching of PAHs (particularly those with high molecular sizes and hydrophobicity) to the deeper layer.44 Overall, the low concentration of PAHs in the deep soil layer may be at least attributed to the low in situ production and relatively weak leaching of PAHs from the upper layer.1,16
3.1.2 Fire intensity.
The PAH data were partitioned based on the fire intensity into mild, moderate, and high groups. The average change in PAH concentrations in soils associated with mild-intensity fires (412% increase; ln
RR = 1.63; n = 29) are significantly higher than those for moderate- (163% increase; ln
RR = 0.97; n = 45) and high- (168% increase; ln
RR = 0.98; n = 62; Fig. 1b) intensity fires. The ash or soil PAH concentrations have been reported to decrease,16 increase,20 or show no significant change1 with increasing fire intensity. High forest fire intensity is usually associated with high fire temperatures and high organic matter consumption.16 Our dataset derived from different sites supports that mild-intensity fires produced more PAHs than moderate- and high-intensity fires. To the best of our knowledge, few, if any, studies have examined the mechanisms of PAH formation from controlled burning of forest fuels at different fire intensities. Nevertheless, lab biomass burning studies found that the PAH content in biomass started to increase at approximately 350 °C but would start to decrease when the temperature was higher than 500 °C.45–49 For example, char materials pyrolyzed at 450, 525, and 1000 °C had PAH concentrations of 16, 7, and 3 μg g−1, respectively.50 It is possible that the high temperature also promotes the pyromineralization16 and volatilization51 of the PAHs. In addition, during the high-temperature (>500 °C) combustion and pyrolysis, organic matters generated more graphitic black carbon with more than six rings instead of the extractable 16 priority PAHs.52,53
Our analysis also reveals that the total TEQ of PAHs differs significantly based on the fire intensities (Fig. S2†). This indicates that the toxicity associated with the mild fire intensity is relatively higher than those associated with the moderate and high fire intensities. This is mainly because of the high production of 2–3 ring PAHs after mild-intensity fires, based on their respective contributions to the total TEQ. The reason for the higher proportion of low-ring PAHs in mild-intensity fires could be that organic matter experienced greater volatilization and mineralization in moderate- and high-intensity fires.16
3.1.3 Post-fire duration.
The post-fire duration was divided into the following groups: <1 month, 1–6 months, 6–12 months, 12–24 months, and >24 months (Fig. 1c). The concentration of PAHs on an average is highest in the first month after fires (280% increase; ln
RR = 1.33, n = 57; Fig. 1). Subsequently, the PAH concentrations at 1–6 months (251% increase; ln
RR = 1.26; n = 34), 6–12 months (224% increase; ln
RR = 1.18; n = 19), 12–24 months (117% increase; ln
RR = 0.77; n = 12), and >24 months (no significant increase; ln
RR = 0.08; n = 14) decreased with increasing post-fire duration, and eventually attain the level of the reference.
Reduction in PAH concentrations with increasing time after a fire has been reported in many studies.1,20,22,23,51 According to these studies, the average PAH concentrations in a burned plot can be restored to the level of the reference in approximately two years. Fires commonly produce low-molecular-weight PAHs, which are easily volatilized, leached, and degraded.51 According to previous studies, soil erosion promoted by fires is a likely reason for the rapid decrease in PAH concentrations after fires.23 For example, in April 2000, a fire in Gangwon province in South Korea burned more than 17
000 ha of a forest, and the concentration of PAHs in the soil after the fire (up to 1200 ng g−1) was approximately 24 times higher than that of the unaffected (49 ng g−1) area. However, the soil PAH concentrations dissipated rapidly, returning to the level of the reference plot within five months. This was because the rainy season started two months after the fire and caused significant soil erosion and leaching.20 This suggests that under intense erosion, the soil PAH concentrations may return to the pre-fire level in less than two years (multiple site average). PAHs were adsorbed on ash/soil particles, which dissolve in surface runoff and enter the surface water system via soil erosion.1,20,22,23 According to previous studies, PAHs increase downstream after fires,19 which indicates that erosion and leaching loss represents an essential mechanism for reducing the soil PAH concentrations.54 Microbial degradation and plant uptake are other important degradation pathways for PAHs in soils.55 Direct metabolism and co-metabolism between plants and microorganisms enhance the degradation of PAHs in soil.55,56 Although PAHs in soils have been demonstrated to decline quickly after fires, their potential for polluting downstream waters and the atmosphere require further examination.
3.1.4 Vegetation types.
In our study, data were categorized into three groups including broad-leaved forest, coniferous forest, and shrubs (Fig. 1d). However, the meta-analysis results show no statistically significant difference in PAH concentrations among these groups. This implies that other factors (e.g., the soil depth, fire intensity, and post-fire duration) are more influential towards the soil PAH concentrations in different study sites. Nevertheless, based on field observations and laboratory investigations, some studies suggest that forest types represent a significant factor influencing the concentrations of PAHs in soils immediately after fires.1,57 For example, based on ash and surface soil samples collected immediately after fires, Campos et al.1 reported that the post-fire PAH concentrations in pine forest soil were 1.5 times higher than that in eucalyptus forest soil. In addition, Olivella noted that the concentration of PAHs in ash from a pine forest was about 15 times higher than that from an oak forest.18 Further, Rey-Salgueiro et al.57 demonstrated that the PAH concentrations in ash from pine trees were approximately twice that from eucalyptus trees based on simulated fires. Although these studies showed that plant species may influence the concentration and composition of PAHs in ash and surface soil within a short term after fire, the underlying mechanisms await further examination in the future.1,57
3.2 Impact of fire on PAH composition profiles
The concentration and toxic equivalent of each PAH ring group increased significantly relative to those in reference plots (Fig. 3). The increases of 2- and 3-ring PAHs (ln
RR of concentration = 1.66 and 1.60; ln
RR of toxic equivalent = 1.66 and 1.62, respectively) are significantly higher than those of 4-, 5-, and 6-ring PAHs (ln
RR of concentration = 0.91, 0.41, and 0.42; ln
RR of toxic equivalent = 0.76, 0.41, and 0.40, respectively).
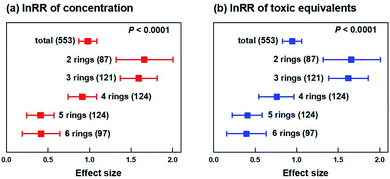 |
| Fig. 3 Plot showing changes in the concentrations of PAHs with different rings (2-, 3-, 4-, 5-, and 6-ring) in soils and the toxic equivalent responses after the fires. Effect sizes are shown as mean weighted log response ratios (ln RR) and the error bars represent 95% confidence intervals. An effect size of 0 indicates no change relative to the reference, while values >0 or <0 indicate an increase or decrease, respectively. Numbers in parentheses indicate the number of observations (N) for each parameter. P <0.05 indicates significant between-group heterogeneity. | |
Our results demonstrate that the contributions of PAHs involving different ring numbers to the total concentration and toxic equivalent are significantly altered by forest fires. Greater changes in the concentrations and toxic equivalent of 2–3-ring PAHs compared to the 5–6 ring PAHs support a greater impact of fires on the production of 2–3-ring PAHs.20,23,58
Diagnostic ratios are often employed for discriminating sources of PAHs.31,59 Among these, the Flt/(Flt + Pyr) ratio >0.5 and Ant/(Ant + Phe) ratio >0.1 are used to attribute PAHs to sources including fossil fuels, forest fires, and other combustion processes.31 Considering that biomass combustion is the principal known source of PAHs in soils after fires in the present study, various datasets enable an assessment of the reliability of these diagnostic ratios for PAHs derived from fire. According to the results, the Flt/(Flt + Pyr) and Ant/(Ant + Phe) ratios correspondingly indicate that 80.4% and 65.0% of the samples are derived from pyrogenic sources. The errors associated with both the Flt/(Flt + Pyr) and Ant/(Ant + Phe) are acceptable. Nevertheless, the Flt/(Flt + Pyr) ratio >0.5 is probably a more reliable diagnostic criterion for forest fire-derived PAHs than the Ant/(Ant + Phe) ratio >0.1 (Fig. 4).
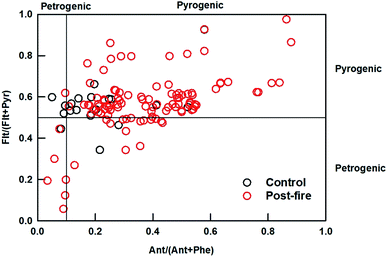 |
| Fig. 4 Plot for the diagnosis of the sources of PAHs in ash and soil layers after a forest fire. An Ant/(Ant + Phe) ratio >0.1 indicates that PAHs in the soil are mainly from a pyrogenic source, whereas a ratio <0.1 highlights a dominantly petrogenic source. Relatedly, an Flt/(Flt + Pyr) ratio >0.5 suggests a pyrogenic source, while a ratio <0.5 is primarily linked to a petrogenic source. | |
3.3 Geochemical and environmental implications
According to data in the fourth version of the Global Fire Emissions Database (GFED4), the global area burned in the preceding two decades was approximately 3.4 × 108 ha a−1;60,61 Assuming that moderate-intensity fires occur on such land areas around the world each year, approximately 1.6 × 107 g ash ha−1 will be generated,62 with a total post-fire increment of PAHs in fresh wildfire ash (<1 month after fire) of approximately 1634.1 ng g−1. A rough extrapolation shows that such a magnitude of forest fires around the globe may introduce nearly 8.9× 109 g of PAHs to soils each year. This value is relatively smaller compared to the fire-derived PAH emission to the atmosphere (6.8 × 1010 g a−1) estimated by Shen et al.63
According to environmental health risk analysis, the exposure of adults and children to PAHs in soils after fires falls within safe ranges for carcinogenic and non-carcinogenic risks. The carcinogenic and non-carcinogenic risks associated with the three exposure pathways are of comparable magnitude, but present the following order: ingestion > dermal > inhalation.
The ILCR results demonstrate relatively low carcinogenic risk levels for adults and children via the three soil exposure pathways after fires (Table 1). ILCR levels from 10−6 to 10−4 indicate potential health risks, while values >10−4 suggest high potential health risks. In the present study, the carcinogenic risks associated with the PAH levels from the forest fires for adults and children are negligible, irrespective of the exposure pathway. Based on the highest PAH concentrations that occur within the first month after fires, the calculated ILCR risk levels remain lower than 10−6.
Table 1 Calculated health risk levels associated with PAHs in soil after a fire incident (the average values are shown with the ranges in parentheses)
Health risk |
Exposure pathways |
Adult |
Child |
ILCR levels |
Ingestion |
2.47 × 10−8 (1.76 × 10−12 to 6.89 × 10−7) |
5.75 × 10−8 (4.11 × 10−12 to 4.75 × 10−7) |
Dermal contact |
1.65 × 10−8 (1.18 × 10−12 to 4.61 × 10−7) |
2.95 × 10−8 (2.11 × 10−12 to 8.23 × 10−7) |
Inhalation |
1.19 × 10−12 (1.37 × 10−14 to 5.35 × 10−11) |
8.48 × 10−13 (6.06 × 10−16 to 2.37 × 10−11) |
HQ levels |
Ingestion |
1.15 × 10−4 (4.89 × 10−8 to 5.09 × 10−3) |
2.67 × 10−4 (1.14 × 10−7 to 1.19 × 10−2) |
Dermal contact |
1.09 × 10−6 (1.65 × 10−9 to 2.60 × 10−5) |
1.93 × 10−6 (2.95 × 10−9 to 4.65 × 10−5) |
Inhalation |
8.76 × 10−9 (1.44 × 10−11 to 3.77 × 10−7) |
3.89 × 10−9 (6.38 × 10−12 to 1.67 × 10−7) |
The HQ results also reveal low non-carcinogenic risk levels for adults and children via the three exposure pathways (Table 1). The total non-carcinogenic risks (HI) for both adults and children for the three pathways are approximately 10−4. In the present study, all HQ and HI values are significantly <1, and these indicate no non-carcinogenic risk. Nap was the dominant contributor to the non-carcinogenic risks based on the three exposure pathways for both adults and children. The ingestion and dermal contact exposure risks are higher for children than adults, because the former are more susceptible to accidental eating and contact incidents.
However, because of differences in the exposure levels and individuals, the human health risk assessment involves significant uncertainty. Therefore, although the assessment results indicate exposures to PAHs fall within safe ranges, caution is still required. In fact, considering the variations associated with the fire intensity, post-fire duration, and soil type, the human health risks of PAHs in soils after fires are complicated. In addition to human beings, the soil health and local communities of plants, microbes, and animals may also be impacted by the elevated PAH levels after fires, which would further affect the ecosystem services.64 It should also be noted that risks linked to PAHs released into the atmosphere65–67 and to water bodies15,68,69 have not been considered in the present study and should be also carefully examined in the future.
4. Conclusions
In the present study, we synthesized previous findings on the effects of forest fires on soil PAH concentrations using a meta-analysis approach. The soil layer, fire intensity, and post-fire duration were found to simultaneously affect the soil PAH concentrations in response to fire, while the vegetation type was a less important factor. The PAH concentrations and toxicity levels in soils increased significantly after fires, and then declined to the levels of unaffected areas within two years. Considering that PAHs produced by fires are predominantly low-molecular-weight compounds characterized by low toxic potentials, the increase of the TEQs on an average was less than that of concentrations. The risk assessment results showed that human exposure to PAHs in the post-fire soils was within safe levels, which indicated that forest fires minimally impact soil quality in relation to PAHs. However, although low-molecular-weight PAHs are less toxic, their solubility and volatility are notably higher than those of high-molecular-weight PAHs. Therefore, the transfer of these low-molecular-weight compounds from the soil to water or the atmosphere may pose risks to other environmental media and requires further investigation.
Conflicts of interest
There are no conflicts to declare.
Acknowledgements
This work was financially supported by the Shenzhen Science & Technology Project (20200925155227003 and JCYJ20190809142611503), National Natural Science Foundation of China (42192513 and 41807360), Guangdong Natural Science Funds for Distinguished Young Scholars (2021B1515020082), and Key Platform and Scientific Research Projects of Guangdong Provincial Education Department (2019KZDXM028 and 2020KCXTD006).
References
- I. Campos, N. Abrantes, P. Pereira, A. C. Micaelo, C. Vale and J. J. Keizer, Forest fires as potential triggers for production and mobilization of polycyclic aromatic hydrocarbons to the terrestrial ecosystem, Land Degrad. Dev., 2019, 30, 2360–2370, DOI:10.1002/ldr.3427.
- S. H. Doerr and C. Santín, Global trends in wildfire and its impacts: perceptions versus realities in a changing world, Philos. Trans. R. Soc., B, 2016, 371, 20150345, DOI:10.1098/rstb.2015.0345.
- W. M. Jolly, M. A. Cochrane, P. H. Freeborn, Z. A. Holden, T. J. Brown, G. J. Williamson and D. M. J. S. Bowman, Climate-induced variations in global wildfire danger from 1979 to 2013, Nat. Commun., 2015, 6, 7537, DOI:10.1038/ncomms8537.
- D. M. J. S. Bowman, C. A. Kolden, J. T. Abatzoglou, F. H. Johnston, G. R. van der Werf and M. Flannigan, Vegetation fires in the anthropocene, Nat. Rev. Earth Environ., 2020, 1, 500–515, DOI:10.1038/s43017-020-0085-3.
- K. Fang, Q. Yao, Z. Guo, B. Zheng, J. Du, F. Qi, P. Yan, J. Li, T. Ou, J. Liu, M. He and V. Trouet, ENSO modulates wildfire activity in China, Nat. Commun., 2021, 12, 1764, DOI:10.1038/s41467-021-21988-6.
- X. J. Walker, J. L. Baltzer, S. G. Cumming, N. J. Day, C. Ebert, S. Goetz, J. F. Johnstone, S. Potter, B. M. Rogers, E. A. G. Schuur, M. R. Turetsky and M. C. Mack, Increasing wildfires threaten historic carbon sink of boreal forest soils, Nature, 2019, 572, 520–523, DOI:10.1038/s41586-019-1474-y.
- A. L. Westerling, Increasing western US forest wildfire activity: sensitivity to changes in the timing of spring, Philos. Trans. R. Soc., B, 2016, 371, 20150178, DOI:10.1098/rstb.2015.0178.
- I. Campos, N. Abrantes, J. J. Keizer, C. Vale and P. Pereira, Major and trace elements in soils and ashes of eucalypt and pine forest plantations in portugal following a wildfire, Sci. Total Environ., 2016, 572, 1363–1376, DOI:10.1016/j.scitotenv.2016.01.190.
- J. Kong, J. Yang and E. Bai, Long-Term effects of wildfire on available soil nutrient composition and stoichiometry in a Chinese boreal forest, Sci. Total Environ., 2018, 642, 1353–1361, DOI:10.1016/j.scitotenv.2018.06.154.
- A. V. Bogorodskaya, E. N. Krasnoshchekova, I. N. Bezkorovainaya and G. A. Ivanova, Post-fire transformation of microbial communities and invertebrate complexes in the pine forest soils, central Siberia, Contemp. Probl. Ecol., 2010, 3, 653–659, DOI:10.1134/s199542551006007x.
- J. P. Nunes, S. H. Doerr, G. Sheridan, J. Neris, C. Santín, M. B. Emelko, U. Silins, P. R. Robichaud, W. J. Elliot and J. Keizer, Assessing water contamination risk from vegetation fires: challenges, opportunities and a framework for progress, Hydrol. Processes, 2018, 32, 687–694, DOI:10.1002/hyp.11434.
- S. Verma and S. Jayakumar, Impact of forest fire on physical, chemical and biological properties of soil: a review, Proc. Int. Acad. Ecol. Environ. Sci., 2012, 2, 168–176 CAS.
- H. Knicker, How does fire affect the nature and stability of soil organic nitrogen and carbon? A review, Biogeochemistry, 2007, 85, 91–118, DOI:10.1007/s10533-007-9104-4.
- K. Srogi, Monitoring of environmental exposure to polycyclic aromatic hydrocarbons: a review, Environ. Chem. Lett., 2007, 5, 169–195, DOI:10.1007/s10311-007-0095-0.
- I. Campos and N. Abrantes, Forest fires as drivers of contamination of polycyclic aromatic hydrocarbons to the terrestrial and aquatic ecosystems, Curr. Opin. Environ. Sci. Health, 2021, 24, 100293, DOI:10.1016/j.coesh.2021.100293.
- H. Chen, A. T. Chow, X. W. Li, H. G. Ni, R. A. Dahlgren, H. Zeng and J. J. Wang, Wildfire burn intensity affects the quantity and speciation of polycyclic aromatic hydrocarbons in soils, ACS Earth Space Chem., 2018, 2, 1262–1270, DOI:10.1021/acsearthspacechem.8b00101.
- E. J. Kim, J. E. Oh and Y. S. Chang, Effects of forest fire on the level and distribution of PCDD/Fs and PAHs in soil, Sci. Total Environ., 2003, 311, 177–189, DOI:10.1016/s0048-9697(03)00095-0.
- M. A. Olivella, T. G. Ribalta, A. R. de Febrer, J. M. Mollet and F. X. C. de las Heras, Distribution of polycyclic aromatic hydrocarbons in riverine waters after Mediterranean forest fires, Sci. Total Environ., 2006, 355, 156–166, DOI:10.1016/j.scitotenv.2005.02.033.
- M. Vila-Escale, T. Vegas-Vilarrúbia and N. Prat, Release of polycyclic aromatic compounds into a Mediterranean Creek (Catalonia, NE Spain) after a forest fire, Water Res., 2007, 41, 2171–2179, DOI:10.1016/j.watres.2006.07.029.
- E. J. Kim, S. D. Choi and Y. S. Chang, Levels and patterns of polycyclic aromatic hydrocarbons (PAHs) in soils after forest fires in South Korea, Environ. Sci. Pollut. Res., 2011, 18, 1508–1517, DOI:10.1007/s11356-011-0515-3.
- M. S. García-Falcón, B. Soto-González and J. Simal-Gándara, Evolution of the concentrations of polycyclic aromatic hydrocarbons in burnt woodland soils, Environ. Sci. Technol., 2006, 40, 759–763, DOI:10.1021/es051803v.
- E. Simon, S. D. Choi and M. K. Park, Understanding the fate of polycyclic aromatic hydrocarbons at a forest fire site using a conceptual model based on field monitoring, J. Hazard. Mater., 2016, 317, 632–639, DOI:10.1016/j.jhazmat.2016.06.030.
- A. Vergnoux, L. Malleret, L. Asia, P. Doumenq and F. Theraulaz, Impact of forest fires on pah level and distribution in soils, Environ. Res., 2011, 111, 193–198, DOI:10.1016/j.envres.2010.01.008.
- L. V. Hedges, J. Gurevitch and P. S. Curtis, The Meta-analysis of response ratios in experimental ecology, Ecology, 1999, 80, 1150–1156, DOI:10.1890/0012-9658(1999)080[1150:tmaorr]2.0.co;2.
- S. C. Chen and C. M. Liao, Health risk assessment on human exposed to environmental polycyclic aromatic hydrocarbons pollution sources, Sci. Total Environ., 2006, 366, 112–123, DOI:10.1016/j.scitotenv.2005.08.047.
- I. C. T. Nisbet and P. K. LaGoy, Toxic equivalency factors (TEFs) for polycyclic aromatic hydrocarbons (PAHs), Regul. Toxicol. Pharmacol., 1992, 16, 290–300, DOI:10.1016/0273-2300(92)90009-x.
- R. L. Collins, G. A. Parks and G. A. Marlatt, Social determinants of alcohol consumption: the effects of social interaction and model status on the self-administration of alcohol, J. Consult. Clin. Psychol., 1985, 53, 189–200, DOI:10.1037/0022-006x.53.2.189.
- W. V. den Noortgate, M. C. Opdenakker and P. Onghena, The effects of ignoring a level in multilevel analysis, Sch. Effect. Sch. Improv., 2005, 16, 281–303, DOI:10.1080/09243450500114850.
- E. A. Ainsworth, Rice production in a changing climate: a meta-analysis of responses to elevated carbon dioxide and elevated ozone concentration, Global Change Biol., 2008, 14, 1642–1650, DOI:10.1111/j.1365-2486.2008.01594.x.
- C. Z. Liao, R. H. Peng, Y. Q. Luo, X. W. Zhou, X. Wu, C. M. Fang, J. K. Chen and B. Li, Altered ecosystem carbon and nitrogen cycles by plant invasion: a meta-analysis, New Phytol., 2008, 177, 706–714, DOI:10.1111/j.1469-8137.2007.02290.x.
- Y. Wu, A. Salamova and M. Venier, Using diagnostic ratios to characterize sources of polycyclic aromatic hydrocarbons in the Great Lakes atmosphere, Sci. Total Environ., 2021, 761, 143240, DOI:10.1016/j.scitotenv.2020.143240.
- M. B. Yunker, R. W. Macdonald, R. Vingarzan, R. H. Mitchell, D. Goyette and S. Sylvestre, PAHs in the Fraser River basin: a critical appraisal of PAH ratios as indicators of PAH source and composition, Org. Geochem., 2002, 33, 489–515 CrossRef CAS.
- C. Peng, W. Chen, X. Liao, M. Wang, Z. Ouyang, W. Jiao and Y. Bai, Polycyclic aromatic hydrocarbons in urban soils of Beijing: status, sources, distribution and potential risk, Environ. Pollut., 2011, 159, 802–808 CrossRef CAS PubMed.
- Y. N. Chen, J. Q. Zhang, F. Zhang, F. X. Li and M. Zhou, Polycyclic aromatic hydrocarbons in farmland soils around main reservoirs of Jilin Province, China: occurrence, sources and potential human health risk, Environ. Geochem. Health, 2018, 40, 791–802, DOI:10.1007/s10653-017-0024-5.
- J. Wang, M. L. Liu, S. C. Zhang, Y. T. Lu and H. Yao, Concentration, sources and ecological risks of PAHs of different land use types in Shenfu New City, China Environ. Sci., 2017, 38, 703–710, DOI:10.13227/j.hjkx.201607096.
- B. B. Yu, X. J. Xie, L. Q. Ma, H. D. Kan and Q. X. Zhou, Source, distribution, and health risk assessment of polycyclic aromatic hydrocarbons in urban street dust from Tianjin, China, Environ. Sci. Pollut. Res., 2014, 21, 2817–2825, DOI:10.1007/s11356-013-2190-z.
- US EPA, Superfund risk assessment, part B, Washington, D. C., available from: https://www.epa.gov/risk/superfund-risk-assessment.
- L. Rey-Salgueiro, E. Martínez-Carballo, A. Merino, J. A. Vega, M. T. Fonturbel and J. Simal-Gandara, Polycyclic aromatic hydrocarbons in soil organic horizons depending on the soil burn severity and type of ecosystem: polycyclic aromatic hydrocarbons in soil organic horizons, Land Degrad. Dev., 2018, 29, 2112–2123, DOI:10.1002/ldr.2806.
- H. I. Abdel-Shafy and M. S. M. Mansour, A review on polycyclic aromatic hydrocarbons: source, environmental impact, effect on human health and remediation, Egypt. J. Pet., 2016, 25, 107–123, DOI:10.1016/j.ejpe.2015.03.011.
- K. M. Smits, E. Kirby, W. J. Massman and L. S. Baggett, Experimental and modeling study of forest fire effect on soil thermal conductivity, Pedosphere, 2016, 26, 462–473, DOI:10.1016/s1002-0160(15)60057-1.
- G. S. Campbell, J. D. Jr Jungbauer, K. L. Bristow and R. D. Hungerford, Soil temperature and water content beneath a surface fire, Soil Sci., 1995, 159, 363–374, DOI:10.1097/00010694-199506000-00001.
-
S. Drooger, Soil temperatures under a catchment scale experimental fire, MPhil thesis, Wageningen University, Wageningen, 2009.
-
V. Andreu, J. L. Rubio and R. Cerni, Long term effects of forest fires on soil erosion and nutrient losses, in Soil Erosion and Degradation as a Consequence of Forest Fires, ed. M. Sala and J. L. Rubio, Geoforma Ediciones, Logroño, 1994, pp. 79–89 Search PubMed.
- L. F. Bano, The role of fire and soil heating on water repellency in wildland environments: a review, J. Hydrol., 2000, 231–232, 195–206, DOI:10.1016/s0022-1694(00)00194-3.
- T. McGrath, R. Sharma and M. Hajaligol, An experimental investigation into the formation of polycyclic-aromatic hydrocarbons (PAH) from pyrolysis of biomass materials, Fuel, 2001, 80, 1787–1797, DOI:10.1016/s0016-2361(01)00062-x.
- D. Nakajima, S. Nagame, H. Kuramochi, K. Sugita, S. Kageyama, T. Shiozaki, T. Takemura, F. Shiraishi and S. Goto, Polycyclic aromatic hydrocarbon generation behavior in the process of carbonization of wood, Bull. Environ. Contam. Toxicol., 2007, 79, 221–225, DOI:10.1007/s00128-007-9177-8.
- R. A. Brown, A. K. Kercher, T. H. Nguyen, D. C. Nagle and W. P. Ball, Production and characterization of synthetic wood chars for use as surrogates for natural sorbents, Org. Geochem., 2006, 37, 321–333, DOI:10.1016/j.orggeochem.2005.10.008.
- S. E. Hale, J. Lehmann, D. Rutherford, A. R. Zimmerman, R. T. Bachmann, V. Shitumbanuma, A. O'Toole, K. L. Sundqvist, H. P. H. Arp and G. Cornelissen, Quantifying the total and bioavailable polycyclic aromatic hydrocarbons and dioxins in biochars, Environ. Sci. Technol., 2012, 46, 2830–2838, DOI:10.1021/es203984k.
- P. Devi and A. K. Saroha, Effect of pyrolysis temperature on polycyclic aromatic hydrocarbons toxicity and sorption behaviour of biochars prepared by pyrolysis of paper mill effluent
treatment plant sludge, Bioresour. Technol., 2015, 192, 312–320, DOI:10.1016/j.biortech.2015.05.084.
- C. Y. Wang, Y. D. Wang and H. M. S. K. Herath, Polycyclic aromatic hydrocarbons (PAHs) in biochar – Their formation, occurrence and analysis: A review, Org. Geochem., 2017, 114, 1–11, DOI:10.1016/j.orggeochem.2017.09.001.
- A. R. Harper, C. Santín, S. H. Doerr, C. A. Froyd, D. Albini, X. L. Otero, L. Viñas and B. Pérez-Fernández, Chemical composition of wildfire ash produced in contrasting ecosystems and its toxicity to Daphnia magna, Int. J. Wildland Fire, 2019, 28, 726–737, DOI:10.1071/wf18200.
- H. W. Sun and Z. L. Zhou, Impacts of charcoal characteristics on sorption of polycyclic aromatic hydrocarbons, Chemosphere, 2008, 71, 2113–2120, DOI:10.1016/j.chemosphere.2008.01.016.
- M. Keiluweit, P. S. Nico, M. G. Johnson and M. Kleber, Dynamic molecular structure of plant biomass-derived black carbon (biochar), Environ. Sci. Technol., 2010, 44, 1247–1253, DOI:10.1021/es9031419.
- Y. Y. Qiu, Y. X. Gong and H. G. Ni, Contribution of Soil Erosion to PAHs in Surface Water in China, Sci. Total Environ., 2019, 686, 497–504, DOI:10.1016/j.scitotenv.2019.05.459.
- F. X. Kong, G. D. Sun and Z. P. Liu, Degradation of polycyclic aromatic hydrocarbons in soil mesocosms by microbial/plant bioaugmentation: performance and mechanism, Chemosphere, 2018, 198, 83–91, DOI:10.1016/j.chemosphere.2018.01.097.
- S. M. Bamforth and I. Singleton, Bioremediation of Polycyclic aromatic hydrocarbons: current knowledge and future directions, J. Chem. Technol. Biotechnol., 2005, 80, 723–736, DOI:10.1002/jctb.1276.
- L. Rey-Salgueiro, M. S. García-Falcón, B. Soto-González and J. Simal-Gándara, Procedure to measure the level of polycyclic aromatic hydrocarbons in wood ashes used as fertilizer in agroforestry soils and their transfer from ashes to water, J. Agric. Food Chem., 2004, 52, 3900–3904, DOI:10.1021/jf049760+.
- S. D. Choi, Time trends in the levels and patterns of polycyclic aromatic hydrocarbons (PAHs) in pine bark, litter, and soil after a forest fire, Sci. Total Environ., 2014, 470–471, 1441–1449, DOI:10.1016/j.scitotenv.2013.07.100.
- L. Chrysikou, P. Gemenetzis, A. Kouras, E. Manoli, E. Terzi and C. Samara, Distribution of persistent organic pollutants, polycyclic aromatic hydrocarbons and trace elements in soil and vegetation following a large scale landfill fire in northern Greece, Environ. Int., 2008, 34, 210–225, DOI:10.1016/j.envint.2007.08.007.
- L. Giglio, J. T. Randerson and G. R. van der Werf, Analysis of daily, monthly, and annual burned area using the fourth-generation global fire emissions database (GFED4), J. Geophys. Res.: Biogeosci., 2013, 118, 317–328, DOI:10.1002/jgrg.20042.
- F. J. Pérez-Porras, P. Triviño-Tarradas, C. Cima-Rodríguez, J. E. Meroño-de-Larriva, A. García-Ferrer and F. J. Mesas-Carrascosa, Machine learning methods and synthetic data generation to predict large wildfires, Sensors, 2021, 21, 3694, DOI:10.3390/s21113694.
- C. Santín, S. H. Doerr, X. L. Otero and C. J. Chafer, Quantity, composition and water contamination potential of ash produced under different wildfire severities, Environ. Res., 2015, 142, 297–308, DOI:10.1016/j.envres.2015.06.041.
- H. Z. Shen, Y. Huang, R. Wang, D. Zhu, W. Li, G. F. Shen, B. Wang, Y. Y. Zhang, Y. C. Chen, Y. Lu, H. Chen, T. C. Li, K. Sun, B. G. Li, W. X. Liu, J. F. Liu and S. Tao, Global atmospheric emissions of polycyclic aromatic hydrocarbons from 1960 to 2008 and future predictions, Environ. Sci. Technol., 2013, 47, 6415–6424, DOI:10.1021/es400857z.
- O. S. Sojinu, O. O. Sonibare and E. Y. Zeng, Concentrations of polycyclic aromatic hydrocarbons in soils of a mangrove forest affected by forest fire, Toxicol. Environ. Chem., 2011, 93, 450–461, DOI:10.1080/02772248.2010.532130.
- X. H. Li, L. L. Ma, X. F. Liu, S. Fu, H. X. Cheng and X. B. Xu, Polycyclic aromatic hydrocarbon in urban soil from Beijing, China, J. Environ. Sci., 2006, 18, 944–950, DOI:10.1021/acs.estlett.9b00010.
- J. K. Schuster, T. Harner, K. Su, A. Eng, A. Wnorowski and J. P. Charland, Temporal and spatial trends of polycyclic aromatic compounds in air across the Athabasca oil sands region reflect inputs from open pit mining and forest fires, Environ. Sci. Technol. Lett., 2019, 6, 178–183, DOI:10.1021/acs.estlett.9b00010.
- G. R. Wentworth, Y. Aklilu, M. S. Landis and Y. M. Hsu, Impacts of a large boreal wildfire on ground level atmospheric concentrations of PAHs, VOCs and ozone, Atmos. Environ., 2018, 178, 19–30, DOI:10.1016/j.atmosenv.2018.01.013.
- C. B. Ren, Q. Q. Zhang, H. W. Wang and Y. Wang, Characteristics and source apportionment of polycyclic aromatic hydrocarbons of groundwater in Hutuo River alluvial-pluvial fan, China, based on PMF model, Environ. Sci. Pollut. Res., 2021, 28, 9647–9656, DOI:10.1007/s11356-020-11485-6.
- B. X. Mai, S. H. Qi, E. Y. Zeng, Q. S. Yang, G. Zhang, J. M. Fu, G. Y. Sheng, P. A. Peng and Z. S. Wang, Distribution of polycyclic aromatic hydrocarbons in the coastal region off Macao, China:assessment of input sources and transport pathways using compositional analysis, Environ. Sci. Technol., 2003, 37, 4855–4863, DOI:10.1021/es034514k.
Footnote |
† Electronic supplementary information (ESI) available. See DOI: 10.1039/d1em00377a |
|
This journal is © The Royal Society of Chemistry 2022 |