DOI:
10.1039/D2CS00490A
(Tutorial Review)
Chem. Soc. Rev., 2022,
51, 9861-9881
Indium arsenide quantum dots: an alternative to lead-based infrared emitting nanomaterials
Received
11th August 2022
First published on 21st November 2022
Abstract
Colloidal quantum dots (QDs) emitting in the infrared (IR) are promising building blocks for numerous photonic, optoelectronic and biomedical applications owing to their low-cost solution-processability and tunable emission. Among them, lead- and mercury-based QDs are currently the most developed materials. Yet, due to toxicity issues, the scientific community is focusing on safer alternatives. In this regard, indium arsenide (InAs) QDs are one of the best candidates as they can absorb and emit light in the whole near infrared spectral range and they are RoHS-compliant, with recent trends suggesting that there is a renewed interest in this class of materials. This review focuses on colloidal InAs QDs and aims to provide an up-to-date overview spanning from their synthesis and surface chemistry to post-synthesis modifications. We provide a comprehensive overview from initial synthetic methods to the most recent developments on the ability to control the size, size distribution, electronic properties and carrier dynamics. Then, we describe doping and alloying strategies applied to InAs QDs as well as InAs based heterostructures. Furthermore, we present the state-of-the-art applications of InAs QDs, with a particular focus on bioimaging and field effect transistors. Finally, we discuss open challenges and future perspectives.
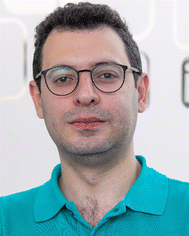
Houman Bahmani Jalali
| Houman Bahmani Jalali is a Researcher at the Istituto Italiano di Tecnologia (IIT), Genoa (Italy). He graduated in Nano Science & Nano Engineering at Istanbul Technical University (Istanbul, Turkey) in 2015 and then obtained his Ph.D. degree in Biomedical Sciences and Engineering at Koç University (Istanbul, Turkey) in 2020. His Ph.D. thesis titled “Novel Biocompatible Quantum Dots and Nanoengineered Assemblies for Optoelectronic Neural Interfaces” was awarded the “Best Doctoral Thesis of 2020” by “The Council of Higher Education” of Turkey. He joined IIT at the end of 2020 where he was awarded a Marie Skłodowska-Curie Individual Fellowship to work on “Fully RoHS-compliant Infrared Light Emitting Diodes Based on Novel Lead-free Quantum Dots”. His research focuses on “green” and RoHS-compliant quantum dots, in particular III–V systems for optoelectronic and bioelectronic applications. |
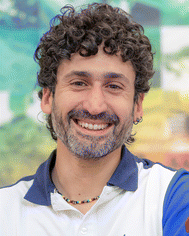
Luca De Trizio
| Dr Luca De Trizio is a Technologist at the Istituto Italiano di Tecnologia, Genova (Italy). He graduated in Materials Science in 2008 at the University of Milano Bicocca (Italy) and obtained his PhD in Nanostructures and Nanotechnology from the same institution in 2013. During his PhD, in 2010–2011, he worked as fellow at IIT and, in 2012, at the Molecular Foundry of the Lawrence Berkeley National Lab. From 2013 to 2019, he worked as a postdoc researcher at the Nanochemistry Department at IIT. Since 2020 he has been one of the Deputy Directors for Student Mentorship at the Open University Affiliated Research Centre at IIT. His research interests include the colloidal synthesis of transparent conductive oxides, plasmonic materials, luminescent semiconductors, electrocatalytic materials their characterization and the study of post-synthetic chemical transformations of nanocrystals. |
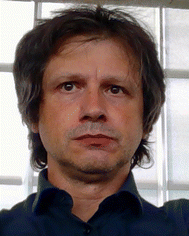
Liberato Manna
| Liberato Manna received his PhD in Chemistry in 2001 from the University of Bari (Italy) and worked at UC Berkeley (USA) as a visiting Student and subsequently at the Lawrence Berkeley Lab (USA) as a postdoctoral fellow until 2003. He was then scientist at the National Nanotechnology Lab in Lecce (Italy) and he moved to the Istituto Italiano di Tecnologia (IIT), Genova (Italy) in 2009 as head of the Nanochemistry Group. From 2010 to 2021 he has also been part-time professor at TU Delft (The Netherlands). Currently, he is also associate director of IIT for the Materials and Nanotechnology programs at IIT. His research interests include the synthesis and assembly of colloidal nanocrystals, the study of structural, chemical and surface transformations in nanoscale materials, and their applications in energy, photonics, and electronics. |
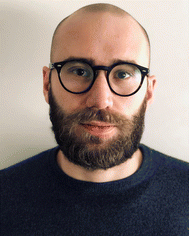
Francesco Di Stasio
| Francesco Di Stasio graduated in Materials Science and Engineering at the University of Genoa in 2008 (Italy), then he obtained a PhD in Physics at University College London (UK) in 2012. He worked as a research Scientist at Cambridge Display Technology (Sumitomo Chemical group, UK) until he undertook postdoctoral research at the Istituto Italiano di Tecnologia (IIT, Italy). In 2015 he was awarded a Marie Skłodowska-Curie Individual Fellowship at the Institute of Photonic Sciences (ICFO, Spain). Since 2020, he is Principal Investigator of the Photonic Nanomaterials group at IIT after being awarded e European Research Council Starting grant. His research focuses on the development of novel optoelectronic devices based on colloidal nanocrystals; in particular, electrically driven light-sources such as light-emitting diodes, single-photon emitters, and lasers. |
Key learning points
(1) History and evolution of the colloidal synthesis of indium arsenide QDs.
(2) Recent advances in chemical strategies for synthesis improvement of indium arsenide QDs.
(3) Understanding of the surface chemistry and trap passivation of indium arsenide QDs.
(4) Recent biomedical, electronic and optoelectronic applications of indium arsenide QDs.
(5) Perspectives and challenges of the colloidal indium arsenide QDs in the future.
|
Introduction
Colloidal quantum dots (QDs) have already demonstrated their applicability in consumer electronic products operating in the visible spectral range.1,2 Yet, significant developments are still required for QDs to become applicable in the next generation of infrared (IR) technologies due to the challenges of both synthesis and optical characterization in that region of the spectrum.3,4 Also, there are much fewer available QDs active in the IR range compared to semiconductor QDs active in the visible range. Among them, the most studied ones are lead- and mercury-based QDs in terms of synthesis development,5,6 compositional modulation,7 band-gap engineering,8–10 and surface passivation.11–14 These “classical” IR QDs can now be easily synthesized and surface functionalized to achieve a strong and narrow photoluminescence (PL) emission. However, their use in the next-generation optoelectronic applications is severely restricted by their inherent toxicity.15
In this context, a promising alternative IR emitter is indium arsenide (InAs), a III–V semiconductor with a narrow bulk bandgap of 0.35 eV at room temperature (0.40 eV at 77 K),16 a zinc blende crystal structure (lattice constant of 6.05 Å)16 InAs is characterized by In–As bonds that are more covalent than those of II–VI semiconductors (i.e. the Philips ionicity of InAs is 0.3617 while those of CdSe and PbS are 0.7017 and 0.77,17 respectively). The high covalency of the In–As bond has several consequences on the physical properties of this material.17 First, in a covalent material such as InAs, carriers are more efficiently screened than in more ionic materials, and this translates into a large exciton Bohr radius for InAs (the size of which is still “debated” and has been reported to be ∼45 nm18 or ∼31 nm19). This means that a size dependence in the optical properties of InAs QDs is also found in relatively large crystals, a feature which provides a handle for tuning such properties across a broad size range, deep in the near infrared (NIR). Also, covalent bonds are less susceptible to be ruptured by polar molecules, such as water, compared to more ionic bonds, thus leading to an overall improved chemical stability,17,20,21 especially with respect to leaching of As species in aqueous environments compared for example to the more ionic Cd, Pb and Hg based semiconductors. In semiconductors, the presence of surface states is very detrimental for the optical properties due to unpassivated surface dangling bonds, as photogenerated carriers are easily trapped in them. The depth of these trap states, starting from relatively shallow levels in strongly ionic semiconductors, increases with the increasing covalency of the bond.17 So, it is critical for III–V QD systems to have their surface well passivated, for example by an epitaxial shell growth of a larger band gap material. It is also worth reminding that InAs is the only “Restriction of Hazardous Substances” (RoHS) compliant semiconductor22 having tunable optical absorption and emission in the whole near infrared (NIR) spectral range (Fig. 1). Yet, despite the comparatively lower toxicity of InAs compared to Pb- and Cd-based materials,23 the leaching of inherent-toxic indium24 and arsenic25 elements can still lead to health and environmental risks, so effective surface passivation approaches are needed also to tackle this issue.26
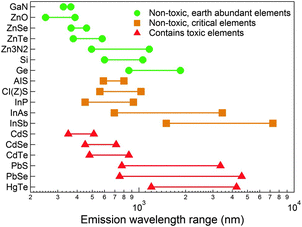 |
| Fig. 1 Emission wavelength range of different semiconductors in the visible and IR spectral windows. InAs is the only RoHS-compliant semiconductor that can emit in the full range of the NIR. InAs is considered critical due to the limited supply of indium element. Reprinted with permission from ref. 64, Copyright 2015, The Royal Society of Chemistry. | |
The optical characteristic of InAs QDs makes them promising candidates for efficient photovoltaic heterojunctions,27 quantum light emitting diodes28 and biological imaging.29,30 They have an absorption cross-section per dot at excitonic peak around 3.15 × 10−16 R1.28 cm2, estimated by Yu et al.,31 corresponding to a reduced radius-dependency than PbS QDs (7.494 × 10−17 R2.31 cm2).32 On the other hand, InAs QDs are suitable for optical communication33 and deep tissue bioimaging34 since they can emit in the range of 1300–1550 nm, where the transmission losses are minimized. Moreover, InAs QDs feature n-type conductivity in ambient atmosphere due to their electron donating surface states,27,35–38 unlike lead chalcogenides that switch from n-type to p-type upon air exposure.35,39–41 A NIR absorbing QD with n-type characteristic can be coupled to various p-type light-harvesting materials and used for efficient heterojunction solar cells.27
Despite the important role that InAs QDs could play in such applications, currently their in-device performance is limited due to their poor optical properties.3,4 The PL linewidth and PL quantum yield (PLQY) of InAs-based QDs indeed lag far behind those of the “state-of-the-art” lead sulfide (PbS)7,9,42–45 and lead selenide (PbSe)45,46 core/shell QDs.47 The reason is the more challenging synthesis of InAs compared to that of II–VI (CdSe,48–51 CdTe49,52 and CdS49,53) and IV–VI QDs (PbS,54–57 PbSe39 and PbTe58), for which a wide range of precursors, with a very tunable reactivity, is available to control both the size and size distribution, even at low temperatures.17,21 Moreover, the few available arsenic precursors are very reactive, making it hard to have a good control over the nucleation and growth of the nanocrystals (NCs).21,59,60 Consequently, broad size distributions are usually obtained, resulting in broad absorption and PL peaks. Furthermore, the temperature required to grow InAs QDs with good crystallinity (>240 °C) is higher than that of II–VI HgTe (<150 °C)6 and II–VI PbS (<210 °C)61 QDs due to the more covalent nature of the In–As bonds. To obtain efficient emitters, the surface of InAs QDs should be overcoated with a shell of an inorganic material (forming core/shell heterostructures) capable of yielding a Type-I band alignment (see “Surface chemistry and trap passivation” section for more details). Unfortunately, InAs has a large lattice parameter (6.06 Å)20 compared to that of many Cd-free wide bandgap materials such as ZnSe (5.66 Å),62 ZnS (5.4262 Å) and ZnO (5.20 Å).63 Hence, the overgrowth of a shell of these materials results in heterostructures (e.g. InAs/ZnS or InAs/ZnSe) that are highly strained and that typically feature a low PLQY due to the presence of interfacial defects. Therefore, any significant advancement in the synthesis of InAs QDs capable of improving these figures of merit will have a strong technological impact on various areas of IR technology.
In this tutorial review, we summarize the recent progress on colloidal InAs QDs. The review is structured in four main sections: (I) synthesis methods. These are discussed in detail, highlighting their strengths and weaknesses; (II) InAs surface chemistry, InAs based core/shell systems and their optical characteristics; (III) electronic properties and carrier dynamics of InAs QDs; (IV) applications of InAs QDs in bioimaging, field effect transistors (FETs), NIR light emitting diodes (LEDs) and photovoltaics. Finally, we provide an outlook on the future research directions in this field.
Synthesis of colloidal InAs QDs
Syntheses based on tris(trimethylsilyl)arsine (TMS-As) precursor, and analogues
The first wet-chemical synthesis route to InAs was reported by Wells et al. in 1989, and consisted of a dehalosilylation reaction (eqn (1)).65 Such dehalosilylation route represented a milestone for the synthesis of III–V semiconductors, as it opened up a solution phase chemical route. Indeed, prior to that, III–V compounds were synthesized via organometallic chemical vapor deposition (OMCVD)66 and molecular beam epitaxy (MBE)67,68 only, as no wet chemical routes had yet been developed. In that work, Wells et al. stirred a solution of indium chloride (InCl3) and tris(trimethylsilyl)arsine (TMS-As) in pentane at room temperature for 3 days, after which the solution was kept at 70–75 °C for 4 days. Such reaction releases directly As3− monomers which react with In3+ cations (to form InAs), with the concomitant formation of Me3SiCl (Me stands for methyl), a low boiling compound.65 Finally, the solution was heated to 150 °C overnight to remove the volatile Me3SiCl byproduct. The final product was InAs with 98% purity. The same group later effectively eliminated the Me3SiCl byproduct through an annealing step at higher temperature (400 °C) and obtained 99.65% pure (albeit non-emissive) nanocrystalline InAs.69 | Me3SiCl–As + InCl3 (75 °C) → InAs + 3 Me3SiCl | (1) |
Few years later, in 1996, Guzelian et al. used trioctylphosphine (TOP) as both solvent and capping agent and performed the same heat-up strategy at higher temperature (240–265 °C) than the 75 °C of the work of Wells et al.70 Upon size selection of the products, they could isolate InAs QDs with size-tunable band-edge emission in the 850–1200 nm range and demonstrated the presence of quantum confinement in InAs QDs for the first time (Fig. 2a). Further improvements in the synthesis of InAs QDs were made possible by employing the so-called hot-injection method: Peng et al. and Battaglia et al. in 1998 injected TMS-As into a hot solution of indium acetate [In(Ac)3], carboxylic acids (i.e. palmitic acid and myristic acid) and 1-octadecene (1-ODE) to obtain InAs QDs.71,72 This approach became the standard way to produce InAs QDs and it has since then been further optimized.29,73,74
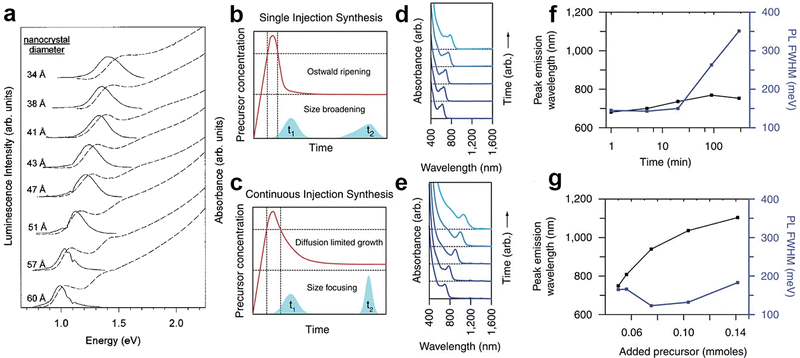 |
| Fig. 2
Synthesis of colloidal InAs QDs via TMS-As, and analogues. (a) The first size-tunable InAs QDs synthesized via the hot-injection technique using TOP as both solvent and capping agent. Reprinted with permission from ref. 70, Copyright 1996, AIP Publishing. Growth mechanism (b and c) of InAs QD synthesized via typical single hot-injection and continuous hot-injection techniques. Absorbance (d and e), PL tunability and PL width (f and g) of InAs QD synthesized via (d and f) a typical single hot-injection and (e and g) continuous hot-injection techniques. Reprinted with permission from ref. 30, Copyright 2016, Springer Nature. | |
Size and size distribution control of InAs QDs synthesized via TMS-As, and analogues.
Tuning the size and simultaneously achieving a narrow size distribution is an important challenge for the colloidal synthesis of III–V QDs.2,21,75 The size distribution of the QDs is correlated to the width of their first exciton absorption peak, normally measured as half width at half maximum (HWHM). Since the first colloidal synthesis of InAs QDs by the Wells’ dehalosilylation reaction,65 the community has explored the reactions between InX3 (where X = halide or carboxylate) and TMS-As seeking to improve the size distribution of InAs QDs.30,71,76,77 The problem with TMS-As is its high reactivity (i.e. high conversion rate) that leads to a fast nucleation of InAs QDs, hence a fast depletion of monomers, which are then not available for the growth step. As a consequence, InAs QDs tend to grow in the Ostwald ripening regime (i.e. shrinking and dissolution of small particles, with the released monomers that then feed larger particles, a process that quickly leads to a broadening of the size distribution).21,59,72,78 Efforts to tune the precursors conversion kinetics to achieve temporal separation between the nucleation and the growth stages have only been moderately successful in the case of InAs QDs. In this regard, Harris et al. and Franke et al. investigated the effect of using TMS-As analogues, namely tris(isopropyldimethylsilyl) arsine76 and tris(trimethylgermyl)arsine (TMGe-As)60 in the synthesis of InAs QDs. Their results unexpectedly showed that changes in precursor reactivity, even up to 2–3 orders of magnitude, have negligible effects on the growth kinetics and size broadening of the InAs QDs.76 In another study, Franke et al. obtained large InAs QDs with relatively narrow size distributions (PL linewidth <135 meV) by prolonging the size-focusing regime using a multiple injection approach (Fig. 2b and c).30 In details, they first nucleated InAs QDs by employing a sub stoichiometric amount of As precursor (As/In ratio of 10%, using TMGe-As and indium(III) acetate) and then grew the formed nuclei by the addition of the residual amount of arsenic precursor, using a syringe pump. This strategy led to size tunable InAs QDs with PL peak ranging from 700 nm to 1200 nm without any size-selective purification step (Fig. 2d–g).
Syntheses based on trisdimethylamino arsine (amino-As) precursor
Since TMS-As and analogues, as well as arsine (AsH3),79,80 are highly pyrophoric, reactive, hazardous and relatively expensive,72,81 a few alternative arsenide precursors, including triphenylarsine (AsPh3),82 arsenic silylamide ([(Me3Si)2N]2AsCl)83 and [tBu2AsInEt2]284 have been explored. However, they all led to polydisperse InAs QDs with low PLQY. On the other hand, Green et al. used a safe, cheap and commercially available arsenic precursor, namely tris(dimethylamino)arsine (amino-As) for the first time.85 They were able to synthesize InAs QDs by thermolysis of InCl3 and amino-As in 4-ethylpyridine at 167 °C for up to 6 days. Griegel et al. improved then this technique by combining amino-As as the arsenic precursor with tris(dimethylamino)phosphine (amino-P) as a reducing agent for the synthesis of InAs QDs (the reaction was carried out at 190 °C for 30 min).86 The synthesis consists of two step: (i) the addition of amino-As to a mixture of indium and zinc halides dissolved in oleylamine (OAm), which generates dimethylamine and tris(oleylamino)arsine, As(NHOl)3, via a transamination reaction (eqn (2); (ii) the addition of a reducing agent, amino-P in this specific case, such that phosphorous is oxidized from +3 to +5, to trigger the nucleation of InAs QDs (eqn (3). This second step is crucial, as As(+III) species have to be reduced to As(−III) (see the below paragraph for more details). The overall synthesis scheme is the following: | Amino-As + 3OlNH2 → As(NHOl)3 + 3Me2NH | (2) |
| InCl3 + As(NHOl)3 + P3+ + (NHOl)3 → InAs + 3P5+ + (NHOl)4 Cl | (3) |
Role of reducing agents in the synthesis of InAs with amino-As precursor.
The addition of the reducing agent is of paramount importance for the synthesis of InAs QDs with amino-As, since this compound is not able to undergo disproportionation, unlike what observed for amino-P [i.e. 4 P(+III) → P(−III) + 3 P(+V)].86–89 In the synthesis of InAs QDs by amino-As, the reducing agent governs the release of As3− monomers and therefore the nucleation and growth kinetics. In this regard, Srivastava et al. replaced amino-P with diisobutylaluminum hydride (DIBAL-H) (Fig. 3a) and synthesized size tunable InAs QDs with a broad excitonic absorption peak in the range of 750–1450 nm (Fig. 3c and d).89 To optimize the synthesis kinetics, the same group tested other types of reducing agents, namely amino-P, Alane N,N dimethylethylamine complex (DMEA-AlH3), DIBAL-H and lithium triethylborohydride (LiEt3BH), and monitored the corresponding InAs products.90 According to their study, the precursors conversion rate could be controlled by tuning the reducing strength of the reducing agent and so the size and size distribution of the InAs QDs (Fig. 3e–g).90 In details, LiEt3BH (the strongest reducing agent tested) led to the formation of undesired metallic indium particles together with large InAs crystallites.90–92 Conversely, amino-P (the weakest reducing agent tested) delivered InAs QDs with a broad size distribution (HWHM of 170 meV), as evidenced by the almost featureless absorption peak of the resulting product.90 On the other hand, DMEA-AlH3, which features a slightly higher reducing power than DIBAL-H, was found to ensure the best control over the size distribution of InAs QDs, with HWHM as low as 120 meV90 (Fig. 3g). Furthermore, it was observed that amino-P could be activated only at elevated temperatures (240–280 °C), while DIBAL-H or DMEA-AlH3 can form amorphous molecular intermediates (also called InAs clusters) even at room temperature.90 The list of all precursors used for the synthesis of InAs QDs via hot-injection is summarized in Table 1.
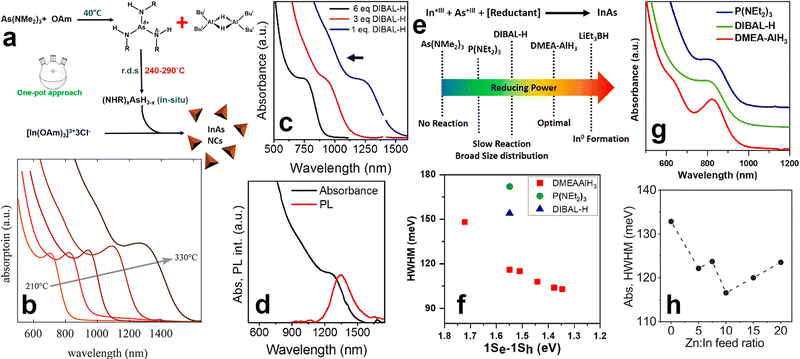 |
| Fig. 3
Synthesis of colloidal InAs QDs via amino-As. (a) The mechanism regulating the formation of InAs QDs using DBAL-H as reducing agent. Reprinted with permission from ref. 89, Copyright 2016, American Chemical Society. (b) Excitonic absorption peaks versus reaction temperature of the colloidal InAs QDs synthesized via indium(I) chloride as both indium source and reducing agent. Reprinted with permission from ref. 99, Copyright 2020, American Chemical Society. (c) Optical absorption spectra of InAs QDs grown at 240 °C for 5 min with different concentrations of DIBAL-H. (d) Optical absorption and PL spectrum of ∼5 nm InAs QDs. (e) Schematic representation of the reducing power of different reducing agents used for the synthesis of InAs QDs via amino-As. Reprinted with permission from ref. 89, Copyright 2016, American Chemical Society. (f) HWHM and (g) absorption spectra of InAs QDs synthesized using different reducing agents. Reprinted with permission from ref. 90, Copyright 2018, American Chemical Society. (h) HWHM of InAs QDs synthesized by different Zn : In feed ratios. Reprinted with permission from ref. 100, Copyright 2022, American Chemical Society. | |
Table 1 List of chemicals used for the synthesis of InAs QDs via the hot-injection method. In(Ac)3: indium(III) acetate, InCl3: indium(III) chloride, InCl: indium(I) chloride, TMS-As: tris(trimethylsilyl)arsine, amino-As: trisdimethylamino arsine, TMGe-As: tris(trimethylgermyl)arsine, AsCl3: antimony chloride, As(OLA)3: trioleylarsane, DIBAL-H: diisobutylaluminum hydride, DMEA-AlH3: Alane N,N dimethylethylamine complex, LiEt3BH: lithium triethylborohydride, MA: myristic acid, PA: palmitic acid, OA: oleic acid, TOP: tri-n-octyl phosphine, DDPA: dodecylphosphonic acid, OAm: oleylamine, 1-ODE: 1-octadecene (pyrophoric items are marked by *)
Indium source |
Arsenide source |
Reducing agent |
Ligand |
Solvent |
Ref. |
In(Ac)3 |
As(TMS)3* |
— |
PA, MA, TOP |
1-ODE |
71 and 76
|
In(Ac)3 |
As(TMS)3* |
— |
TOP, LA |
1-ODE |
93
|
In(Ac)3 |
As(TMS)3* |
— |
OA |
1-ODE |
94 and 95
|
In(Ac)3 |
As (iPrDMSi)3* |
— |
MA, TOP |
1-ODE |
76
|
In(Ac)3 |
As(TMGe)3* |
— |
OA, TOP |
1-ODE |
30 and 76
|
In(Ac)3 |
As(TEGe)3* |
— |
MA, TOP |
1-ODE |
76
|
In(Ac)3 |
AsCl3 |
LiEt3BH* |
OAm |
OAm |
96
|
InCl3 |
Amino-As |
Amino-P |
OAm |
OAm |
86 and 97
|
InCl3 |
Amino-As |
DIBAL-H* |
OAm |
OAm |
89
|
InCl3 |
Amino-As |
DMEA-AlH3* |
OAm |
1-ODE |
90
|
InCl3 |
Amino-As |
LiEt3BH* |
OAm |
1-ODE |
90
|
InCl3 |
As(OLA)3 |
DIBAL-H* |
OAm |
OAm |
98
|
InCl |
Amino-As |
InCl |
TOP, DDPA |
OAm |
99
|
In(Ac)3 |
Amino-As |
DMEA-AlH3* |
OAm + ZnCl2 |
OAm |
100
|
InCl3 |
Amino-As |
Amino-P |
OAm |
OAm |
97 and 101
|
Since most of the reducing agents employed for the synthesis of InAs QDs are hazardous and/or pyrophoric (e.g. DMEA-AlH3, LiEt3BH and DIBAL-H), recently Ginterseder et al. explored the use of indium(I) chloride as both the indium source and reducing agent.99 This chemical has a limited toxicity and has been already used in synthetic organic chemistry as an alternative to highly reactive hydride sources.102–104 The authors hypothesized that the oxidation of In1+ to In3+ provides electrons for the reduction of As3+ to As3− and the consequent formation of In–As species. By this method, it is possible to synthesize gram scale products and tune the absorption peak of the InAs QDs in the 700–1400 nm range (by varying the reaction temperature) (Fig. 3b).
Seeded growth approach for the synthesis of larger InAs QDs
In the TMS-As based strategy, the control over the size distribution of InAs QDs can be achieved only on small QDs, with emission wavelengths shorter than ∼900 nm, since TMS-As triggers a fast nucleation of InAs QDs. In this synthesis scheme, an almost complete depletion of monomers takes place at the early stages of the synthesis, thus preventing the further QDs growth in the size focusing regime. In the amino-As route, it is possible to modulate the reactivity of the As precursor by carefully adjusting parameters such as the reducing power of the reducing agent and the temperature. With the aim of synthesizing large QDs, Leeman et al. lowered the injection temperature of amino-As to 170 °C (compared to 270 °C previously employed by the same group97) and increased the volume of the solvent (i.e. to reduce the concentration of monomers in order to achieve a larger nucleation radius)105 eventually obtaining larger nuclei and consequently larger InAs QDs.101 The resulting InAs QDs exhibited a band-edge absorption tunable from 1140 nm to 1400 nm with relatively broad HWHM (∼150 meV).
While the one-pot synthesis approach to produce large InAs QDs with narrow size distribution still requires further developments, other approaches have shown interesting results. In this regard, the so-called “seeded-growth” strategy, performed either with TMS-As or with amino-As precursors, can be used to grow large InAs QDs with good control over the size distribution. In this method, precursors are added to a dispersion of pre-formed QDs (also called seeds) to promote their growth while avoiding the nucleation of new crystals, so that the growth rate of the seeds can be controlled by tuning the addition rate of the precursors.2,106,107 These precursors are clusters bringing the advantage of containing both In3+ and As3− species that are readily available to react with the growing seeds. These clusters can be produced with either TMS-As (reacting the latter with indium oleate at room temperature,108,109Fig. 4b) or amino-As (via its reaction at room temperature with both InCl3 and reducing agents such as DIBAL-H or DMEA-AlH3). For example, Tamang et al. synthesized 2.5 nm sized InAs seeds (Fig. 4c) via TMS-As that exhibited an excitonic peak at 1.77 eV and HWHM larger than 100 meV (Fig. 4b). Then, they grew these seeds via the addition of InAs clusters at various temperatures (270–300 °C) and at different injection rates (using a syringe pump, Fig. 4a) and obtained InAs QDs with a rather narrow size distribution (HWHM <80 meV, Fig. 4e), in the size range of ∼3 to 6 nm (Fig. 4d). In another study, Kim et al. optimized the QDs growth in the size focusing regime and synthesized 9 nm sized InAs QDs with an even narrower size distribution (HWHM of 60.5 meV) having excitonic peak at 1600 nm (Fig. 4f) by carefully engineering the injection rate of InAs clusters.109
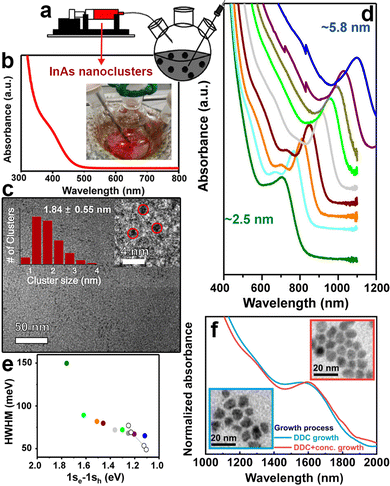 |
| Fig. 4
Synthesis of large InAs QDs via the seeded growth method. (a) Slow and continuous injection of amorphous InAs nanoclusters into InAs QD seeds using a syringe pump. (b) Absorption spectra and (c) TEM image of small amorphous InAs nanoclusters. (d) Absorption spectra of differently sized InAs QDs synthesized via the controlled injection of amorphous nanocluster solution (at 0.005 mmol min−1, temperature 270 °C) (e) HWHM of the first excitonic peak of InAs QDs synthesized by adding 0.05 to 3 mmol (filled circles) of precursor solutions compared with the initial seed solution (green). Empty circles represented literature values for high-quality PbS QDs for comparison. Reprinted with permission from ref. 108, Copyright 2016, American Chemical Society (f) Absorption spectra of InAs QDs grown using diffusion-dynamics-control (DDC) for 9 h, with excitonic peaks at 1579 (blue line) and optimized DDC with increased precursor concentration for 10h, with excitonic peak at 1600 nm (red line), respectively (inset: TEM images of these InAs QDs). Reprinted with permission from ref. 109, Copyright 2021, Springer Nature. | |
Shape control of InAs QDs
Tuning the shape of QDs represents an effective way to tailor their chemical, physical, and optical properties.2 A practical way to manipulate the shape of colloidal QDs relies on the use of specific surfactants. In details, the several facets that a QD can expose are characterized by different surface energies and chemical behaviours (i.e. different types and geometric arrangements of atoms and of broken bonds), often related to anisotropies in the crystal structure.105,110 The higher affinity of a specific surfactant to some facets than others can affect the corresponding growth rates, leading to QDs featuring non-symmetric shapes, as extensively reported for CdSe111–113 and InP114 QDs. In the case of InAs QDs, Trentler et al. synthesized nanorods(NRs)/nanowires(NWs) for the first time by employing thiophenol.92 In other works, metal nanoparticles of either indium,115 gold,110,115 bismuth116–118 or silver115 have been used to promote the nucleation and growth of anisotropic InAs nanostructures. For example, Kan et al. synthesized InAs NRs by injecting dodecanethiol-stabilized gold nanoparticles with diameter of ∼2 nm into a solution of InCl3, TMS-As and TOPO at high temperature (360 °C).110 The resulting InAs NRs had a diameter of 4.1 nm and variable lengths (ranging from 9.4 nm to 22.4 nm) the latter varying as a function of the added gold concentration. The InAs NRs exhibited length-dependent optical properties and a reduction in the PL intensity and a red-shift of the bandgap (up to 120 meV) were seen by increasing the rod length.110 In InAs NRs, the length is governed by a strongly quantum-confined regime, unlike CdSe NRs in which a medium to weak confinement regime is observed, possibly due to the larger bulk exciton Bohr radius of InAs (35 nm) compared to that of CdSe (5 nm).110
Synthesis of InAs-based alloyed/doped QDs
Features of QDs such as band gap and PLQY are strongly dependent on the QDs’ size. However, they are also dependent on the chemical composition, and as such they can be readily tuned by synthesizing alloyed QDs.2,119–121 In the specific case of InAs, the incorporation of 3+ cations (Ga),122 or 3− anions (Sb123 or P86,97,124) leads to alloyed InAs QDs with compositionally tunable optical features. For example, Kim et al. synthesized InAsxP1−x alloyed QDs (by using TMS-As and TMS-P) that had a radial graded composition, with increasing arsenic content from the center to the edge of the dots. They were able to tune the emission of the QDs from 600 nm to 800 nm by changing the As-to-P ratio while keeping the size almost unchanged (Fig. 5a).124 In another study, Kim et al. synthesized InAsxSb1−x QDs by the hot injection of TMS-As and TMS-Sb and observed a red-shift of the excitonic feature from ∼700 nm to ∼850 nm by increasing the Sb content, since bulk InSb has narrower band gap than InAs (Fig. 5b).123 However, the PL width increased from 84 nm to 164 nm when going from InAs to InAs0.86Sb0.14, indicating a poor control over the size distribution while employing the two pnictide sources together (Fig. 5c). For the case of In1−xGaxAs alloyed QD, Park et al. synthesized InAsxGa1−x by the hot-injection of TMS-As and tuned the emission of InAs from 700 nm to 580 nm (Fig. 5d), with the In0.2Ga0.8As/ZnSe featuring a PLQY of 25.6%.122
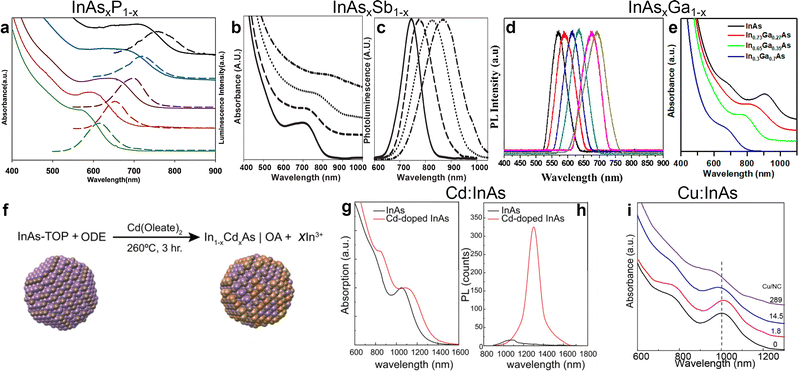 |
| Fig. 5
Synthesis of alloyed/doped InAs QDs. (a) Absorbance (solid line) and PL (dashed line) of InP (green), InAs0.33P0.66 (red), InAs0.66P0.33 (brown), InAs0.82P0.18 (blue), and InAs (black), from bottom to top, respectively. Reprinted with permission from ref. 124, Copyright 2005, American Chemical Society. (b) Absorbance and (c) PL of InAs (solid line), InAs0.97Sb0.03 (dashed line), InAs0.90Sb0.10 (dotted line), InAs0.86Sb0.14 (dash-dotted line). Reprinted with permission from ref. 123, Copyright 2006, The Royal Society of Chemistry. (d) Normalized PL of various In1−xGaxAs/ZnSe QDs; (right to left: in content 5%−30%). Reprinted with permission from ref. 122, Copyright 2016, American Chemical Society. (e) Absorption spectra of InAs (black), In0.73AsGa0.27 (red), In0.65AsGa0.35 (green) and In0.3AsGa0.7 (blue) QDs. Reprinted with permission from ref. 20, Copyright 2018, American Chemical Society. (f) Scheme of Cd doping of InAs synthesized via TMS-As. (g) Absorption and (h) PL of undoped (black lines) and Cd-doped (red lines) InAs QDs. Reprinted with permission from ref. 36, Copyright 2020, John Wiley and Sons. (i) Absorption spectra of undoped and Cu doped InAs QDs. Reprinted with permission from ref. 129, Copyright 2019, American Chemical Society. | |
Another toolkit for preparing alloyed QDs is represented by cation exchange reactions, in which cations of pre-formed colloidal NCs are partially substituted with new cations, while retaining the size and shape of the QDs.125 This strategy has been largely employed in various II–VI, I–VI, IV−VI and even III–V systems to get either alloyed QDs or even heterostructures, including core/shell systems.2,126,127 In the case of InAs QDs, cation exchange reactions have been successfully exploited by Srivastava et al. who exchanged In3+ with Ga3+ cations in preformed InAs QDs, thus forming ternary In–Ga–As QDs with tunable bandgap.20 In details, the absorption of InAsxGa1−x QDs could be tuned from ∼950 nm to ∼750 nm by increasing the Ga content (Fig. 5e).20 Interestingly, the cation exchange reaction was performed in molten salts (namely, a mixture of CsBr:LiBr:KBr), a reaction environment that allows to work at relatively high temperatures (380–500 °C), at which traditional organic solvents and surface ligands would either evaporate or decompose.128
In terms of doping, Asor et al. doped the surface of InAs with cadmium by the dropwise addition of Cd(oleate)2 to a preheated solution of InAs QDs in 1-ODE and OAm at 260 °C under inert atmosphere (Fig. 5f).36 Their results indicated that cadmium doping not only increases the chemical stability of InAs QDs against oxidation under ambient atmosphere, but also transforms them from n-type to p-type (see “Applications” section for more details). As regarding the optical properties, cadmium doping led to the broadening and red-shifting (by 95 meV) of the excitonic absorption peak (Fig. 5g), as well as a red-shifting (by 190 meV) the PL peak and increase the PL intensity by an order of magnitude (Fig. 5h). In another study, Tripathi et al. doped InAs QDs with copper via the reaction of copper chloride (CuCl2) at room temperature.129 The copper doping led to an improvement in the carrier mobility (see “Applications” section for more details)129 and a redshift of the absorption peak (however less marked than in the case of cadmium, see Fig. 5i). This type of diffusion-based doping allows direct comparison of the electronic properties upon introducing different dopants (e.g. Cu,130 Au131 and Ag37) to InAs QDs.
Surface chemistry and trap passivation of InAs QDs
The large surface-to-volume ratio of QDs typically plays a detrimental role in the recombination of photoexcited carriers since surface uncoordinated atoms act as non-radiative recombination sites (i.e. traps).132 Both surface states and surface ligands play a crucial role in determining the final electronic and optical properties of InAs QDs.100 While the surface chemistry of “classical” II–VI and IV–VI QD systems has been studied extensively, that of InAs QDs is still under investigation. As the very first study in this direction, Leemans et al. examined the surface chemistry of InAs QDs synthesized with amino-As (and amino-P as the reducing agent).97 The InAs QDs analyzed in their work had a truncated tetrahedron shape (Fig. 6b) terminated by In(111)-facets in which In3+ atoms are passivated by Cl− ions (X-type ligand) and OAm (L-type ligands) (Fig. 6a).97 In another study, the same group replaced the native, long-chain OAm ligands by a combination of short-chain stabilizers such as n-butylamine and 3-mercapto-1,2-propanediol to enhance the inter-dots charge carrier mobility.101 Recently, Zhu et al. revealed that most of the InAs trap states originate from surface indium vacancies. These can be partially passivated by zinc chloride (ZnCl2) acting as Z-type ligands (Fig. 6c) and leading to a slight improvement in the PLQY from below 1% to 2% (Fig. 6d).100 In another study, Kim et al. effectively passivated indium vacancies via fluoride ions using hydrofluoric acid (HF) (Fig. 6e).18 Surface fluorination of InAs cores red-shifts the PL (250 meV) and boosts the PLQY from 1.5% to 11% (Fig. 6f). They justified this significant PL red-shift by the InAs large exciton Bohr radius, which enables excitons to spread over a large volume, thus indicating that the optical behavior of InAs QDs is strongly susceptible to changes in the electronic structure of the surface. In addition, the electron effective mass of bulk InAs (0.024 m0133) is significantly smaller than that of other classical semiconductors such as CdSe (0.11 m0133) and InP (0.08 m0134). This entails a decreased confinement of the electron wavefunction in the InAs core region and a decrease in radiative recombination.133
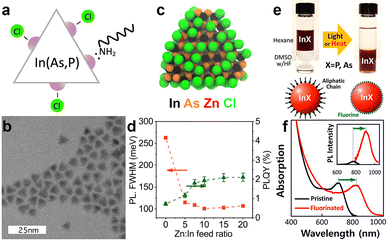 |
| Fig. 6
Surface chemistry and trap passivation of InAs QDs. (a) Schematic of the proposed surface termination and (b) TEM image of the tetradedron-shaped InAs QDs synthesized via Amino-As. Reprinted with permission from ref. 97 Copyright 2021, American Chemical Society. (c) Model of InAs QD synthesized via amino-As and passivated with ZnCl2. (d) The effect of Zn : In ratio on the PL fwhm and PLQY of the InAs QDs. Reprinted with permission from ref. 100 Copyright 2022, American Chemical Society. (e) Trap passivation of InAs QDs via surface fluorination. (f) Absorption spectra of not-passivated (black) and passivated InAs QDs via HF (red). (Inset: Normalized PL spectra). Reprinted with permission from ref. 18 Copyright 2018, American Chemical Society. | |
InAs based heterostructures and their optical characteristics
The choice of shell materials for InAs is limited due to the large lattice parameters of InAs, as previously mentioned.100 CdSe is the most popular and effective shelling material for InAs since it has a very low lattice mismatch with InAs (0.001%)135 compared to ZnSe (6.44%135) and ZnS (10.7%135). Apart from the optimal lattice matching, CdSe should form Type-I band alignment with InAs considering the bulk bandgaps of the two materials (Fig. 7a). However, CdSe forms a quasi Type-II alignment with InAs, thus delocalizing the electron wavefunction in the shell region.133,135,136 For this reason, it is necessary to cover InAs/CdSe QDs with another shell material (e.g. CdS, ZnSe or ZnS) to effectively confine the photoexcited carriers. Franke et al. synthesized InAs/CdSe/CdS QDs with emission peaked at 970 nm and a PLQY of 82%.30 The same authors observed that the PLQY values of InAs/CdSe/CdS QDs decreased when increasing the size of the InAs core, with 1425 nm emissive QDs having a PLQY of 16%. Analogously, Cao et al. reported a PLQY of 18% and broad PL fwhm (0.2 eV) for large InAs QDs overcoated with 1.2 nm CdSe shell emitting at 1120 nm.133 Upon subsequent shelling of these systems with a ZnSe outer layer (forming InAs/CdSe/ZnSe heterostructures), the PLQY could be further increased to 52% (PL peak ∼ 1065 nm).137
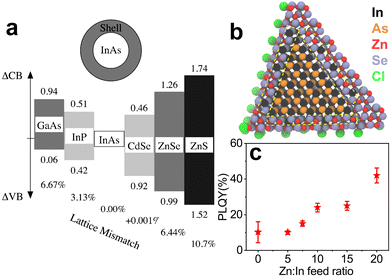 |
| Fig. 7 (a) Lattice mismatch and band offsets of different shelling materials suggested for InAs QDs. Reprinted with permission from ref. 135 Copyright 2000, American Chemical Society. (b) Model of a InAs/ZnSe core/shell QD with a hybrid In–Zn–Se layer between the pure InAs core and pure ZnSe outer shell. (c) PLQY of InAs/ZnSe core/shell QDs obtained from InAs cores made with different Zn : In feed ratios. Reprinted with permission from ref. 100 Copyright 2022, American Chemical Society. | |
InAs is considered as an alternative to toxic lead and cadmium chalcogenides, so shell materials based on Cd are not suitable to obtain RoHS-compliant emitters. In this regard, ideal shelling materials are ZnSe and ZnS, which are supposed to make a Type-I alignment with InAs (Fig. 7a). On the other hand, Zhang et al. showed that InAs/ZnSe core/shell structures are sensitive to air and they ascribed it to the extension of the electron wave function to the ZnSe shell.138 In any case, since ZnS and ZnSe have high lattice mismatch with InAs, the reported InAs/ZnS(Se) core/shell structures exhibit low PLQY values in the order of 10%.73,86 For these reasons, various attempts have been made to form graded intermediate shells of lattice-matching materials, such as InP, which has low lattice mismatch with InAs (3.13%).135 Xie et al. have grown InAs/InP/ZnSe QDs with a PLQY as high as 76% and PL peaked at 980 nm.74 Sagar et al. synthesized Cd-free InAs/In(Zn)P/GaP/ZnSe emitting at ∼1107 nm with a PLQY of 23%.47 They developed a single precursor complex to form InZnP as intermediate shell that was able to preserve the excellent size dispersion (6%) of the InAs core. In another study, InAs/In(Zn)P/ZnSe/ZnS heterostructures were prepared by the injection of In(Zn) and TMS-P precursor solutions into an InAs core solution. The QDs had a PLQY of 25% and an emission peak of 873 nm.139 Recently, Enright et al. synthesized InAs/InP/ZnSe heterostructures emitting at ∼830 nm with a PLQY >50% and found that the electron wavefunction extends isotropically into the InP shell but remains close to the InAs/InP interface, in analogy with the report of Sagar et al.140 In a recent work, our group prepared InAs/ZnSe QDs in which the lattice mismatch between the core and the shell was minimized via the formation of an In–Zn–Se interlayer, boosting the PLQY up to 42% with an emission at 860 nm (Fig. 7c).100 As mentioned earlier, Zhu et al. used ZnCl2 as additive in the InAs core synthesis, which formed an In–Zn–Se interlayer and released the strain between InAs and ZnSe (Fig. 7b) (Table 2).
Table 2 InAs QD based heterostructures and their optical characteristics
Core material |
Core size (nm) |
Shell material |
Shell thickness |
Excitonic peak (nm) |
PL peak after shelling (nm) |
fwhm (nm, meV) |
PLQY (%) |
Ref. |
InAs |
1.7 |
InP |
n/a |
886–991 |
n/a |
n/a |
n/a |
135
|
InAs |
n/a |
InP |
n/a |
990–1082 |
n/a |
n/a |
n/a |
133
|
InAs |
n/a |
InP |
1–3 nm |
880 |
905 |
60–75, 48–60 |
1.2 |
74
|
InPAs |
2–4.23 |
InP/ZnSe |
0.4 nm |
580–720 |
625–780 |
68, 54 |
3.5 |
141
|
InAs |
n/a |
InP/ZnSe |
2–3 nm |
910 |
985 |
60–75, 48–60 |
76 |
74
|
InAs |
2.7 ± 0.2 |
InP/ZnSe |
1.5 nm + 2 ML |
∼800 |
∼870 |
∼75, 130 |
60 |
140
|
InAs |
n/a |
InP/ZnSe/ZnS |
n/a |
n/a |
∼775 |
∼150, 260 |
36 |
138
|
InAs |
3.1 |
InZnP/GaP/ZnSe |
n/a |
∼953 |
∼1107 |
∼124, 100 |
23 |
47
|
InAs |
3 |
In(Zn)P/ZnSe/ZnS |
∼6.6 |
∼780 |
∼890 |
∼90, 72 |
25 |
139
|
InAs |
3.4 |
CdSe |
1.2 nm |
∼1030 |
∼1120 |
∼248, 200 |
18 |
133
|
InAs |
1.4 |
ZnCdS |
2.9 nm |
755 |
795 |
82, 66 |
35–50 |
29
|
InAs |
3.1–5.5 |
CdSe |
1 ML |
∼800 |
∼850 |
70, 56 |
∼35 |
142
|
InAs |
3.1–5.5 |
CdSe |
2 ML |
∼900 |
∼950 |
70, 56 |
∼92 |
142
|
InAs |
3.1–5.5 |
CdSe |
3 ML |
∼990 |
∼1050 |
70, 56 |
∼85 |
142
|
InAs |
3.1–5.5 |
CdSe |
4 ML |
∼1010 |
∼1100 |
70, 56 |
∼67 |
142
|
InAs |
3.1–5.5 |
CdSe |
5 ML |
∼1025 |
∼1080 |
70, 56 |
∼56 |
142
|
InAs |
n/a |
CdSe |
0.5 m |
700–1400 |
1000–1500 |
∼148, 119 |
∼13 |
99
|
InAs |
3.1 |
CdSe |
1.8 nm |
n/a |
∼1320 |
n/a |
20 |
143
|
InAs |
n/a |
CdSe/CdS |
n/a |
n/a |
970 |
n/a |
82 |
30
|
InAs |
n/a |
CdSe/CdS |
n/a |
n/a |
1100 |
n/a |
37 |
30
|
InAs |
n/a |
CdSe/CdS |
n/a |
n/a |
1300 |
n/a |
26 |
30
|
InAs |
n/a |
CdSe/CdS |
n/a |
n/a |
1450 |
n/a |
16 |
30
|
InAs |
3.1 |
CdSe/CdS |
1.8 nm |
n/a |
∼1362 |
n/a |
24 |
143
|
InAs |
1.9 |
CdSe/ZnSe |
2.8 |
n/a |
885 |
∼185, 149 |
70 |
137
|
InAs |
3.8 |
CdSe/ZnSe |
1.8 |
n/a |
1065 |
∼160, 129 |
52 |
137
|
InAs |
6.3 |
CdSe/ZnSe |
1.5 |
n/a |
1425 |
∼200, 161 |
2.5 |
137
|
InAs |
3.1 |
CdSexS1−x/CdS |
4.5 nm |
n/a |
∼1320 |
∼260, 209 |
30 |
143
|
InAs |
2–2.4 |
ZnSe |
1.5 nm |
1160–1240 |
1300 |
110–180, 88–145 |
n/a |
144
|
InAs |
n/a |
ZnSe |
n/a |
645–771 |
750–920 |
85–121, 68–97 |
1–2 |
73
|
InAs |
3.4 |
ZnSe |
n/a |
∼900 |
1010 |
147, 118 |
5–10 |
86
|
InAs |
3.4 |
ZnS |
n/a |
∼835 |
925 |
111, 89 |
5–10 |
86
|
In(Zn)As |
n/a |
ZnSe/ZnS |
n/a |
490 |
538 |
60, 48 |
60 |
93
|
In(Zn)As |
n/a |
In(Zn)P/GaP/ZnS |
n/a |
∼775 |
∼850 |
110, 192 |
75 |
94 and 95
|
InGa0.2As0.8 |
n/a |
ZnSe |
n/a |
500–600 |
580–700 |
∼50, 40 |
25.6 |
122
|
InGa0.5As0.5 |
n/a |
CdS |
n/a |
∼750 |
825 |
∼100, 80 |
9.8 |
20
|
Zn–InAs |
2.8 |
ZnSe |
∼2 ML |
∼810 |
860 |
195 |
42 |
100
|
Electronic properties and carrier dynamics
Steiner et al. have pioneered the study of electronic properties of colloidal InAs QDs using scanning tunneling spectroscopy (STS).145 In a seminal paper in 1999, they were able to identify atomic-like electronic states with s and p character in InAs QDs.146 In the tunnelling current–voltage experiments, these states are seen as multiplets (two- and six-fold for s and p states, respectively), as each peak corresponds to the injection of a single electron in the QDs. Therefore, the injection of a second electron in a partially filled s type QD “orbital” will require an additional charging energy contribution. The same group also observed that the conduction band ground state was more red-shifted than the valence band ground state in two dimensional InAs QDs arrays, a behavior that could be easily traced to the remarkably smaller effective mass of the electrons (me) compared to that of the hole (mh, mh/me = 17).145,147 Hence, electrons are more easily delocalized than holes across multiple QDs, leading to stronger coupling of the electron states compared to the hole states. In addition, InAs presents electron-donating surface states.38 All these aspects contribute to make InAs QDs one of the few n-type colloidal semiconductors in the NIR, independently of its processing, thus enabling the fabrication of photovoltaics based on rectifying junctions.27 Furthermore, InAs in the nanoscale held major promise for low-threshold carrier multiplication (CM) thanks to its energy band gap tunable in the NIR combined with the considerable difference in me and mh (with respect to CdSe, PbS and PbSe QDs).147,148
The expected low-threshold CM motivated studies on core-only and core/shell InAs QDs carrier dynamics to elucidate the various multi-exciton processes (multi-exciton dynamics plays a central role in determining the applicability of QDs in a variety of optoelectronic applications). Yet, the literature on this topic is quite limited, and this can be ascribed to the typically poor optical properties of InAs QDs resulting from not yet optimized colloidal synthesis procedures.148–152 Transient absorption (TA) measurements have been carried out on both core-only and core/shell InAs QDs to determine the carrier cooling rate, single- and bi- exciton lifetimes combined with their relative amplitude and Auger recombination. Schaller et al. performed one of the first TA studies in 2007 on InAs and InAs/CdSe core/shell QDs probing the 1S transition.148 The authors observed pump-intensity-dependent TA dynamics from which they extracted a single-exciton lifetime of 192 ps and 205 ns and a bi-exciton lifetime of 8.3 ps and 16 ps for InAs core-only and InAs/CdSe core/shell QDs, respectively (Fig. 9a and b). In addition, they measured a carrier cooling rate of 0.5 eV ps−1. Sub-picosecond carrier cooling has been reported for InAs cores of different sizes as well as in core/shell QDs,149,150,152 and a fast carrier cooling is commonly observed in other QDs such as CdSe153 ones. On the other hand, bi-exciton and single-exciton lifetimes varies greatly in all reports with the former ranging from 8 to 53 ps.148,150,151,154
Auger recombination
Auger recombination (AR) is a three-particle non-radiative process, where the energy of an electron–hole pair is transferred to either an electron or a hole instead of emitting a photon.155 AR is frequently studied via TA or PL lifetime analyses.156 Since InAs QDs show very short Auger lifetimes (τAR, <50 ps148), which can limit their optoelectronic applications, it is necessary to suppress AR to employ them in lasers,157–161 LEDs162–164 and single-photon sources.165 So far, in the literature there are reports on AR rates between 10 and 30 ps for InAs QDs, thus suggesting that AR is the main recombination channel of bi-excitons.150,151 Spencer et al. in 2019 performed pump-fluence-dependent absolute pump-probe transients of InAs QDs employing a beam scanning technique to limit the repetitive excitation.151 Through this advanced technique, they identified a τAR of 26 ± 5 ps for their 6.2 nm sized InAs QDs (Fig. 8c). Importantly, such value is consistent with what reported by Schaller et al. for 4.3 nm InAs QDs (τAR = 8.3 ps) considering QD volume scaling.148,166 Indeed, it is possible to slow down this process by improving the core/shell interfacial layer and by developing band engineering strategies. For that, Sagar et al. developed a continuously graded thick CdSexS1−x shell on InAs QDs to increase the degree of charge carrier delocalization from the core to the shell.143 This delocalization can reduce the electron–hole Coulombic interaction and slow down the Auger recombination to ∼105 ps, yielding a PLQY of 30% with emission at 1320 nm.143 On the other hand, Sagar et al. did not clearly demonstrate the influence of the graded shelling on the photophysical properties of InAs QDs.
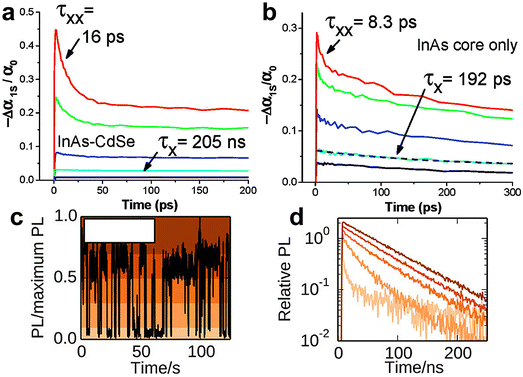 |
| Fig. 8
Carrier dynamics of InAs QDs. (a) Pump dependent TA dynamics measured for an InAs/CdSe core/shell QDs colloidal suspension (λpump = 800 nm, λprobe = 1000 nm) showing a slow single-exciton relaxation at low pump intensity (black and cyan lines) and a fast relaxation component for high pump intensity (blue, green, and red lines). (b) Pump dependent TA dynamics measured for an InAs QDs suspension (λprobe = 800 nm, λprobe = 950 nm) showing a constant single-exciton dynamic with constant decay. Reprinted with permission from ref. 148 Copyright 2007, American Chemical Society. (c) PL of a single InAs/CdZnS QD over time. (d) PL lifetime of the single InAs/CdZnS QD in panel (c) during emission states of various intensity: high, intermediate, and low emission intensity conditions (colors of curves correspond to the shaded region in panel c, see the reference). Reprinted with permission from ref. 168 Copyright 2014, American Chemical Society. | |
Single QD PL
Single InAs QD PL has been mainly addressed by the works of Correa et al. and Bischof et al.167,168 Here, the limited number of reports is due to the weak NIR emission of individual InAs QDs whose observation requires superconducting nanowire single-photon detectors. In 2012, Correa et al. performed PL measurements on single InAs/CdSe core/shell QDs where they observed two state blinking with an emission spectra centered at 1300 nm.167 Following that seminal work, the group reported further measurements on InAs/CdZnS168 in 2014, where they were able to perform a detailed analysis of the emission dynamics. Here, they observed PL blinking from 24 individual InAs/CdZnS QDs. Some of the QDs demonstrated binary blinking (switching between states of high intensity and low intensity, as observed in CdSe),169 while other QDs exhibited an intermediate intensity. The varying blinking behavior was accompanied by a radiative lifetime ranging from 50 to 200 ns and the authors could correlate a decrease of radiative lifetime to a decreased emission intensity (i.e. high, intermediate and low intensity states, Fig. 8c and d). Antibunching measurements performed on the very same QDs allowed the identification of the biexciton PLQY, which was found to vary from <1% up to 43%.168
Applications
Bioimaging
Semiconductor QDs have been widely used for biomedical applications such as bioimaging,170,171 biosensing172 and neural photostimulation.63,173–175 InAs QDs are of interest for bioimaging and biomedical labeling since they can be both excited and detected in the NIR-II region of the spectrum (1000–1700 nm) (Fig. 9a), where tissue absorption and auto-fluorescence is minimal (Fig. 9b and c).124,176,177 Furthermore, InAs QDs are RoHS-compliant and less toxic than Cd-, Pb- and Hg-based materials, as already mentioned. In this regard, Kim et al. successfully exploited InAs QDs in a sentinel lymph node mapping experiment for the first time.141 In details, they synthesized InAsxP1−x/InP/ZnSe alloyed QDs (by using TMS-As and TMS-P reagents) and capped them with oligomeric phosphines. These QDs had a small hydrodynamic size (∼12 nm), aqueous stability and PLQY of 3.5% in water. Despite their low PLQY, 150 pmol of subdermally injected InAs0.82P0.18/InP/ZnSe alloyed QDs could be easily detected by bioimaging systems since they can enter the lymphatics and migrate within 1 minute to the sentinel node (Fig. 9d). In another study, the same group employed dihydrolipoic acid conjugated to a short poly(ethylene glycol) (DHLA-PEG) as surfactants for more emissive InAs/ZnSe core/shell QDs (PLQY of 6–9% in water).73 In contrast to oligomeric phosphines, QDs capped with DHLA-PEG can be used as efficient and specific in vivo labels since they can circulate for a longer time (up to 5 minutes) and migrate out of the blood vessels and into the interstitial fluid (Fig. 9e). Another possible capping agent for InAs QDs is 3-mercaptopropionic acid (3-MPA), which was used by Xie et al. for ex vivo fluorescence imaging of major organs of mice (Fig. 9f).74 In terms of imaging depth, Allen et al. could clearly image tumor vasculature (E0771 mammary) even up to 200 μm using InAs/ZnCdS core/shell QDs (Fig. 9g).29
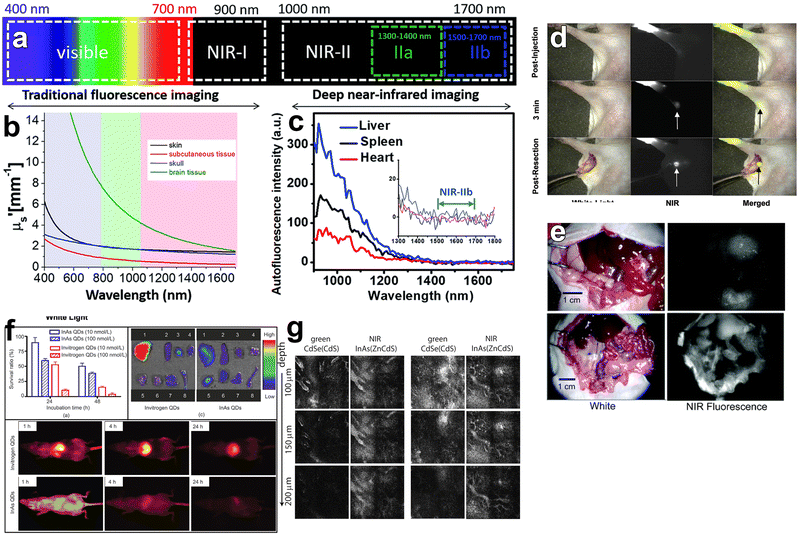 |
| Fig. 9
In vivo bioimaging using InAs based QDs emitting in NIR-I region. (a) Spectral ranges of fluorescence imaging. (b) Reduced scattering coefficient (μs) of the various tissue types: skin (black), brain tissue (green), skull (blue) and subcutaneous tissue (red). (c) Auto-fluorescence spectrum of ex vivo mouse liver (blue), spleen (black) and heart (red) tissue. Reprinted with permission from ref. 176, Copyright 2018, The Royal Society of Chemistry. (d) Images of injected oligomeric phosphines capped InAs0.82P0.18/InP/ZnSe core/shell/shell QDs taken by white light, NIR fluorescence, and color/NIR merge, respectively. Reprinted with permission from ref. 141 Copyright 2005, American Chemical Society. (e) InAs/ZnSe core/shell QDs capped with DHLA (up) and DHLA-PEG (down) used for bioimaging. Reprinted with permission from ref. 73 Copyright 2006, American Chemical Society. (f) Cytotoxicity and bioimaging of mice major organs via 3-MPA capped InAs/InP/ZnSe core/shell/shell QDs. Reprinted with permission from ref. 74 Copyright 2008, Springer Nature. (g) Images of the InAs/ZnCdS and CdSe/CdS core/shell QDs injected intravenously and imaged simultaneously in a mammary tumor in a mouse (scale bar: 100 μm). Reprinted with permission from ref. 29 Copyright 2010, American Chemical Society. | |
In contrast to previous QDs emitting below 900 nm, Franke et al. synthesized InAs/CdSe/CdS core/shell/shell QDs emitting at 970 nm, 1100 nm and 1300 nm.30 They injected the mixture of these QDs into an anaesthetized mouse via the tail vein and the vasculature of the mouse brain was imaged through the intact skin and skull using diffuse 808 nm excitation. Their results showed that longer imaging wavelengths enhance the spatial resolution of bio structures by increasing the signal to background ratio (Fig. 10a). Furthermore, they saw a tradeoff between increased resolution and decreased imaging speed at longer imaging wavelengths. These points indicate the need for NIR-II emitting InAs QDs with narrow emission and high PLQYs at the red edge of short-wavelength infrared region (SWIR) detectors. Similarly, Bruns et al. used InAs/CdSe/CdS (λem = 1080 nm) and InAs/CdSe/ZnSe (λem = 1280 nm) core/shell/shell QDs and functionalized them with three distinct surface coatings (phospholipid micelles, lipoproteins and composite) to tailor the physiological properties for specific SWIR imaging applications.77 Surface functionalization with phospholipid micelles allowed long blood circulations times and thus enabled assessment and quantification of heart rate and respiration of both sedated and awake mice (Fig. 10b). By tracking individual composite InAs particles during intravital microscopy, they could directly identify arteries/veins, and quantify blood flow in the vasculature of the brain (Fig. 10c).
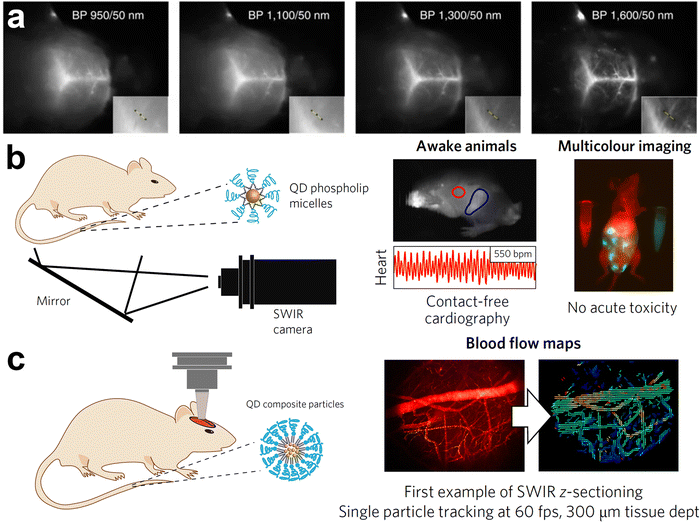 |
| Fig. 10
In vivo bioimaging using InAs based QDs emitting in NIR-II region. (a) Fluorescence imaging of mouse brain vasculature through intact skin and skull using a mixture of InAs/CdSe/CdS core/shell/shell QDs emitting at 970 nm, 1100 nm, 1300 nm and 1600 nm. Reprinted with permission from ref. 30, Copyright 2016, Springer Nature. (b) Bioimaging in the ventral orientation at 30 fps with InAs QDs functionalized with phospholipid micelles under excitation of 808 nm allowed imaging of vital signs such as heart rate (λExc = 808 nm). (c) High-resolution and high-speed SWIR intravital imaging using InAs composite QDs in a mouse with a cranial window to generate flow maps of microvascular networks (λExc = 808 nm; longpass: 1000 nm). Reprinted with permission from ref. 77, Copyright 2017, Springer Nature. | |
Electronics
It is possible to fabricate stable p–n homojunction-based devices such as field-effect transistors (FETs) by controlling the carrier type and transport in QD solids.178,179 Narrow band-gap III–V semiconductors (e.g. InAs, InSb and InN) are of interest for FETs due to their high electron mobility (2 × 104 cm2 V−1 s−1 at 300 K for InAs16), high chemical stability and, most importantly, RoHS-compliance. Furthermore, surface dangling bonds of these semiconductors pin the InAs surface Fermi level in the conduction band and create an electron accumulation layer on the surface.16,38,180 Likewise, InAs QDs show n-type behavior which has been attributed to electron donating surface states, as probed by Steiner et al. using scanning tunneling spectroscopy.145
In 2010, Soreni-Harari et al. fabricated FETs using colloidal InAs QDs for the first time and investigated the effect of the interface region within the active layer of InAs through a dedicated surface and film treatment (Fig. 11a).181 Firstly, they replaced the native TOP molecules capping InAs QDs with aniline via a ligand exchange procedure, then assembled QDs onto the amine linking sites of 3-(trimethoxysilyl)propylamine (APS)-functionalized surface, and finally crosslinked them with ethylenediamine (EDA). Using this approach, they achieved high quality films exhibiting an Ion/off of 105 and a linear regime mobility of 1.2 × 10−5 cm2 V−1 s−1. Their InAs QDs based device showed n-type conduction characteristic and no p-channel conductance. Recently, Tripathi et al. showed that copper doping of InAs QDs improves the n-type mobility by 150% due to the surface trap passivation (Fig. 11b).129 In another study, Liu et al. reported a performance enhancement of InAs based FETs using InAs QDs capped with molecular metal chalcogenide complexes (MCCs).182 The electron mobility of InAs QDs was enhanced up to a factor of 106 when replacing the native organic ligands with inorganic anions such as Cu7S4− (Fig. 11d and f). The FETs were fabricated by depositing ∼27 nm of Cu7S4− capped InAs QDs on silicon wafer obtaining an electron mobility of 16 cm2 V−1 s−1 in the linear regime and 14.8 cm2 V−1 s−1 in the saturation regime (a value which is orders of magnitude higher than previously reported for InAs-181 and CdSe-183–186 FETs). The electron mobility increased with increasing annealing temperature and rapid thermal annealing (RTA) gave the highest mobility (Fig. 11c).
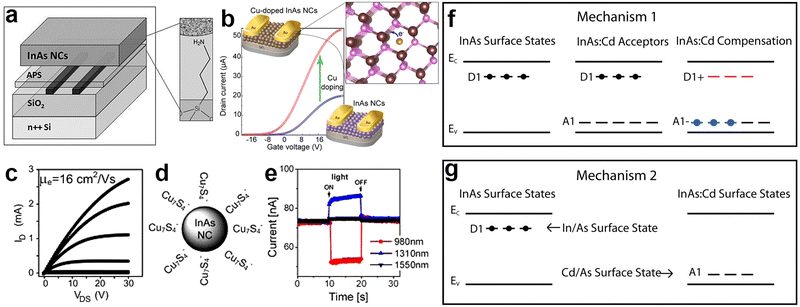 |
| Fig. 11
InAs QD based field effect transistors (FETs). (a) Schematic of InAs QD based FET consisting of heavily doped silicon gate, 100 nm dry thermal silicon oxide, Cr/Au (5 nm/50 nm) source/drain contacts, 3-(trimethoxysilyl)propylamine (APS) self-assembled molecule and InAs QD film as the semiconductor. Reprinted with permission from ref. 181, Copyright 2010, John Wiley and Sons (b) illustration of the InAs and Cu:InAs based FET and enhancement of the mobility upon Cu doping. Reprinted with permission from ref. 129 Copyright 2019, American Chemical Society. (c) Plots of the drain current (ID) vs. drain-source voltage (VDS) measured at different gate voltages (VG), (d) schematic representaion and (e) ambipolar photoresponse of FET device based on Cu7S4 capped InAs QDs. Reprinted with permission from ref. 182 Copyright 2013, American Chemical Society. (f and g) Two approaches for the p-type doping of Cd:InAs QDs. Reprinted with permission from ref. 179 Copyright 2010, American Chemical Society. | |
In order to manipulate the charge carrier type of InAs QDs and n-type to p-type conversion for FETs, two approaches were reported. In the first approach, Cd atoms were incorporated at indium sites in the lattice, leading to acceptor states above the valence band maximum and inducing a p-type semiconductor behavior (Fig. 11f).179 In the second approach, Cd atoms were doped on the InAs surface, acting as substitutional p-dopants (see “synthesis of InAs-based alloyed/doped QDs” subsection for more details) (Fig. 11g).36,179 Furthermore, Cd doping created a protective layer and increased the chemical stability against oxidation under ambient atmosphere. The characteristics and performance of the InAs based FETs are summarized in Table 3.
Table 3 FET characteristics of the reported InAs based FET devices. All of them are n-type except Cd:InAs, which is p-type
QD type |
Ligand |
Layer thickness (nm) |
μ
lin (cm2V−1 s−1) |
μ
sat (cm2V−1 s−1) |
I
on/off
|
Ref. |
InAs |
Cu7S4− |
∼27 |
16.0 |
14.8 |
∼103 |
182
|
InAs |
DOA |
50 |
1.2 × 10−4 |
1.3 × 10−4 |
103 |
27
|
InAs |
Br |
50 |
1.4 × 10−3 |
1.7 × 10−3 |
104 |
27
|
InAs |
MPA |
50 |
2.0 × 10−3 |
2.5 × 10−3 |
104 |
27
|
InAs |
TOP |
n/a |
2.5 × 10−6–5 × 10−6 |
n/a |
103 |
181
|
InAs |
EDA |
n/a |
8.5 × 10−6–1.2 × 10−5 |
n/a |
105 |
181
|
InAs |
EDT |
35 |
0.08 |
0.07 |
105 |
129
|
Cu:InAs |
EDT |
35 |
0.18 |
0.15 |
104 |
129
|
Cd:InAs |
EDT |
35 |
1.5 × 10−3 |
n/a |
n/a |
36
|
Optoelectronics
InAs QDs have only marginally being tested in common optoelectronic devices such as LEDs95,144,187,188 and photovoltaic cells.27 Yet, to date, there have been no reports on amplified spontaneous emission or lasing from InAs QDs. Regarding photovoltaic applications, Song et al. obtained power conversion efficiency of 7.92% from a p–n junction between n-type InAs and p-type PbS colloidal QD layers (Fig. 12a and b).27 They assembled defect-controlled conductive InAs films via a two-step surface modification. They stripped the native ligands (oleic acid) from InAs QDs with NOBF4 and replaced them with either 1,2-ethanedithiol (EDT), 3-MPA, I, Br or Cl. Interestingly, the presence of these new ligands led to a shift of the band edges of the QDs up to 0.4 eV. The dipole moment of the ligand/QD interface and the intrinsic dipole moment of the ligand were employed to modify the energy levels.189 Ligand-induced surface dipoles can indeed control the energy levels in addition to quantum confinement-controlled bandgap modification. Halide ligands create deeper band energy levels since they have large interface dipole moments as reported for PbS QDs.190 Among them, InAs capped with I− showed the deepest energy level (−5.4 eV). On the other hand, EDT capped InAs exhibited EVB around −5.1 eV possibly due to the offset direction of the interface dipole moment and the ligand intrinsic dipole moment (Fig. 12c). These findings reveal that rational ligand engineering holds promise for the fabrication of defect-controlled conductive InAs based assemblies and efficient photovoltaics.
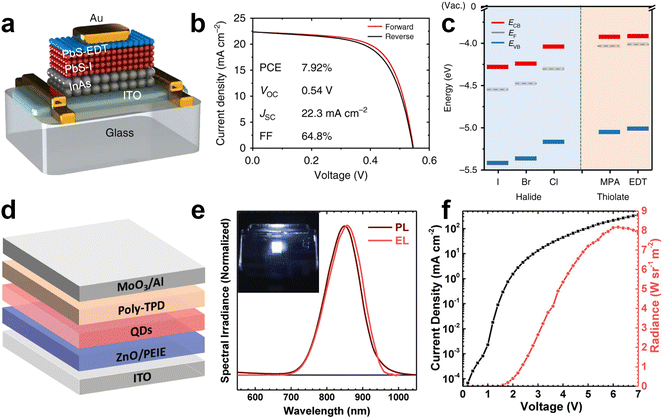 |
| Fig. 12
InAs QDs for photovoltaics and NIR LEDs. (a) Schematic and (b) J–V of the n-type InAs based p–n junction photovoltaics. (c) Energy levels ECB, EVB, and EF (vs. the vacuum level) of InAs QD films capped with different ligands. Reprinted with permission from ref. 27, Copyright 2018, Springer Nature. (d) Schematic of ITO/ZnO/PEIE/In(Zn)As@In(Zn)P@GaP@ZnS QDs/Poly-TPD/MoO3/Al NIR LED and (e) EL and PL spectra of In(Zn)As/In(Zn)P/GaP/ZnS QDs. (Inset: NIR image of the operating NIR LED). (f) Current density and radiance versus voltage characteristics of InAs based NIR LED. Reprinted with permission from ref. 95, Copyright 2020, John Wiley and Sons. | |
Electroluminescence (EL) has been one of the prime applications of colloidal QDs. While QD LEDs emitting in the visible spectral region are well-established,2,164,191–193 NIR QD LED are still not fully developed. InAs QDs represent one of the most appealing QDs for the fabrication of NIR LEDs, since they are fully RoHS-complaint and capable of fully covering the NIR spectrum. Due to the low in-film PLQY and high nonradiative processes, there are only few reports on the application of InAs in EL devices. In 2002 Tessler et al. reported the very first NIR LED based on InAs QDs where they incorporated InAs/ZnSe core/shell QDs in a conjugated polymer matrix exhibiting energy transfer to the NIR emitting QDs. The hybrid organic/inorganic NIR LED showed external quantum efficiency (EQE) of 0.5% and emission around 1200 nm.144 A second example is the recent work from Wijaya et al., where they built an inverted NIR LED based on In(Zn)As/In(Zn)P/GaP/ZnS core/shell/shell/shell, ZnO electron transport layer and a poly(N,N′-bis-4-butylphenyl-N,N′-bisphenyl)benzidine (Poly-TPD) hole transport layer (Fig. 12d).95 They demonstrated a NIR LED emitting in the 800–900 nm range with an EQE of 4.6% and radiance of 8.2 W sr−1 m−2 (Fig. 12e and f). By employing similar core/shell/shell/shell QDs, but featuring a larger InAs core, the same group reported NIR LEDs with an EQE up to 13.3% at 1006 nm.187 It should be noted here that such performance was achieved using QDs with a relatively complex architecture, which requires an elaborate synthesis protocol based on both TMS-As and TMS-P. On the contrary, De Franco et al. recently demonstrated a stable NIR LED based on much simpler InAs/ZnSe QDs synthesized via amino-As and reported EQE of 5.5% at 947 nm.188 These works highlight the potential of InAs QDs for NIR LEDs; yet, they point out as well the necessity to develop efficient InAs QDs emitting in NIR-II region and device engineering for increasing the EQE of NIR LEDs.
Outlook & future aspects
Herein, we have reviewed comprehensively the progress made so far on the colloidal synthesis and applications of InAs QDs. These results enrich the field of NIR QDs and suggest a promising future for InAs QDs since it is the only RoHS-compliant available semiconductor that can absorb and emit in the whole range of NIR. However, the overall toxicity of InAs QDs must be assessed in the future. For instance, it is still unknown whether the cytotoxicity of InAs QDs originates from the released In3+ and As3− ions upon etching or from the intracellular distribution of QDs and the associated nanoscale effects as reported for Cd-based QDs.194 From the synthesis point of view, the research on colloidal InAs QDs is a hot topic as improvements in the synthesis are required to achieve further control over the size, size distribution and optical properties enabling a variety of optoelectronic/electronic applications. These issues need to be addressed before InAs QDs can gain equivalent attention in NIR technology as lead chalcogenides. In particular, the low PLQY of InAs QDs currently limits their application in NIR LEDs and so far, most of the studies on NIR LED were focused on lead-containing QDs7 or perovskite host matrix showing EQE up to 16%.195 In terms of optical properties, it is necessary to elucidate further the carrier dynamics and assess the exciton fine structure as well as the origin of the dark exciton radiative recombination in core and core/shell InAs QDs; along the same line of what reported for InP QDs (the “neighboring” III–V semiconductor system, see ref. 196). Furthermore, we also have little knowledge about the surface chemistry of InAs QDs and how ligands can affect their surface energy, nucleation, growth, and shape evolution. So, comprehensive XPS, FTIR, and NMR studies are needed since surface chemistry engineering is crucial to create “strain-free” core/shell systems (to increase their PLQY) and facilitate the development of InAs based NIR LEDs.100,188 Future progress on novel precursors and synthetic methodologies should not only pursue the goal of making more luminescent InAs QDs, but also reduce non-radiative processes such as Auger recombination, specifically for QD-based optical gain media in the NIR range. In addition, the photo- as well as chemical-stability of InAs must be investigated in detail. On the other hand, InAs QDs can be attractive for luminescent solar concentrators (LSCs) due to their tunable absorption over the entire visible range as well as the closely-matched PL with the low-energy part of the EQE spectrum of the silicon-based photovoltaic cell. However, efficient LSCs demand reduced reabsorption while maintaining a high PLQY, so effective material design and device engineering are still required for InAs QDs as performed previously for InP-2,197–199 and CISeS-200 QD based LSCs. Taken together, the urgent need for RoHS-compliant IR semiconductors creates a powerful motivation for the QD community to work on efficient InAs QDs. On the other hand, the range of 1550–1600 nm is important for telecommunication as well as quantum secure communication28 and deep tissue imaging34 and so far only lead- (PbSe201,202 and PbTe203) and mercury-based (HgTe204 and HgSe205) QDs can emit efficiently in this range. Shifting the emission wavelength to the 1550–1600 nm range is therefore another challenge for future studies, since the longest reported emission wavelength of colloidal InAs QDs is around 1500 nm.99 The synthesis of alloyed In–As–Sb QDs could be one approach to tune the PL emission range to longer wavelength. Large-scale production is yet another issue for InAs QDs considering the hazardous and expensive arsenide precursors as well as limited supply of indium element. For that, commercially available and non-hazardous precursors must be developed; while non-injection organometallic synthesis approaches to prepare large quantities require extensive investigation. Last but not least, the identification of structural defects of InAs synthesized via either TMS-As or amino-As is still a challenge, so strategies to cure such defects have to be explored as reported for GaAs QDs.206 Finally, progress on InAs QDs relies on novel synthesis techniques as well as effective post-synthesis modifications, which provide the keys to efficient optical and electronic performance.
Abbreviations
AR | Auger recombination |
amino-As | Tris(dimethylamino) arsine |
APS | 3-(Trimethoxysilyl)propylamine |
AsTMGe3 | Tris(trimethylgermyl)arsine |
AsH3 | Arsine |
AsPh3 | Arsenic silylamide |
CM | Carrier multiplication |
Cd | Cadmium |
CdS | Cadmium sulfide |
CdSe | Cadmium selenide |
C6H15In | Triethylindium |
DIBAL-H | Diisobutylaluminum hydride |
DMAH | Dimethylaluminum hydride |
DMEA-AlH3 | Dimethylethylamine complex |
DOA | Dioctylamine |
EDT | 1,2-Ethanedithiol |
EL | Electroluminescence |
EQE | External quantum efficiency |
eV | Electron volt |
FET | Field effect transistor |
HF | Hydrofluoric acid |
In(Ac)3 | Indium acetate |
InAs | Indium arsenide |
InCl3 | Indium chloride |
InP | Indium phosphide |
(iPrDMSi)3As | Tris(isopropyldimethylsilyl) arsine |
LA | Lauric acid |
LED | Light emitting diode |
LiEt3BH | Lithium triethylborohydride |
LSC | Luminescent solar concentrators |
MA | Myristic acid |
MBE | Molecular beam epitaxy |
MCC | Metal chalcogenide complexes |
[(Me3Si)2N]2AsCl | Triphenylarsine |
ML | Monolayer |
MPA | 3-Mercaptopropionic acid |
NC | Nanocrystal |
NIR | Near infrared |
NW | Nanowire |
NR | Nanorod |
OAm | Oleylamine |
ODE | Octadecene |
PA | Palmitic acid |
PbS | Lead sulfide |
PL | Photoluminescence |
PLQY | Photoluminescence quantum yield |
PNC | Prenucleation cluster |
P(NEt2)3 | Tris(diethylamino)phosphine |
QD | Quantum dot |
RES | Reticuloendothelial system |
RoHS | Restriction of hazardous substances |
SWIR | Shortwave infrared |
TA | Transient absorption |
(TEGe)3As | Tris(triethylgermanyl)arsine |
TOP | Trioctylphosphine |
TMGe3As | Tris(trimethylgermyl)arsine |
TMS-As | Tris(trimethylsilyl)arsine |
RTA | Rapid thermal annealing |
ZnSe | Zinc selenide |
Conflicts of interest
There are no conflicts of interest to declare.
Acknowledgements
L. M., F. D. S., and H. B. J. acknowledge support by the European Union's Horizon 2020 research and innovation program under the Marie Skłodowska-Curie Grant Agreement 101024823 (INFLED). F. D. S. also acknowledges support by the European Research Council via the ERC-StG “NANOLED” (Grant 851794).
References
- M. Liu, N. Yazdani, M. Yarema, M. Jansen, V. Wood and E. H. Sargent, Nat. Electron., 2021, 4, 548–558 CrossRef.
- H. B. Jalali, S. Sadeghi, I. B. Dogru Yuksel, A. Onal and S. Nizamoglu, Nano Res., 2022, 15, 4468–4489 CrossRef.
- M. Jiao, A. S. Portniagin, X. Luo, L. Jing, B. Han and A. L. Rogach, Adv. Opt. Mater., 2022, 10, 2200226 CrossRef.
- H. Lu, G. M. Carroll, N. R. Neale and M. C. Beard, ACS Nano, 2019, 13, 939–953 Search PubMed.
- A. Shrestha, M. Batmunkh, A. Tricoli, S. Z. Qiao and S. Dai, Angew. Chem., Int. Ed., 2019, 58, 5202–5224 CrossRef PubMed.
- C. Gréboval, A. Chu, N. Goubet, C. Livache, S. Ithurria and E. Lhuillier, Chem. Rev., 2021, 121, 3627–3700 CrossRef.
- L. Gao, L. N. Quan, F. P. García de Arquer, Y. Zhao, R. Munir, A. Proppe, R. Quintero-Bermudez, C. Zou, Z. Yang, M. I. Saidaminov, O. Voznyy, S. Kinge, Z. Lu, S. O. Kelley, A. Amassian, J. Tang and E. H. Sargent, Nat. Photonics, 2020, 14, 227–233 CrossRef.
- S. Pradhan, M. Dalmases and G. Konstantatos, Adv. Mater., 2020, 32, 2003830 CrossRef.
- S. Pradhan, F. Di Stasio, Y. Bi, S. Gupta, S. Christodoulou, A. Stavrinadis and G. Konstantatos, Nat. Nanotechnol., 2019, 14, 72–79 CrossRef CAS PubMed.
- F. Xu, X. Ma, C. R. Haughn, J. Benavides, M. F. Doty and S. G. Cloutier, ACS Nano, 2011, 5, 9950–9957 CrossRef CAS.
- M. Biondi, M.-J. Choi, S. Lee, K. Bertens, M. Wei, A. R. Kirmani, G. Lee, H. T. Kung, L. J. Richter and S. Hoogland, ACS Energy Lett., 2021, 6, 468–476 CrossRef CAS.
- Y. Cao, A. Stavrinadis, T. Lasanta, D. So and G. Konstantatos, Nat. Energy, 2016, 1, 16035 CrossRef CAS.
- C. L. Hartley and J. L. Dempsey, Chem. Mater., 2021, 33, 2655–2665 CrossRef CAS.
- X. Lan, O. Voznyy, F. P. García de Arquer, M. Liu, J. Xu, A. H. Proppe, G. Walters, F. Fan, H. Tan, M. Liu, Z. Yang, S. Hoogland and E. H. Sargent, Nano Lett., 2016, 16, 4630–4634 CrossRef CAS PubMed.
- G. Schileo and G. Grancini, J. Mater. Chem. C, 2021, 9, 67–76 RSC.
- A. Milnes and A. Polyakov, Mater. Sci. Eng., B, 1993, 18, 237–259 CrossRef.
- J. R. Heath, Chem. Soc. Rev., 1998, 27, 65–71 RSC.
- T.-G. Kim, D. Zherebetskyy, Y. Bekenstein, M. H. Oh, L.-W. Wang, E. Jang and A. P. Alivisatos, ACS Nano, 2018, 12, 11529–11540 CrossRef CAS.
- P. Reiss, M. Carrière, C. Lincheneau, L. Vaure and S. Tamang, Chem. Rev., 2016, 116, 10731–10819 CrossRef CAS PubMed.
- V. Srivastava, V. Kamysbayev, L. Hong, E. Dunietz, R. F. Klie and D. V. Talapin, J. Am. Chem. Soc., 2018, 140, 12144–12151 CrossRef CAS.
- S. Tamang, C. Lincheneau, Y. Hermans, S. Jeong and P. Reiss, Chem. Mater., 2016, 28, 2491–2506 CrossRef.
- E. Directive, Off. J. Eur. Communities, 2013, 46, 19–23 Search PubMed.
- J. Sobhanan, P. Jones, R. Kohara, S. Sugino, M. Vacha, C. Subrahmanyam, Y. Takano, F. Lacy and V. Biju, Nanoscale, 2020, 12, 22049–22058 RSC.
- H. Lin and P. Hwang, Bull. Environ. Contam. Toxicol., 1998, 61, 123–128 CrossRef PubMed.
- V. K. Sharma and M. Sohn, Environ. Int., 2009, 35, 743–759 CrossRef CAS PubMed.
- S. A. Jewett and A. Ivanisevic, Acc. Chem. Res., 2012, 45, 1451–1459 CrossRef CAS PubMed.
- J. H. Song, H. Choi, H. T. Pham and S. Jeong, Nat. Commun., 2018, 9, 4267 CrossRef PubMed.
- T. Müller, J. Skiba-Szymanska, A. B. Krysa, J. Huwer, M. Felle, M. Anderson, R. M. Stevenson, J. Heffernan, D. A. Ritchie and A. J. Shields, Nat. Commun., 2018, 9, 862 CrossRef.
- P. M. Allen, W. Liu, V. P. Chauhan, J. Lee, A. Y. Ting, D. Fukumura, R. K. Jain and M. G. Bawendi, J. Am. Chem. Soc., 2010, 132, 470–471 CrossRef CAS PubMed.
- D. Franke, D. K. Harris, O. Chen, O. T. Bruns, J. A. Carr, M. W. B. Wilson and M. G. Bawendi, Nat. Commun., 2016, 7, 12749 CrossRef CAS.
- P. Yu, M. C. Beard, R. J. Ellingson, S. Ferrere, C. Curtis, J. Drexler, F. Luiszer and A. J. Nozik, J. Phys. Chem. B, 2005, 109, 7084–7087 CrossRef CAS PubMed.
- L. Cademartiri, E. Montanari, G. Calestani, A. Migliori, A. Guagliardi and G. A. Ozin, J. Am. Chem. Soc., 2006, 128, 10337–10346 CrossRef PubMed.
- K. Takemoto, M. Takatsu, S. Hirose, N. Yokoyama, Y. Sakuma, T. Usuki, T. Miyazawa and Y. Arakawa, J. Appl. Phys., 2007, 101, 081720 CrossRef.
- M. Zhang, J. Yue, R. Cui, Z. Ma, H. Wan, F. Wang, S. Zhu, Y. Zhou, Y. Kuang, Y. Zhong, D.-W. Pang and H. Dai, Proc. Natl. Acad. Sci. U. S. A., 2018, 115, 6590–6595 CrossRef.
- D. Zhitomirsky, M. Furukawa, J. Tang, P. Stadler, S. Hoogland, O. Voznyy, H. Liu and E. H. Sargent, Adv. Mater., 2012, 24, 6181–6185 CrossRef.
- L. Asor, J. Liu, Y. Ossia, D. C. Tripathi, N. Tessler, A. I. Frenkel and U. Banin, Adv. Funct. Mater., 2021, 31, 2007456 CrossRef CAS.
- D. Mocatta, G. Cohen, J. Schattner, O. Millo, E. Rabani and U. Banin, Science, 2011, 332, 77–81 CrossRef CAS PubMed.
- M. Noguchi, K. Hirakawa and T. Ikoma, Phys. Rev. Lett., 1991, 66, 2243 CrossRef CAS PubMed.
- D. V. Talapin and C. B. Murray, Science, 2005, 310, 86–89 CrossRef CAS.
- M. Shim and P. Guyot-Sionnest, Nature, 2000, 407, 981–983 CrossRef CAS.
- J. Y. Woo, J.-H. Ko, J. H. Song, K. Kim, H. Choi, Y.-H. Kim, D. C. Lee and S. Jeong, J. Am. Chem. Soc., 2014, 136, 8883–8886 CrossRef CAS.
- X. Gong, Z. Yang, G. Walters, R. Comin, Z. Ning, E. Beauregard, V. Adinolfi, O. Voznyy and E. H. Sargent, Nat. Photonics, 2016, 10, 253–257 CrossRef.
- G. J. Supran, K. W. Song, G. W. Hwang, R. E. Correa, J. Scherer, E. A. Dauler, Y. Shirasaki, M. G. Bawendi and V. Bulović, Adv. Mater., 2015, 27, 1437–1442 CrossRef PubMed.
- L. Sun, J. J. Choi, D. Stachnik, A. C. Bartnik, B.-R. Hyun, G. G. Malliaras, T. Hanrath and F. W. Wise, Nat. Nanotechnol, 2012, 7, 369–373 CrossRef PubMed.
- O. E. Semonin, J. C. Johnson, J. M. Luther, A. G. Midgett, A. J. Nozik and M. C. Beard, J. Phys. Chem. Lett., 2010, 1, 2445–2450 CrossRef.
- B. L. Wehrenberg, C. Wang and P. Guyot-Sionnest, J. Phys. Chem. B, 2002, 106, 10634–10640 CrossRef CAS.
- L. K. Sagar, G. Bappi, A. Johnston, B. Chen, P. Todorović, L. Levina, M. I. Saidaminov, F. P. García de Arquer, S. Hoogland and E. H. Sargent, Chem. Mater., 2020, 32, 2919–2925 CrossRef CAS.
- M. L. Steigerwald, A. P. Alivisatos, J. Gibson, T. Harris, R. Kortan, A. Muller, A. Thayer, T. Duncan, D. Douglass and L. E. Brus, J. Am. Chem. Soc., 1988, 110, 3046–3050 CrossRef CAS.
- C. B. Murray, D. J. Norris and M. G. Bawendi, J. Am. Chem. Soc., 1993, 115, 8706–8715 CrossRef CAS.
- D. V. Talapin, A. L. Rogach, A. Kornowski, M. Haase and H. Weller, Nano Lett, 2001, 1, 207–211 CrossRef CAS PubMed.
- B. Blackman, D. M. Battaglia, T. D. Mishima, M. B. Johnson and X. Peng, Chem. Mater., 2007, 19, 3815–3821 CrossRef.
- G. Morello, M. De Giorgi, S. Kudera, L. Manna, R. Cingolani and M. Anni, J. Phys. Chem. C, 2007, 111, 5846–5849 CrossRef.
- K.-T. Yong, Y. Sahoo, M. T. Swihart and P. N. Prasad, J. Phys. Chem. C, 2007, 111, 2447–2458 CrossRef.
- M. A. Hines and G. D. Scholes, Adv. Mater., 2003, 15, 1844–1849 CrossRef.
- W. Lin, K. Fritz, G. Guerin, G. R. Bardajee, S. Hinds, V. Sukhovatkin, E. H. Sargent, G. D. Scholes and M. A. Winnik, Langmuir, 2008, 24, 8215–8219 CrossRef CAS.
- L. Cademartiri, J. Bertolotti, R. Sapienza, D. S. Wiersma, G. Von Freymann and G. A. Ozin, J. Phys. Chem. B, 2006, 110, 671–673 CrossRef CAS.
- K. A. Abel, J. Shan, J.-C. Boyer, F. Harris and F. C. van Veggel, Chem. Mater., 2008, 20, 3794–3796 CrossRef CAS.
- J. J. Urban, D. V. Talapin, E. V. Shevchenko and C. B. Murray, J. Am. Chem. Soc., 2006, 128, 3248–3255 CrossRef CAS.
- P. M. Allen, B. J. Walker and M. G. Bawendi, Angew. Chem., Int. Ed., 2010, 49, 760–762 CrossRef CAS.
- D. K. Harris and M. G. Bawendi, J. Am. Chem. Soc., 2012, 134, 20211–20213 CrossRef CAS.
- H. Li, D. Chen, L. Li, F. Tang, L. Zhang and J. Ren, CrystEngComm, 2010, 12, 1127–1133 RSC.
- F. Pietra, L. De Trizio, A. W. Hoekstra, N. Renaud, M. Prato, F. C. Grozema, P. J. Baesjou, R. Koole, L. Manna and A. J. Houtepen, ACS Nano, 2016, 10, 4754–4762 CrossRef CAS PubMed.
- H. Bahmani Jalali, M. Mohammadi Aria, U. M. Dikbas, S. Sadeghi, B. Ganesh Kumar, M. Sahin, I. H. Kavakli, C. W. Ow-Yang and S. Nizamoglu, ACS Nano, 2018, 12, 8104–8114 CrossRef CAS.
- J. Q. Grim, L. Manna and I. Moreels, Chem. Soc. Rev., 2015, 44, 5897–5914 RSC.
- R. L. Wells, C. G. Pitt, A. T. McPhail, A. P. Purdy, S. Shafieezad and R. B. Hallock, Chem. Mater., 1989, 1, 4–6 CrossRef CAS.
- T. Kuech, Mater. Sci. Rep., 1987, 2, 1–49 CrossRef.
- M. Yano, M. Nogami, Y. Matsushima and M. Kimata, Jpn. J. Appl. Phys., 1977, 16, 2131 CrossRef.
- R. A. A. Kubiak, E. H. C. Parker, S. Newstead and J. J. Harris, Appl. Phys. A, 1984, 35, 61–66 CrossRef.
- R. L. Wells, S. R. Aubuchon, S. S. Kher, M. S. Lube and P. S. White, Chem. Mater., 1995, 7, 793–800 CrossRef.
- A. Guzelian, U. Banin, A. Kadavanich, X. Peng and A. Alivisatos, Appl. Phys. Lett., 1996, 69, 1432–1434 CrossRef.
- D. Battaglia and X. Peng, Nano Lett., 2002, 2, 1027–1030 CrossRef CAS.
- X. Peng, J. Wickham and A. P. Alivisatos, J. Am. Chem. Soc., 1998, 120, 5343–5344 CrossRef CAS.
- J. P. Zimmer, S.-W. Kim, S. Ohnishi, E. Tanaka, J. V. Frangioni and M. G. Bawendi, J. Am. Chem. Soc., 2006, 128, 2526–2527 CrossRef CAS.
- R. Xie, K. Chen, X. Chen and X. Peng, Nano Res., 2008, 1, 457–464 CrossRef CAS PubMed.
- H. Bahmani Jalali, S. Sadeghi, M. Sahin, H. Ozturk, C. W. Ow-Yang and S. Nizamoglu, Chem. Mater., 2019, 31, 4743–4747 CrossRef CAS.
- D. Franke, D. K. Harris, L. Xie, K. F. Jensen and M. G. Bawendi, Angew. Chem., 2015, 127, 14507–14511 CrossRef.
- O. T. Bruns, T. S. Bischof, D. K. Harris, D. Franke, Y. Shi, L. Riedemann, A. Bartelt, F. B. Jaworski, J. A. Carr and C. J. Rowlands, Nat. Biomed. Eng., 2017, 1, 1–11 CrossRef.
- M. Kahlweit, Adv. Colloid Interface Sci., 1975, 5, 1–35 CrossRef.
- J. Zhang and D. Zhang, CrystEngComm, 2010, 12, 591–594 RSC.
- J. Zhang and D. Zhang, Chem. Mater., 2010, 22, 1579–1584 CrossRef.
- J. E. Mac Dougall, H. Eckert, G. D. Stucky, N. Herron, Y. Wang, K. Moller, T. Bein and D. Cox, J. Am. Chem. Soc., 1989, 111, 8006–8007 CrossRef CAS.
- H. Uesugi, M. Kita and T. Omata, J. Cryst. Growth, 2015, 416, 134–141 CrossRef CAS.
- A. Das, A. Shamirian and P. T. Snee, Chem. Mater., 2016, 28, 4058–4064 CrossRef CAS.
- M. A. Malik, P. O'Brien and M. Helliwell, J. Mater. Chem., 2005, 15, 1463–1467 RSC.
- M. Green, S. Norager, P. Moriarty, M. Motevalli and P. O'Brien, J. Mater. Chem., 2000, 10, 1939–1943 RSC.
- V. Grigel, D. Dupont, K. De Nolf, Z. Hens and M. D. Tessier, J. Am. Chem. Soc., 2016, 138, 13485–13488 CrossRef CAS PubMed.
- M. D. Tessier, K. De Nolf, D. Dupont, D. Sinnaeve, J. De Roo and Z. Hens, J. Am. Chem. Soc., 2016, 138, 5923–5929 CrossRef CAS.
- A. Buffard, S. Dreyfuss, B. Nadal, H. Heuclin, X. Xu, G. Patriarche, N. Mézailles and B. Dubertret, Chem. Mater., 2016, 28, 5925–5934 CrossRef.
- V. Srivastava, E. M. Janke, B. T. Diroll, R. D. Schaller and D. V. Talapin, Chem. Mater., 2016, 28, 6797–6802 CrossRef.
- V. Srivastava, E. Dunietz, V. Kamysbayev, J. S. Anderson and D. V. Talapin, Chem. Mater., 2018, 30, 3623–3627 CrossRef.
- Z. M. Heiden and A. P. Lathem, Organometallics, 2015, 34, 1818–1827 CrossRef.
- T. J. Trentler, K. M. Hickman, S. C. Goel, A. M. Viano, P. C. Gibbons and W. E. Buhro, Science, 1995, 270, 1791–1794 CrossRef CAS.
- D. Darwan, L. J. Lim, T. Wang, H. Wijaya and Z.-K. Tan, Nano Lett, 2021, 21, 5167–5172 CrossRef CAS.
- K. R. G. Lim, D. Darwan, H. Wijaya, Z. C. Lim, J. Shanmugam, T. Wang, L. J. Lim, W. H. Ang and Z. K. Tan, Adv. Mater. Interfaces, 2020, 7, 2000920 CrossRef CAS.
- H. Wijaya, D. Darwan, X. Zhao, E. W. Y. Ong, K. R. G. Lim, T. Wang, L. J. Lim, K. H. Khoo and Z. K. Tan, Adv. Funct. Mater., 2020, 30, 1906483 CrossRef CAS.
- T. Zhao, N. Oh, D. Jishkariani, M. Zhang, H. Wang, N. Li, J. D. Lee, C. Zeng, M. Muduli and H.-J. Choi, J. Am. Chem. Soc., 2019, 141, 15145–15152 CrossRef CAS.
- J. Leemans, K. C. Dumbgen, M. M. Minjauw, Q. Zhao, A. Vantomme, I. Infante, C. Detavernier and Z. Hens, J. Am. Chem. Soc., 2021, 143, 4290–4301 CrossRef CAS PubMed.
- R. Tietze, R. Panzer, T. Starzynski, C. Guhrenz, F. Frenzel, C. Würth, U. Resch-Genger, J. J. Weigand and A. Eychmüller, Part. Part. Syst. Charact., 2018, 35, 1800175 CrossRef.
- M. Ginterseder, D. Franke, C. F. Perkinson, L. Wang, E. C. Hansen and M. G. Bawendi, J. Am. Chem. Soc., 2020, 142, 4088–4092 CrossRef CAS.
- D. Zhu, F. Bellato, H. Bahmani Jalali, F. Di Stasio, M. Prato, Y. P. Ivanov, G. Divitini, I. Infante, L. De Trizio and L. Manna, J. Am. Chem. Soc., 2022, 144, 10515–10523 CrossRef PubMed.
- J. Leemans, V. Pejović, E. Georgitzikis, M. Minjauw, A. B. Siddik, Y. H. Deng, Y. Kuang, G. Roelkens, C. Detavernier and I. Lieberman, Adv. Sci., 2022, 9, 2200844 CrossRef.
- Z.-L. Shen, S.-Y. Wang, Y.-K. Chok, Y.-H. Xu and T.-P. Loh, Chem. Rev., 2013, 113, 271–401 CrossRef PubMed.
- L. Waterworth and I. Worrall, J. Chem. Soc. D, 1971, 569 RSC.
- J. Yadav, A. Antony, J. George and B. V. S. Reddy, Eur. J. Org. Chem., 2010, 591–605 CrossRef CAS.
-
S. Kudera and L. Manna, Colloidal Foundations of Nanoscience, Elsevier, 2022, pp. 85–123 DOI:10.1016/B978-0-12-822089-4.00009-X.
- A. M. Jawaid, S. Chattopadhyay, D. J. Wink, L. E. Page and P. T. Snee, ACS Nano, 2013, 7, 3190–3197 CrossRef CAS PubMed.
- R. Xie, Z. Li and X. Peng, J. Am. Chem. Soc., 2009, 131, 15457–15466 CrossRef CAS.
- S. Tamang, S. Lee, H. Choi and S. Jeong, Chem. Mater., 2016, 28, 8119–8122 CrossRef CAS.
- T. Kim, S. Park and S. Jeong, Nat. Commun., 2021, 12, 1–8 CrossRef PubMed.
- S. Kan, T. Mokari, E. Rothenberg and U. Banin, Nat. Mater., 2003, 2, 155–158 CrossRef CAS.
- V. F. Puntes, K. M. Krishnan and A. P. Alivisatos, Science, 2001, 291, 2115–2117 CrossRef.
- X. Peng, L. Manna, W. Yang, J. Wickham, E. Scher, A. Kadavanich and A. P. Alivisatos, Nature, 2000, 404, 59–61 CrossRef PubMed.
- L. Manna, E. C. Scher and A. P. Alivisatos, J. Am. Chem. Soc., 2000, 122, 12700–12706 CrossRef.
- T. Kim, Y. Kim, S. Park, K. Park, Z. Wang, S. H. Oh, S. Jeong and D. Kim, Adv. Mater., 2022, 34, 2110665 CrossRef PubMed.
- S. Kan, A. Aharoni, T. Mokari and U. Banin, Faraday Discuss., 2004, 125, 23–38 RSC.
- Q. Hang, F. Wang, P. D. Carpenter, D. Zemlyanov, D. Zakharov, E. A. Stach, W. E. Buhro and D. B. Janes, Nano Lett., 2008, 8, 49–55 CrossRef CAS.
- D. D. Fanfair and B. A. Korgel, Cryst. Growth Des., 2005, 5, 1971–1976 CrossRef CAS.
- F. Wang, H. Yu, S. Jeong, J. M. Pietryga, J. A. Hollingsworth, P. C. Gibbons and W. E. Buhro, ACS Nano, 2008, 2, 1903–1913 CrossRef CAS PubMed.
- R. E. Bailey and S. Nie, J. Am. Chem. Soc., 2003, 125, 7100–7106 CrossRef CAS.
- W. K. Bae, L. A. Padilha, Y.-S. Park, H. McDaniel, I. Robel, J. M. Pietryga and V. I. Klimov, ACS Nano, 2013, 7, 3411–3419 CrossRef CAS.
- Z. Liu, J. Zito, M. Ghini, L. Goldoni, M. Prato, H. Bahmani Jalali, I. Infante, L. De Trizio and L. Manna, Nano Lett., 2022, 22, 8567–8573 CrossRef CAS PubMed.
- J. P. Park, J.-j Lee and S.-W. Kim, J. Am. Chem. Soc., 2016, 138, 16568–16571 CrossRef CAS.
- S.-W. Kim, S. Sujith and B. Y. Lee, Chem. Commun., 2006, 4811–4813 RSC.
- S.-W. Kim, J. P. Zimmer, S. Ohnishi, J. B. Tracy, J. V. Frangioni and M. G. Bawendi, J. Am. Chem. Soc., 2005, 127, 10526–10532 CrossRef.
- H. Li, M. Zanella, A. Genovese, M. Povia, A. Falqui, C. Giannini and L. Manna, Nano Lett., 2011, 11, 4964–4970 CrossRef.
- B. J. Beberwyck and A. P. Alivisatos, J. Am. Chem. Soc., 2012, 134, 19977–19980 CrossRef.
- B. J. Beberwyck, Y. Surendranath and A. P. Alivisatos, J. Phys. Chem. C, 2013, 117, 19759–19770 CrossRef.
- C.-J. Li, P. Li, K. Wang and E. E. Molina, AIMS Energy, 2014, 2, 133–157 Search PubMed.
- D. C. Tripathi, L. Asor, G. Zaharoni, U. Banin and N. Tessler, J. Phys. Chem. C, 2019, 123, 18717–18725 CrossRef CAS.
- Y. Amit, H. Eshet, A. Faust, A. Patllola, E. Rabani, U. Banin and A. I. Frenkel, J. Phys. Chem. C, 2013, 117, 13688–13696 CrossRef CAS.
- J. Liu, Y. Amit, Y. Li, A. M. Plonka, S. Ghose, L. Zhang, E. A. Stach, U. Banin and A. I. Frenkel, Chem. Mater., 2016, 28, 8032–8043 CrossRef CAS.
- C. R. Kagan, E. Lifshitz, E. H. Sargent and D. V. Talapin, Science, 2016, 353, 653 CrossRef PubMed.
- Y. W. Cao and U. Banin, Angew. Chem., Int. Ed., 1999, 38, 3692–3694 CrossRef CAS.
- B. Chen, D. Li and F. Wang, Small, 2020, 16, 2002454 CrossRef CAS PubMed.
- Y. Cao and U. Banin, J. Am. Chem. Soc., 2000, 122, 9692–9702 CrossRef CAS.
- Y.-W. Cao, J. Aksenton, V. Soloviev and U. Banin, MRS Online Proc. Libr., 1999, 571, 75 CrossRef.
- A. Aharoni, T. Mokari, I. Popov and U. Banin, J. Am. Chem. Soc., 2006, 128, 257–264 CrossRef.
- J. Zhang, R. Li, W. Sun, Q. Wang, X. Miao and D. Zhang, J. Mater. Chem. C, 2014, 2, 4442–4448 RSC.
- H. Wijaya, D. Darwan, K. R. G. Lim, T. Wang, K. H. Khoo and Z.-K. Tan, Chem. Mater., 2019, 31, 2019–2026 CrossRef.
- M. J. Enright, D. Jasrasaria, M. M. Hanchard, D. R. Needell, M. E. Phelan, D. Weinberg, B. E. McDowell, H.-W. Hsiao, H. Akbari and M. Kottwitz, J. Phy. Chem. C, 2022, 126, 7576–7587 CrossRef CAS.
- S.-W. Kim, J. P. Zimmer, S. Ohnishi, J. B. Tracy, J. V. Frangioni and M. G. Bawendi, J. Am. Chem. Soc., 2005, 127, 10526–10532 CrossRef CAS.
- R. Xie and X. Peng, Angew. Chem., Int. Ed., 2008, 47, 7677–7680 CrossRef CAS.
- L. K. Sagar, G. Bappi, A. Johnston, B. Chen, P. Todorović, L. Levina, M. I. Saidaminov, F. P. García de Arquer, D.-H. Nam, M.-J. Choi, S. Hoogland, O. Voznyy and E. H. Sargent, Chem. Mater., 2020, 32, 7703–7709 CrossRef CAS.
- N. Tessler, Science, 2002, 295, 1506–1508 CrossRef PubMed.
- D. Steiner, A. Aharoni, U. Banin and O. Millo, Nano Lett, 2006, 6, 2201–2205 CrossRef PubMed.
- U. Banin, Y. Cao, D. Katz and O. Millo, Nature, 1999, 400, 542–544 CrossRef.
- M. Califano, ACS Nano, 2009, 3, 2706–2714 CrossRef.
- R. D. Schaller, J. M. Pietryga and V. I. Klimov, Nano Lett, 2007, 7, 3469–3476 CrossRef CAS PubMed.
- J. Pijpers, E. Hendry, M. Milder, R. Fanciulli, J. Savolainen, J. L. Herek, D. Vanmaekelbergh, S. Ruhman, D. Mocatta and D. Oron, J. Phys. Chem. C, 2008, 112, 4783–4784 CrossRef CAS.
- J. J. Pijpers, M. T. Milder, C. Delerue and M. Bonn, J. Phys. Chem. C, 2010, 114, 6318–6324 CrossRef CAS.
- A. P. Spencer, W. K. Peters, N. R. Neale and D. M. Jonas, J. Phys. Chem. C, 2018, 123, 848–858 CrossRef.
- J. Pijpers, E. Hendry, M. Milder, R. Fanciulli, J. Savolainen, J. L. Herek, D. Vanmaekelbergh, S. Ruhman, D. Mocatta and D. Oron, J. Phys. Chem. C, 2007, 111, 4146–4152 CrossRef CAS.
- V. Klimov, D. McBranch, C. Leatherdale and M. Bawendi, Phys. Rev. B, 1999, 60, 13740 CrossRef CAS.
- M. Ben-Lulu, D. Mocatta, M. Bonn, U. Banin and S. Ruhman, Nano Lett., 2008, 8, 1207–1211 CrossRef CAS.
-
V. Abakumov, V. I. Perel and I. Yassievich, Nonradiative recombination in semiconductors, Elsevier, 1991 Search PubMed.
- Y. Li, T. Ding, X. Luo, Z. Chen, X. Liu, X. Lu and K. Wu, Nano Res., 2019, 12, 619–623 CrossRef CAS.
- Y.-S. Park, J. Roh, B. T. Diroll, R. D. Schaller and V. I. Klimov, Nat. Rev. Mater., 2021, 6, 382–401 CrossRef CAS.
- V. I. Klimov, Science, 2000, 290, 314–317 CrossRef CAS PubMed.
- F. Fan, O. Voznyy, R. P. Sabatini, K. T. Bicanic, M. M. Adachi, J. R. McBride, K. R. Reid, Y.-S. Park, X. Li and A. Jain, Nature, 2017, 544, 75–79 CrossRef CAS PubMed.
- V. I. Klimov, S. A. Ivanov, J. Nanda, M. Achermann, I. Bezel, J. A. McGuire and A. Piryatinski, Nature, 2007, 447, 441–446 CrossRef PubMed.
- J. Maskoun, N. Gheshlaghi, F. Isik, S. Delikanli, O. Erdem, E. Y. Erdem and H. V. Demir, Adv. Mater., 2021, 33, 2007131 CrossRef.
- P. Anikeeva, C. Madigan, J. E. Halpert, M. Bawendi and V. Bulović, Phys. Rev. B: Condens. Matter Mater. Phys., 2008, 78, 085434 CrossRef.
- C. Zou, C. Zhang, Y.-H. Kim, L. Y. Lin and J. M. Luther, ACS Photonics, 2021, 8, 386–404 CrossRef.
- Y.-H. Won, O. Cho, T. Kim, D.-Y. Chung, T. Kim, H. Chung, H. Jang, J. Lee, D. Kim and E. Jang, Nature, 2019, 575, 634–638 CrossRef CAS.
- A. Eich, T. C. Spiekermann, H. Gehring, L. Sommer, J. R. Bankwitz, P. P. Schrinner, J. A. Preuß, S. Michaelis de Vasconcellos, R. Bratschitsch and W. H. Pernice, ACS Photonics, 2022, 9, 551–558 CrossRef CAS.
- V. I. Klimov, A. A. Mikhailovsky, D. McBranch, C. A. Leatherdale and M. G. Bawendi, Science, 2000, 287, 1011–1013 CrossRef CAS PubMed.
- R. E. Correa, E. A. Dauler, G. Nair, S. H. Pan, D. Rosenberg, A. J. Kerman, R. J. Molnar, X. Hu, F. Marsili, V. Anant, K. K. Berggren and M. G. Bawendi, Nano Lett, 2012, 12, 2953–2958 CrossRef CAS PubMed.
- T. S. Bischof, R. E. Correa, D. Rosenberg, E. A. Dauler and M. G. Bawendi, Nano Lett., 2014, 14, 6787–6791 CrossRef CAS.
- P. Frantsuzov, M. Kuno, B. Janko and R. A. Marcus, Nat. Phys., 2008, 4, 519–522 Search PubMed.
- M. Bruchez Jr., Science, 1998, 281, 2013–2016 CrossRef CAS.
- W. Cai, D.-W. Shin, K. Chen, O. Gheysens, Q. Cao, S. X. Wang, S. S. Gambhir and X. Chen, Nano Lett., 2006, 6, 669–676 CrossRef PubMed.
- W. C. W. Chan and S. Nie, Science, 1998, 281, 2016–2018 CrossRef PubMed.
- H. Bahmani Jalali, O. Karatum, R. Melikov, U. M. Dikbas, S. Sadeghi, E. Yildiz, I. B. Dogru, G. Ozgun Eren, C. Ergun and A. Sahin, Nano Lett., 2019, 19, 5975–5981 CrossRef PubMed.
- M. Han, H. B. Jalali, E. Yildiz, M. H. Qureshi, A. Şahin and S. Nizamoglu, Commun. Mater., 2021, 2, 19 CrossRef.
- O. Karatum, M. M. Aria, G. O. Eren, E. Yildiz, R. Melikov, S. B. Srivastava, S. Surme, I. B. Dogru, H. Bahmani Jalali and B. Ulgut, Front. NeuroSci., 2021, 15, 652608 CrossRef.
- F. Ding, Y. Zhan, X. Lu and Y. Sun, Chem. Sci., 2018, 9, 4370–4380 RSC.
- Y. T. Lim, S. Kim, A. Nakayama, N. E. Stott, M. G. Bawendi and J. V. Frangioni, Mol. Imaging, 2003, 2, 50 CrossRef CAS.
- C. R. Kagan and C. B. Murray, Nat. Nanotechnol., 2015, 10, 1013–1026 CrossRef CAS PubMed.
- S. M. Geyer, P. M. Allen, L.-Y. Chang, C. R. Wong, T. P. Osedach, N. Zhao, V. Bulovic and M. G. Bawendi, ACS Nano, 2010, 4, 7373–7378 CrossRef CAS.
- S. Heedt, I. Otto, K. Sladek, H. Hardtdegen, J. Schubert, N. Demarina, H. Lüth, D. Grützmacher and T. Schäpers, Nanoscale, 2015, 7, 18188–18197 RSC.
- M. Soreni-Harari, D. Mocatta, M. Zimin, Y. Gannot, U. Banin and N. Tessler, Adv. Funct. Mater., 2010, 20, 1005–1010 CrossRef CAS.
- W. Liu, J.-S. Lee and D. V. Talapin, J. Ame. Chem. Soc, 2013, 135, 1349–1357 CrossRef.
- D. C. Oertel, M. G. Bawendi, A. C. Arango and V. Bulović, Appl. Phys. Lett., 2005, 87, 213505 CrossRef.
- J.-S. Lee, M. V. Kovalenko, J. Huang, D. S. Chung and D. V. Talapin, Nat. Nanotechnol., 2011, 6, 348–352 CrossRef.
- J.-H. Choi, A. T. Fafarman, S. J. Oh, D.-K. Ko, D. K. Kim, B. T. Diroll, S. Muramoto, J. G. Gillen, C. B. Murray and C. R. Kagan, Nano Lett., 2012, 12, 2631–2638 CrossRef.
- D. V. Talapin, J.-S. Lee, M. V. Kovalenko and E. V. Shevchenko, Chem. Rev., 2010, 110, 389–458 CrossRef CAS PubMed.
- X. Zhao, L. J. Lim, S. S. Ang and Z. K. Tan, Adv. Mater., 2022, 2206409 CrossRef CAS.
- M. De Franco, D. Zhu, A. Asaithambi, M. Prato, E. Charalampous, S. Christodoulou, I. Kriegel, L. De Trizio, L. Manna and H. Bahmani Jalali, ACS Energy Lett., 2022, 7, 3788–3790 CrossRef CAS PubMed.
- S. Yang, D. Prendergast and J. B. Neaton, Nano Lett., 2012, 12, 383–388 CrossRef CAS.
- P. R. Brown, D. Kim, R. R. Lunt, N. Zhao, M. G. Bawendi, J. C. Grossman and V. Bulovic, ACS Nano, 2014, 8, 5863–5872 CrossRef CAS.
- O. Karatum, H. B. Jalali, S. Sadeghi, R. Melikov, B. Srivastava and S. Nizamoglu, ACS Photonics, 2019, 6, 939–946 CrossRef CAS.
- G. O. Eren, S. Sadeghi, H. Bahmani Jalali, M. Ritter, M. Han, I. Baylam, R. Melikov, A. Onal, F. Oz and M. Sahin, ACS Appl. Mater. Interfaces, 2021, 13, 32022–32030 CrossRef.
- A. Onal, G. O. Eren, S. Sadeghi, R. Melikov, M. Han, O. Karatum, M. S. Ozer, H. Bahmani Jalali, I. B. Dogru-Yuksel and I. Yilgor, ACS Photonics, 2022, 9, 1304–1314 CrossRef.
- N. Chen, Y. He, Y. Su, X. Li, Q. Huang, H. Wang, X. Zhang, R. Tai and C. Fan, Biomaterials, 2012, 33, 1238–1244 CrossRef PubMed.
- M. Vasilopoulou, H. P. Kim, B. S. Kim, M. Papadakis, A. E. Ximim Gavim, A. G. Macedo, W. Jose da Silva, F. K. Schneider, M. A. Mat Teridi, A. G. Coutsolelos and A. R. bin Mohd Yusoff, Nat. Photonics, 2020, 14, 50–56 CrossRef.
- L. Biadala, B. Siebers, Y. Beyazit, M. D. Tessier, D. Dupont, Z. Hens, D. R. Yakovlev and M. Bayer, ACS Nano, 2016, 10, 3356–3364 CrossRef CAS.
- S. Sadeghi, H. Bahmani Jalali, R. Melikov, B. Ganesh Kumar, M. Mohammadi Aria, C. W. Ow-Yang and S. Nizamoglu, ACS Appl. Mater. Interfaces, 2018, 10, 12975–12982 CrossRef CAS PubMed.
- S. Sadeghi, H. B. Jalali, S. B. Srivastava, R. Melikov, I. Baylam, A. Sennaroglu and S. Nizamoglu, iScience, 2020, 23, 101272 CrossRef CAS.
- H. Bahmani Jalali, S. Sadeghi, I. Baylam, M. Han, C. W. Ow-Yang, A. Sennaroglu and S. Nizamoglu, Nano Res., 2021, 14, 1488–1494 CrossRef CAS.
- F. Meinardi, H. McDaniel, F. Carulli, A. Colombo, K. A. Velizhanin, N. S. Makarov, R. Simonutti, V. I. Klimov and S. Brovelli, Nat. Nanotechnol., 2015, 10, 878–885 CrossRef CAS PubMed.
- C. B. Murray, S. Sun, W. Gaschler, H. Doyle, T. A. Betley and C. R. Kagan, IBM J. Res. Dev., 2001, 45, 47–56 CAS.
- J. M. Pietryga, R. D. Schaller, D. Werder, M. H. Stewart, V. I. Klimov and J. A. Hollingsworth, J. Am. Chem. Soc., 2004, 126, 11752–11753 CrossRef CAS PubMed.
- J. E. Murphy, M. C. Beard, A. G. Norman, S. P. Ahrenkiel, J. C. Johnson, P. Yu, O. I. Mićić, R. J. Ellingson and A. J. Nozik, J. Am. Chem. Soc., 2006, 128, 3241–3247 CrossRef CAS.
- M. V. Kovalenko, E. Kaufmann, D. Pachinger, J. Roither, M. Huber, J. Stangl, G. Hesser, F. Schäffler and W. Heiss, J. Am. Chem. Soc., 2006, 128, 3516–3517 CrossRef.
- L. K. Sagar, W. Walravens, J. Maes, P. Geiregat and Z. Hens, J. Phys. Chem. C, 2017, 121, 13816–13822 CrossRef.
- V. Srivastava, W. Liu, E. M. Janke, V. Kamysbayev, A. S. Filatov, C. Sun, B. Lee, T. Rajh, R. D. Schaller and D. V. Talapin, Nano Lett., 2017, 17, 2094–2101 CrossRef.
|
This journal is © The Royal Society of Chemistry 2022 |