DOI:
10.1039/D1BM01710A
(Paper)
Biomater. Sci., 2022,
10, 294-305
Hypoxia-alleviated sonodynamic therapy based on a hybrid protein oxygen carrier to enhance tumor inhibition†
Received
7th November 2021
, Accepted 17th November 2021
First published on 2nd December 2021
Abstract
Sonodynamic therapy (SDT) is a highly attractive therapy due to its advantages of being non-invasive and having good penetration depth, but tumor hypoxia extremely restricts its therapeutic effect. Here, a novel oxygen-enhanced hybrid protein nanosonosensitizer system (MnPcS@HPO) is designed using human serum albumin (HSA) and hemoglobin (Hb) through disulfide reconfiguration, followed by encapsulating Mn-phthalocyanine (MnPcS), aiming to develop O2 self-supplementing nanoparticles (NPs) for enhanced SDT. Benefitting from the O2-carrying ability of Hb and the tumor-targeting property of HSA, the MnPcS@HPO NPs are able to target tumor sites and alleviate hypoxia. Meanwhile, as a sonosensitizer, MnPcS is excited under US irradiation and activates dioxygen to generate abundant singlet oxygen (1O2), resulting in oxidative damage of tumor cells. Guided by photoacoustic and magnetic resonance dual-modal imaging, the MnPcS@HPO NPs alleviate tumor hypoxia and achieve good SDT efficiency for suppressing tumor growth. This work presents a novel insight into enhanced SDT antitumor activity through natural protein-mediated tumor microenvironment improvement.
1 Introduction
With the fast development of theranostic medicine, various therapeutic strategies have been highly studied for oncotherapy.1–3 Because of their tumor specificity and few side effects to normal tissues, the external noninvasive therapeutic strategies are believed to be the most promising.4,5 Sonodynamic therapy (SDT), which induces deep-seated tumor cell apoptosis/necrosis under noninvasive ultrasound (US) irradiation, has shown much more potential for tumor treatment than other therapeutic models.6–8 The success of SDT highly depends on the properties of the sonosensitizers and the oxygen supply.9–11 Under US treatment, sonosensitizers are activated and then excite surrounding O2 molecules to generate reactive oxygen species (ROS), which results in tumor cell oxidative apoptosis and necrosis.12–14 But one of the typical characteristics of the tumor microenvironment is hypoxia because of the insufficient O2 supply in solid tumors.15,16 Importantly, tumor hypoxia is highly detrimental to the efficacy of O2-mediated SDT.17,18 Moreover, the O2 consumption of SDT might further aggravate tumor hypoxia and in turn restrict the SDT effect. Therefore, enhancing the O2 supply to alleviate the hypoxic microenvironment in tumors is significantly important for improving the efficiency of O2-dependant therapeutic strategies, including SDT.
To overcome the tumor hypoxia, many strategies have been proposed.19–21 For instance, a hyperbaric oxygen chamber has been used to force oxygen into tumor regions. But the poor vascular architecture significantly hinders sufficient local oxygen flowing into the tumor.22–24 Perfluorocarbon (PFC) and modified fluorocarbon (FC) have also been demonstrated to realize hypoxia oxygenation through highly effective oxygen delivery, while poor stability and inadequate targeting ability are their potential issues.18,25,26 Recently, some novel oxygen generators (such as MnO2 and TiOP) have been developed by in situ O2 generation inside tumors.27–29 In addition, metal–organic frameworks (MOF) have also been used as carriers to transport O2 directly to tumors.30,31 But these inorganic nanocarriers are controversial due to their potential risk of metabolism. Compared with the inorganic oxygen carriers, hemoglobin (Hb), which is an inner protein in red blood cells, is a natural and endogenous O2 carrier.32 Hb can reversibly bind oxygen molecules and safely transfer O2, and release O2 in hypoxic regions.33 However, in spite of the obvious merits of Hb as an O2 carrier, free blood-circulating Hb always gradually loses its O2-carried ability.34,35 Therefore, it is necessary to devise deliberate designs for enhancing the O2-loading ability of Hb. Human serum albumin (HSA) has been demonstrated to be a good and natural carrier, and can widely bind diverse water-insoluble agents and target tumors mediated by 60 kDa glycoprotein (gp60)/caveolae endothelial transcytosis and binding to SPARC (secreted protein, acidic and rich in cysteine) with excellent biocompatibility and non-toxicity.36 Meanwhile, HSA is also able to stabilize Hb and improve its O2-loading ability, especially when assisted by some catalytic candidates such as metal porphyrins.37,38
Herein, we have designed a HSA–Hb hybrid protein (HP) nanosystem to load the MnPcS compound, producing a nanosonosensitizer (MnPcS@HPO) for enhanced SDT. As shown in Fig. 1, HSA was first reduced by GSH, which could open the disulfide bond (–S–S–) producing sulfhydryl groups (–SH). The reduced HSA is hybridized with Hb sufficiently through –SH conversion to –S–S–, and MnPcS was simultaneously encapsulated in the hybrid protein, achieving MnPcS@HP nanoparticles (NPs). After being dioxygenated, the multifunctional nanosonosensitizer of MnPcS@HPO NPs was obtained. The MnPcS@HPO NPs could highly enhance the SDT antitumor effect through the extra O2-afforded in the tumor site. The in vitro and in vivo therapeutic results showed obvious tumor cell growth inhibition from MnPcS@HPO with great biosafety. This work is the first report to describe hypoxia-alleviated SDT antitumor activity through wrapping Hb in HSA mutants engineered by a MnPcS complex.
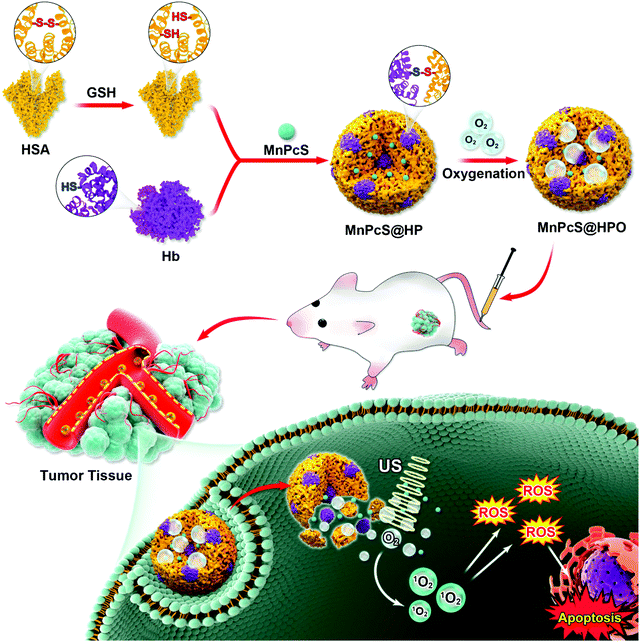 |
| Fig. 1 Illustration of the hybrid protein nanosonosensitizer (MnPcS@HPO) system and the mechanism of MnPcS@HPO-mediated SDT antitumor activity. The –S–S– bonds in HSA are first broken by GSH, and then Hb and MnPcS are added to get MnPcS@HP NPs, which are then oxygenated to achieve MnPcS@HPO NPs. The MnPcS@HPO NPs could be responsively released by GSH overexpressed in the tumor. The released MnPcS could be irradiated by US waves to excite the surrounding O2 to generate ROS and induce tumor cell apoptosis and necrosis. | |
2. Results and discussion
2.1 Preparation and characterization of the MnPcS@HPO NPs
The O2-carrying sonosensitizer nanoparticles were synthesized by a redox synthesis strategy. HSA was first reduced by GSH to generate free sulfhydryl groups (–SH) and adequately hybridized with Hb through disulfide conjugation, during which the MnPcS complex in chloroform (CHCl3) solution was added in. To finally obtain MnPcS@HP NPs, sonication was performed until the solution became clear. The MnPcS@HPO NPs were achieved by charging dioxygen into the solution. Transmission electron microscopy (TEM) was conducted to estimate the successful construction and morphology of the NPs. As revealed in Fig. 2a, the prepared MnPcS@HP NPs were uniformly spherical with an average diameter of around 75 nm. In addition, the elemental mapping image was obtained by SEM. The elements in the MnPcS complex (such as Mn, S, N and C atoms) were all exhibited in the nanoparticles, suggesting the good loading of MnPcS into the Hb carrier (Fig. 2b). Furthermore, the MnPcS loading rate in the MnPcS@HP NPs was also measured by UV–Vis spectrophotometer and calculated to be 84.7%, which suggested good encapsulation.
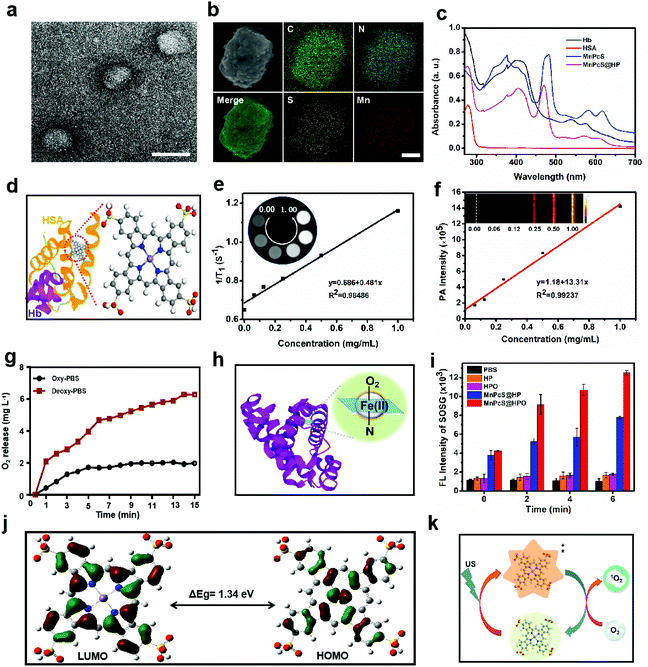 |
| Fig. 2 Characterization and physiochemical properties of the MnPcS@HP NPs. (a) The TEM image of the MnPcS@HP NPs (scale bar = 100 nm), showing the typical spherical shape. (b) The elemental mapping of the MnPcS@HP NPs (scale bar = 25 nm). (c) UV-Vis absorption spectra of MnPcS@HP, indicating the good encapsulation of MnPcS and Hb in HSA. (d) The possible structure model of the HSA-MnPcS complex, in which MnPcS is bound in the hydrophobic slot in HSA. (e) Longitudinal relaxation rates (1/T1) versus the concentration of MnPcS@HP (0, 0.0625, 0.125, 0.25, 0.50 and 1.00 mg mL−1); the T1-weighted MR images of MnPcS@HP in water solution at various concentrations are shown in the inset. (f) The PA signal intensity versus the concentration of MnPcS@HP (0, 0.0625, 0.125, 0.25, 0.50 and 1.00 mg mL−1); the PA images at various concentrations of MnPcS@HP are shown in the inset (λex = 680 nm). (g) Oxygen release profile of the MnPcS@HPO NPs by estimating the dissolved oxygen in various PBS solutions (concentration of 75 μg mL−1). (h) The O2-loaded mode in Hb. (i) 1O2 generation (SOSG as a probe) in the MnPcS@HP solution (concentration of 40 μg mL−1) under US exposure (1.0 W cm−2, 50% duty cycle). (j) Calculations of the orbital distribution of MnPcS and the HOMO–LUMO energy gap. (k) The possible mechanism of the conversion of O2 to 1O2. | |
Dynamic light scattering (DLS) analysis was further executed to explore the monodispersity. Fig. S1† shows the good uniformity with a size of about 75 nm, which is consistent with the results of TEM, and the size was not obviously influenced by either oxygenation or not. Moreover, the dispersion stabilities of the MnPcS@HP NPs were also tested for a week in PBS and fetal bovine serum (FBS) using DLS. From Fig. S2 and S3,† no obvious increase in size occurred in PBS or FBS solution within 7 days, suggesting the good dispersion stability of the nanoparticles. The nanoparticles were then investigated by UV-Vis spectroscopy to explore the effective encapsulation of the components in the MnPcS@HP NPs. From Fig. 2c, the absorption peak at 410 nm suggests the efficient hybridization of Hb in the MnPcS@HP NPs. The absorption peak at 280 nm is the characteristic absorption of HSA. Meanwhile, the MnPcS@HP NPs exhibited the characteristic absorption peaks of the MnPcS complex, where the absorption peak at 500–650 nm represented the Q-bands, while those at 380 nm and 480 nm were the S-bands.
According to relevant references, the effective integration of MnPcS, Hb and HSA might be explained the MnPcS being bound within hydrophobic slots in subdomain IB of HSA with weak axial coordination or salt-bridges between the metal center and basic amino acid ligands, and the recombinant HSA–MnPcS complex is then reassembled with Hb (Fig. 2d).38,39 In addition, the release behavior of the MnPcS@HP NPs was also evaluated in PBS solution (PH = 7.4) and PBS solution with 10 mM glutathione (GSH). Fig. S4† shows that the MnPcS@HP NPs released slowly in PBS with only 30% release at 24 h, while rapid release was observed in PBS with GSH, and almost 60% was released within 1 h. This result indicated that the MnPcS@HP NPs would be stable in circulation in the blood, but the nanoparticles could be released rapidly at the tumor site, since GSH is always overexpressed in tumors.
Mn ions and their derivatives have been developed as T1-MR imaging contrast agents. Therefore, T1-MR imaging of the MnPcS@HP NPs was also estimated in solution with a series of concentrations from 0.00 to 1.00 mg mL−1. Fig. 2e shows that MR imaging was possible at different concentration, and a good linear relationship was observed between concentration and 1/T1 with the r1 relaxivity of 1.323 mM−1 s−1 in solution. In addition, benefitting from the PA properties of porphyrin derivatives, the in vitro PA image of the MnPcS@HP NPs was also measured. Fig. 2f shows that an obvious PA signal was observed and increased as the concentration increased. The PA signal intensities showed a good linear relationship with the concentration of the MnPcS@HP NPs. The MR and PA imaging abilities both indicated that the MnPcS@HP NPs could be used to monitor the in vivo accumulation of the nanoagents.
Efficient O2-carrying and release of the MnPcS@HPO NPs is very important for hypoxic alleviation in tumors. Therefore, the O2 release of the MnPcS@HPO NPs was measured in normal oxygen PBS solution and in deoxygenated PBS solution. As shown in Fig. 2g, there was negligible release in the normal oxygen PBS solution, but rapid release was observed in the deoxygenated PBS solution. This result suggested that Hb in the MnPcS@HPO NPs is a good O2 carrier, in which dioxygen might be bound to the ferrous heme to afford a ferric-superoxo adduct (Fig. 2h).40 The effective O2-loading/release properties provide benefit for enhancing the O2 level in the tumor environment.
As a big ring complex, MnPcS was expected to be activated by US irradiation like porphyrin compounds, which have been widely used as sonosensitizers in SDT due to their unique properties, such as large π-electron conjugated system and catalytic performance. Here, the US-responsive properties of the MnPcS@HPO NPs were explored by detecting the 1O2 generation using SOSG (a singlet oxygen probe). As revealed in Fig. 2i, negligible 1O2 generation was observed in the HP + US and HPO + US groups, while both the MnPcS@HP NPs and MnPcS@HPO NPs could be activated by US to generate 1O2, and the intensity increased over time, indicating that only MnPcS could act as a sonosensitizer. But the MnPcS@HPO NPs excited much more 1O2 generation than the MnPcS@HP NPs, probably due to the sufficient O2 supply from the hybrid protein. To further explore the mechanism of MnPcS-mediated 1O2 generation, density functional theory (DFT) calculations were performed at the Becke three-parameter Lee Yang Parr (B3LYP) level.41 From Fig. 2j, the metal center contributed to the highest occupied molecular orbital (HOMO), and the distribution of the HOMO on the phthalocyanine ring was obviously delocalized onto the metal ion. Meanwhile, the lowest unoccupied molecular orbital (LUMO) was localized on the phthalocyanine ring. The energy gap between the HOMO and LUMO of MnPcS was 1.34 eV, so it is easily excited to the highly excited-state species. Accompanied by the energy level transition of the ground-state and excited-state under US energy irradiation, the surrounding O2 would be excited to 1O2, which might induce tumor cell apoptosis/necrosis (Fig. 2k).36,42
2.2
In vitro SDT effect of the MnPcS@HPO NPs under US treatment
The good O2-supplementation and SDT efficiency of the MnPcS@HPO NPs in solution needed to be demonstrated at the tumor cell level. Before exploring the SDT effect for killing tumor cells, the targeting ability of MnPcS@HP towards 4T1 tumor cells was first detected by confocal laser scanning microscopy (CLSM). From Fig. 3a, after incubation with the MnPcS@HP NPs for different times, the 4T1 cells generated a FL signal, suggesting effective uptake into the 4T1 cells. Moreover, the signal obviously increased with time, indicating the sufficient uptake by tumor cells. Then, the biological safety of the MnPcS@HP NPs for 4T1 tumor cells without US treatment was investigated by a Cell Counting Kit-8 (CCK8) assay. Fig. 3b shows that negligible toxicity occurred to the tumor cells with the concentration below 75 μg mL−1. The SDT efficiency was subsequently measured under US irradiation. From Fig. 3c, there was little damage presented in the cells not treated with US irradiation, whereas obvious cell death occurred both in the MnPcS@HP + US group and the MnPcS@HPO + US group, which were treated by US treatment, suggesting good SDT-mediated cell killing. But the cell death rate in the MnPcS@HPO + US group was much higher (over 75%) than that in the MnPcS@HP + US group (about 55%), probably due to the O2-supplemented SDT efficiency. The FL staining of living/dead cells was subsequently conducted and gave similar results. As revealed in Fig. 3d, the control group without US treatment exhibited mostly live cells (in green), while obvious cell death (in red) was observed both in the MnPcS@HP + US group and the MnPcS@HPO + US group. But obviously, the MnPcS@HPO + US group showed a much stronger red signal, suggesting more cell death.
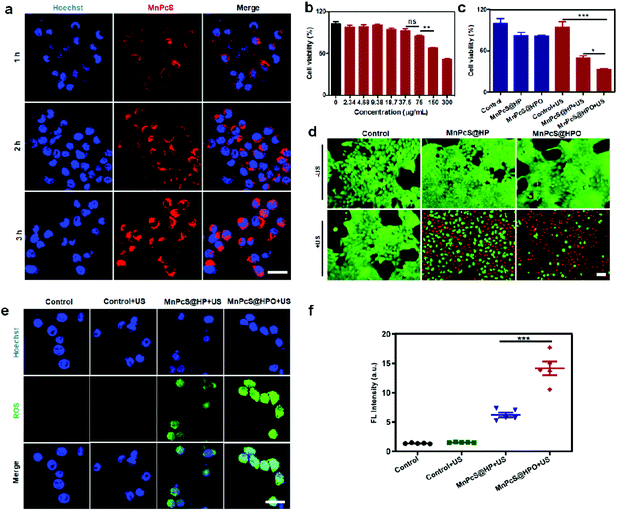 |
| Fig. 3
In vitro SDT efficiency of the MnPcS@HPO NPs. (a) CLSM images of the uptake into 4T1 cells after incubation with MnPcS@HP NPs for different times (MnPcS concentration of 75 μg mL−1, scale bar = 20 μm). (b) The biosafety of MnPcS@HP NPs to 4T1 cells under different concentrations (**p < 0.01). (c) The sonotoxicity of MnPcS@HP NPs and MnPcS@HPO NPs to 4T1 cells (MnPcS concentration of 75 μg mL−1; 1.0 W cm−2, 50% duty cycle; *p < 0.05, ***p < 0.001). (d) The FL images of 4T1 cells after staining with calcein-AM and PI when treated by different methods; viable cells were labeled with calcein-AM (green), and dead/later apoptosis cells were labeled with PI (red) (scale bar = 20 μm). (e) CLSM images of ROS in 4T1 cells stained by DCFH-DA after different treatments (scale bar = 20 μm, US condition: 1.0 W cm−2, 50% duty cycle). (f) The quantitative analysis of the intracellular ROS FL intensity after various treatments (***p < 0.001). | |
The mechanism was explored by measuring the intracellular ROS levels using DCFH-DA as a FL probe. As shown in Fig. 3e, a negligible ROS signal in the US-untreated group and control + US group was observed, while a small ROS signal was generated in the MnPcS@HP + US group, which was probably from MnPcS-mediated SDT. But there was remarkable 1O2 signal observed in the MnPcS@HPO + US group, suggesting O2-enhanced MnPcS-mediated SDT, which would be responsible for tumor cell killing. The semi-quantified ROS intensity was further detected by a Multimode Reader. Fig. 3f shows that the highest ROS intensity existed in the MnPcS@HPO + US group among all the groups, indicating the efficient tumor cell killing by O2-enhanced SDT.
2.3
In vivo accumulation and hypoxia alleviation induced by the MnPcS@HPO NPs
The accumulation of MnPcS@HPO NPs was evaluated using MR imaging (MRI). To detect the in vivo accumulation of MnPcS@HPO NPs, mice bearing 4T1 breast cancer xenografts were used and T1-weighted MRI was performed after intravenous injection of the MnPcS@HPO NPs. Fig. 4a shows that an MRI signal was observed and increased over time. The optimal accumulation signal occurred at 3 h. Moreover, the statistically semi-quantified T1 signal intensity was further analyzed. The signal intensity at 3 h was the highest among the series of time points, which was consistent with the MRI result (Fig. 4b). The effective accumulation of MnPcS@HPO NPs was also assessed by PA imaging (680 nm). Fig. 4c shows that the FL signal changed with time, and the highest signal occurred at 3 h, suggesting the optimal accumulation in the tumor. The semi-quantified PA imaging intensity was also calculated, as shown in Fig. 4d. The maximum PA intensity was observed at 3 h. The above results all suggested that the nanoparticles could effectively target the tumor site.
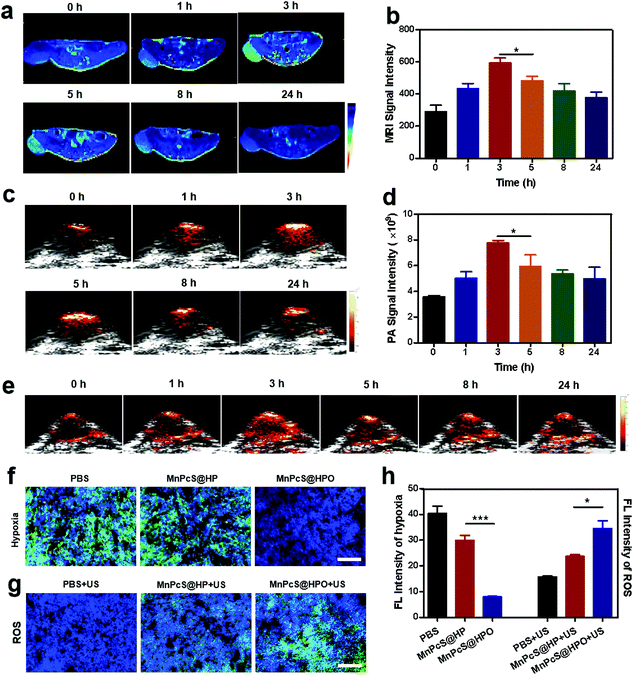 |
| Fig. 4 Evaluation of MnPcS@HPO-mediated tumor hypoxia alleviation (concentration of 600 μg mL−1; US condition: 50% duty cycle, 1.0 W cm−2, 5 min). (a) In vivo MR imaging in the tumor region over time after intravenous injection of MnPcS@HPO NPs, and (b) the semi-quantified MRI signal intensity analysis (*p < 0.05). (c) In vivo PA images in the tumor region over time (λ = 680 nm) after intravenous injection of MnPcS@HPO NPs and (d) the corresponding signal intensity values (*p < 0.05). (e) HbO2 PA imaging in the tumor region over time after intravenous injection of MnPcS@HPO NPs (λ = 850 nm). (f) Hypoxia measurement of 4T1 tumor tissues after staining with a hypoxia probe (green) and nuclear dye (blue) (scale bar = 100 μm). (g) In vivo1O2 examination in tumor tissues after different treatments (scale bar = 100 μm). (h) The relevant semi-quantified hypoxia/1O2 FL intensity (*p < 0.05; ***p < 0.001). | |
With the effective accumulation of MnPcS@HPO NPs, the O2-enhancement in the tumor induced by the MnPcS@HPO NPs was determined by measuring the oxygenated Hb (HbO2) in the tumor vessels using PA imaging (850 nm). As shown in Fig. 4e, after the nanoparticles were intravenously injected, the HbO2 signal increased with time, and the maximum signal occurred at 3 h, which was similar to the accumulation of MnPcS@HPO NPs, suggesting the highest HbO2 concentration at 3 h in the tumor. Moreover, the hypoxia alleviation was further analyzed by a staining assay of tumor slices at 3 h after intravenous injection of the MnPcS@HPO NPs. Compared with the obvious hypoxic signal (in green) in the tumors in other groups, the negligible green FL signal in the MnPcS@HPO group indicated the hypoxia relief due to the effective O2 afforded from the hybrid protein accumulated in the tumor (Fig. 4f).
Hypoxia alleviation is significantly important for SDT anticancer treatment, since the excited sonosensitizers would activate the surrounding O2 molecules to convert to 1O2. Therefore, the 1O2 generation was studied by staining tumor slices with a fluorescent probe (SOSG) after treatment by US irradiation. As shown in Fig. 4g, a large amount of 1O2 signal (in green) was observed in the MnPcS@HPO NPs group, while the signal was slight in the MnPcS@HP NPs group and negligible in the PBS group. The FL signal intensities presenting hypoxic alleviation and 1O2 generation were semi-quantified. Fig. 4h shows that significant hypoxia alleviation and 1O2 enhancement both occurred in the tumor in the MnPcS@HPO group. The above results suggested that MnPcS@HPO NPs could increase the O2 concentration in the tumor and then MnPcS-mediated SDT could convert O2 to generate 1O2, which might induce tumor cell apoptosis and necrosis.
2.4
In vivo oxygen-enhanced SDT efficiency of MnPcS@HPO NPs against tumors
Efficient O2 supply and 1O2 generation in the tumor indicated the good SDT effect for tumor growth inhibition. To investigate the SDT effect of the MnPcS@HPO NPs, mice bearing 4T1 tumors were randomly divided into four treatment groups: PBS group, PBS + US group, MnPcS@HP + US group and MnPcS@HPO + US group. US irradiation was performed at 3 h after treatment with the different methods. The therapeutic effect of each group was evaluated by recording the tumor volume. As shown in Fig. 5a, the PBS group and PBS + US group both exhibited rapid growth of the tumors without any influence of US treatment. But the MnPcS@HP + US group and MnPcS@HPO + US group showed tumor growth inhibition. Compared with the tumor growth suppression from the MnPcS@HP + US group, the MnPcS@HPO + US group exhibited much higher excellent inhibition efficiency, suggesting the better SDT effect of MnPcS after oxygenation. This result was also identified through the excised tumor weights at the end of the treatment of 20 days. Fig. 5b shows that the lowest weight of the tumors was observed in the MnPcS@HPO + US group among all the groups. The good SDT effect of the MnPcS@HPO + US group was further evaluated intuitively through the excised tumor photographs. From Fig. 5c, the tumors treated with MnPcS@HPO + US were obviously smaller than those of the other groups.
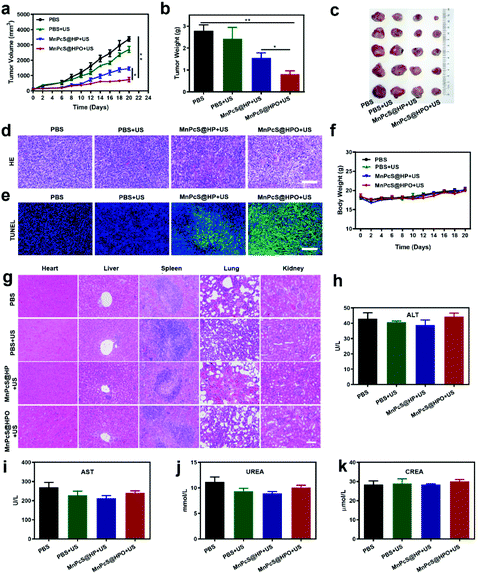 |
| Fig. 5
In vivo SDT effect of the MnPcS@HPO NPs and their biosafety (concentration of 600 μg mL−1; US condition: 50% duty cycle, 1.0 W cm−2, 5 min). (a) Tumor volume curve during the treatment (*p < 0.05, **p < 0.01). (b) The isolated tumor weight (*p < 0.05, **p < 0.01). (c) The photographs of excised tumors at the end of the observation. (d and e) The section photomicrographs stained by H&E (d) and TUNEL (e) of the tumor tissues. Scale bar = 100 μm. (f) The body weight of the mice during the treatment process. (g) The organ section photomicrographs after staining with H&E to evaluate the biosafety. (h and i) The biosafety evaluation of the MnPcS@HPO NPs through serum level analysis (ALT and AST for liver function) and (j and k) serum level analysis (UREA and CREA for renal function) (n = 5). | |
Tumor sections were stained with H&E to evaluate the tumor inhibition mechanism. As revealed in Fig. 5d, no obvious necrosis occurred in the control group and control + US group. Some cell apoptosis/necrosis was observed in the MnPcS@HP + US group, but significant apoptosis and necrosis were observed in the MnPcS@HPO + US group due to the O2-enhanced SDT effect. In addition, a TUNEL assay was further carried out to check the necrosis and apoptosis of the tumor cells. From Fig. 5e, the most significant apoptosis and necrosis occurred in the MnPcS@HPO + US group, compared with the other groups, which demonstrated again the highly enhanced SDT antitumor effect of the MnPcS@HPO NPs compared to the MnPcS@HP NPs.
Biosafety is very important for a therapeutic agent. Here, the biosafety of the MnPcS@HPO + US was first estimated through recording the weight of the mice. Fig. 5f shows that there was no obvious change in weight during the treatment, suggesting the good biosafety of the MnPcS@HP/MnPcS@HPO NPs. A biosafety assessment was then conducted by H&E staining of the mice organs. From Fig. 5g, no obvious damage was observed in the various organs compared with the PBS group, indicating the negligible adverse effects. Also, serum alanine aminotransferase/aspartate aminotransferase (ALT/AST) and creatinine/urea (CREA/UREA) were assayed to analyze the liver and renal damage. Compared with the PBS group, there was no significant difference in the MnPcS@HP/MnPcS@HPO + US group (Fig. 5h–k). All of the above results indicated that MnPcS@HPO-mediated SDT was effective and safe.
3. Conclusion
In summary, we have successfully developed a novel O2-enhanced sonosensitizer nanosystem (MnPcS@HPO) that is simultaneously decorated with metallophthalocyanine and a hybrid protein. The hybrid protein was deliberately used not only as an active targeting carrier but also as an O2-supply system for tumor hypoxia alleviation. Guided by MR and PA imaging, the MnPcS@HPO NPs were monitored to trace their accumulation in the tumor. The effective enrichment of the MnPcS@HPO NPs highly alleviated hypoxia in the tumor microenvironment without any toxicity. Meanwhile, acting as a sonosensitizer, MnPcS was excited to induce 1O2 generation under the action of US irradiation, which induced tumor cell killing. Overall, this system provided an abundance of O2 for SDT-mediated antitumor activity. Considering the excellent biosafety, tumor targeting and inherent O2-loading behavior, hybrid protein encapsulating therapeutic agents could be used not only for SDT antitumor therapy, as illustrated in this work, but also for other O2-based therapies, such as chemotherapy, photodynamic therapy and immune checkpoint blockade antibodies.
4. Experimental section
4.1 Chemicals
Human serum albumin (HSA), glutathione (GSH) and 2′,7′-dichlorofluorescin diacetate (DCFH-DA) were purchased from Sigma-Aldrich (US). Bovine hemoglobin (Hb) was obtained from Hefei Bomei Biotechnology (CHN). Chloroform was purchased from Beijing Chemical Reagent Ltd (CHN). MnPcS was obtained from Yajie Technology Co., Ltd (Huai'an, CHN). The Hypoxyprobe-1 Plus kit was bought from Hypoxyprobe Inc. (USA). Singlet oxygen sensor green (SOSG) and Hoechst 33342 were bought from Thermo Fisher Scientific (USA). Phosphate buffered saline (PBS), fetal bovine serum (FBS), Dulbecco's modified Eagle's medium (DMEM), penicillin–streptomycin and 0.25% (w/v) trypsin solution were purchased from Gibco Life Technologies (USA). Calcein-AM/PI were provided by Invitrogen (USA). Cell counting kit-8 (CCK8) was obtained from Dojindo. All other chemicals used in this study were of analytical reagent grade and used without further purification. Superpure water (18.25 MΩ cm, 25 °C) was used to prepare all solutions. All the chemicals were purchased from commercial sources and used without further purification.
4.2 Animals
BALB/c nude mice were purchased from Vital River Animal Technology Co. Ltd (Beijing, CHN), and were maintained under aseptic conditions in a small animal isolator. The protocols were proposed and given permission by the Animal Care and Use Committee (Shenzhen Institutes of Advanced Technology, Chinese Academy of Sciences).
4.3 Cell culture
The breast cancer line 4T1 was obtained from the American Type Culture Collection (USA). Cells in all experiments were cultured in 1640 medium supplemented with 10% (v/v) fetal bovine serum and 1% streptomycin/penicillin at 37 °C in a 5% CO2 humidified incubator.
4.4 Formulation of the MnPcS@HPO NPs
MnPcS@HPO NPs were first prepared according to a previously described method with some modifications.37 Briefly, 1 mL GSH solution (20 mg mL−1) was added into HSA solution (4 mL, 10 mg mL−1) and shaken on a rotary shaker (37 °C, 170 rpm) for 1 h. Then, dialysis was used to remove the GSH (membrane cutoff MW: 3.5 kDa, 12 h). 8 mg Hb was mixed with the above HSA solution, and then 200 μL MnPcS (5 mg mL−1 chloroform solution) was added. The suspension was kept on an ultrasonic cell disruptor (30% frequency, 5 min) to form the MnPcS@HP NPs. The nanoparticles were purified three times using ultrapure water on an Amicon ultra-4 centrifugal filter (cutoff MW: 100 kDa, 4000 rpm) and through a 220 nm polycarbonate membrane. Then, the MnPcS@HP NPs were sealed and stored in a 4 °C refrigerator. The MnPcS@HPO NPs were freshly obtained by charging a pure oxygen stream at 2 L min−1 for 10 min, before MnPcS@HPO needed to be used in the relevant experiments. The particles were examined by TEM (FEI Tecnai G2 F20 S-Twin, USA), DLS analysis (Zetasizer Nano ZS, Malvern, USA), and UV–Vis absorption (Lambda25, Perkin-Elmer, USA). The atom mapping was achieved on the scanning electron microscope (SEM, Supra 55 Sapphire, Zeiss, GER) and energy spectrometer (Oxford X-Max, Oxford Instruments, UK).
4.5 The encapsulation rate and release efficiency detection
The MnPcS encapsulation efficiency (EE) of the MnPcS@HP was determined using a UV–Vis spectrophotometer according to the absorption of MnPcS at 636 nm and calculated using the following equation: EE = (minitial − msupernatant)/minitial × 100%. The amount of MnPcS was calculated according to the MnPcS calibration curve. To detect the MnPcS release, 1 mL of 1.8 mg mL−1 MnPcS@HP solution was diluted in 1 mL of PBS solution with or without 10 mM GSH on a shaker at 37 °C and then centrifuged (4000 rpm, 5 min) at different time points (0, 0.5, 1, 2, 3, 4, 5, 6, 7, 18, and 24 h). The precipitate and separated liquid were collected and dissolved in DMSO/H2O solution (vH2O
:
vDMSO = 1
:
1). The release rate was obtained according to the concentration analyzed on the UV–Vis spectrophotometer.
4.6 O2 release detection from the MnPcS@HPO NPs
To measure the oxygen release of the MnPcS@HPO NPs in deoxy/oxy-PBS solution, 4 mL PBS was firstly deoxygenated using an ultrasonic cleaner (50–60 Hz) for 30 minutes or oxygenated by pure oxygen stream (2 L min−1) for 10 min. Then, 1 mL ethyl acetate was added to seal the PBS. After this, 1 mL MnPcS@HPO NPs (75 μg mL−1) was added to the bottom of the PBS solution. Oxygen release was measured by a dissolved oxygen meter (Shanghai, REX Instrument, CHN).
4.7
In vitro/in vivo MR and PA imaging
T
1-Weighted in vitro magnetic resonance (MR) images were scanned under a 3.0 T clinical MR scanner (Erlangen, GER). The signal intensity of all T1-weighted MR images was measured in the region of interest (ROI). A series of concentrations of MnPcS complex were measured to calculate the relaxation rates r1 (1/T1). To evaluate the in vivo accumulation of the MnPcS@HPO NPs, 4T1 tumor-bearing mice were intravenously administered with the MnPcS@HPO NPs (600 μg mL−1, 200 μL). After injection, the T1-weighted MRI signal of MnPcS was scanned with a 3.0 T clinical MR scanner.
For in vitro photoacoustic (PA) imaging, a series of concentrations of the MnPcS@HPO NPs (0, 0.0625, 0.125, 0.25, 0.5 and 1 mg mL−1) was captured at 680 nm by a preclinical photoacoustic computerized tomography scanner (Verasonics, Inc. Kirkland, WA, USA). To evaluate the in vivo accumulation of the MnPcS@HPO NPs, tumor-bearing mice were used and the PA signal was recorded before and after intravenous injection of MnPcS@HPO (600 μg mL−1, 200 μL).
4.8
In vitro toxicity detection of MnPcS@HPO NPs
A CCK-8 assay was used to evaluate the toxicity of the MnPcS@HPO NPs. Briefly, 4T1 cells were seeded in 96-well plates (∼1 × 104 cells per well) and incubated overnight at 37 °C in 5% CO2. Then, the cells were treated with MnPcS@HP or MnPcS@HPO (MnPcS concentration of 75 μg mL−1) and incubated for 3 h. After this, the cells were irradiated by US irradiation for 2 min (1 W cm−2, 50% duty cycle, XK-2011R, Wuhan Xingkang, CHN). 21 h later, the cell viability was determined with a CCK-8 assay kit at 450 nm on a multifunctional Microplate Reader (Synergy H1 Hybrid, BioTek, GER).
4.9
In vitro uptake
4T1 cells were seeded into 8-well culture plates at a density of 2 × 104 per well and incubated for a night (37 °C, 5% CO2). Then, the MnPcS@HP NPs (75 μg mL−1 of MnPcS) were added and incubated with the cells for 1 h, 2 h and 3 h. The culture medium was removed, and Hoechst 33342 in fresh medium was used to stain the nucleus for 10 min before detection by confocal laser scanning microscopy (CLSM, TCS SP5II, Leica, Ernst-Leitz-Strasse, GER).
4.10
In vitro live/dead cell analysis
The 4T1 cell viability was assessed using a calcein/PI live/dead assay kit (Beyotime, CHN). Briefly, 4T1 cells were seeded in 96-well plates at 1 × 104 per well and incubated for a night (37 °C, 5% CO2). Then, the cells were incubated with MnPcS@HP or MnPcS@HPO for 3 h. After that, the cells were treated with or without US irradiation (1 W cm−2, 50% duty cycle, 2 min). After incubation for another 21 h, the cells were washed with PBS. Calcein-AM/PI reagent was used to stain the cells for 15 min to detect the live/dead cells on a fluorescent microscope (Olympus IX71, JPN).
4.11
In vitro reactive oxygen species (ROS) detection
The intracellular ROS production of MnPcS@HP or MnPcS@HPO was measured with the DCFH-DA probe. Briefly, 4T1 cells (2 × 104 per well) were seeded into glass-bottom 8-well plates until adherent and then incubated with MnPcS@HP or MnPcS@HPO (75 μg mL−1 of MnPcS) for 3 h. Then, 1 μL DCFH-DA (1 mg mL−1 DMSO) was added and co-incubated for 30 min. After this, the cells were treated with US irradiation (1 W cm−2, 50% duty cycle, 2 min) and cultured for another 1 h. The cells were washed with PBS twice and the cell nuclei were stained with Hoechst 33342 for 10 min at room temperature. The ROS generation was acquired by CLSM (Ernst-Leitz-Strasse, TCS SP5II, GER). Meanwhile, semiquantitative analysis of the ROS was performed by a multifunctional microplate reader.
4.12
In vivo sonodynamic therapy
Female Balb/c nude mice bearing 4T1 tumors were randomly divided into four groups (n = 5 for each group): PBS, PBS + US, MnPcS@HP + US and MnPcS@HPO + US group (600 μg mL−1 MnPcS, 200 μL). After the tumor volume was about 100 mm3, different treatments were conducted through intravenous injection. US exposure (1 W cm−2, 50% duty cycle) was carried out at 3 h. The tumor growth was evaluated every two days. The tumor size was recorded and calculated using the following formula: length × width2 × 0.5. During the therapeutic period of 20 days, the body weights of the mice were also measured.
4.13 Tissue immunohistochemistry
To assess the tumor tissue hypoxia, tumor tissues were stained with the hypoxia marker Hypoxyprobe-1 plus kit. At 3 h after the intravenous injection of MnPcS@HP or MnPcS@HPO, SOSG (10 μg per mice) was intraperitoneally injected into the tumor-bearing mice, and US irradiation (1 W cm−2, 50% duty cycle, 5 min) was conducted on the mice. After another 1 h, the mice were sacrificed and the tumor tissue was fixed in 4% paraformaldehyde, processed into paraffin and cut into 8 μm sections. The ROS signals of the tumors were examined by CLSM. The SDT effect evaluation was also similar to the above methods, except without injection of SOSG and the mice were sacrificed at 20 day. Then, the tumor tissue sections were stained with hematoxylin/eosin (H&E) or with TdT-mediated dUTP nick-end labeling (TUNEL) to estimate the apoptosis after SDT treatment. All of the histological analyses were conducted by CLSM.
4.14
In vivo biosafety evaluation of the MnPcS@HP NPs
Blood samples were collected by extracting blood from the eyeball after 20 days of treatment. The liver/kidney function was assessed by measuring the serum level of AST, ALT, UREA and CREA using activity assay kits according to the manufacturer's instructions. Major organs (heart, liver, spleen, lungs and kidneys) were collected and fixed for 24 h in formaldehyde at room temperature. The embedded paraffin blocks were cut into 8-μm slices for hematoxylin and eosin (H&E) staining. Images of different organs were captured using a fluorescence microscope (Olympus, IX71, JPN).
4.15 Statistical analysis
All statistical analyses were performed using GraphPad Prism software. The experimental data were reported as mean ± standard deviation (SD). The above data were statistically analyzed by one-way analysis of variance (ANOVA) tests between two groups or multiple groups. Values with *P < 0.05, **P < 0.01 and ***P < 0.001 were considered as significantly significant.
Author contributions
Aiqing Ma and Hong Pan: Conceptualization, methodology, writing-reviewing and editing; Hong Pan, Chengyu Lu and Aiqing Ma: supervision, administrative; Ting Yin and Jia Yin: writing–original draft preparation, Ting Yin, Jia Yin, Hui Ran, Yaguang Ren, Qingxia Shi and Yuzhi Qiu: technical, material support; acquisition of data; Lanlan Liu and Aiqing Ma: formal analysis (e.g., statistical analysis, biostatistics, computational analysis).
Conflicts of interest
The authors declare no conflict of interest.
Acknowledgements
This work was supported by the National Natural Science Foundation of China (81901864, 81971749, 82072064 and 21701033), the Natural Science Foundation of Guangdong Province (2021A1515010131 and 2019A1515011524), the Guangdong Province Universities and Colleges Pearl River Scholar Fund (4SG21006G), the Shenzhen Science and Technology Program (JCYJ20170818162259843 and JCYJ20210324115607020), the Guangdong Province Universities and Colleges Characteristic Innovation fund (2021KTSCX035 and 2021KTSCX036), the Guangdong Medical University PHD researcher Fund (2021), the Guangdong Medical University Discipline Construction Project (4SG21277P), and Special Funds of Scientific Technological Innovation of Undergraduates in Guangdong Province (Pdjh2021b0223 and pdjh2021b0227).
References
- M. S. Yavuz, Y. Y. Cheng, J. Y. Chen, C. M. Cobley, Q. Zhang, M. Rycenga, J. W. Xie, C. Kim, K. H. Song, A. G. Schwartz, L. H. V. Wang and Y. N. Xia, Gold nanocages covered by smart polymers for controlled release with near-infrared light, Nat. Mater., 2009, 8, 935–939 CrossRef CAS.
- W. P. Fan, B. Yung, P. Huang and X. Y. Chen, Nanotechnology for Multimodal Synergistic Cancer Therapy, Chem. Rev., 2017, 117, 13566–13638 CrossRef CAS PubMed.
- G. Liu, X. Zhao, Y. Zhang, J. Xu, J. Xu, Y. Li, H. Min, J. Shi, Y. Zhao and J. Wei, Engineering Biomimetic Platesomes for pH–Responsive Drug Delivery and Enhanced Antitumor Activity, Adv. Mater., 2019, 31, 1900795.1–1900795.12 Search PubMed.
- R. Liang, L. Liu, H. He, Z. Chen, Z. Han, Z. Luo, Z. Wu, M. Zheng, Y. Ma and L. Cai, Oxygen-Boosted Immunogenic Photodynamic Therapy with Gold Nanocages@Manganese Dioxide to Inhibit Tumor Growth and Metastases, Biomaterials, 2018, 177, 149–160 CrossRef CAS PubMed.
- W. Fan, B. Yung, P. Huang and X. Chen, Nanotechnology for Multimodal Synergistic Cancer Therapy, Chem. Rev., 2017, 117, 13566–13658 CrossRef CAS.
- P. Huang, X. Qian, Y. Chen, L. Yu, H. Lin, L. Wang, Y. Zhu and J. Shi, Metalloporphyrin-Encapsulated Biodegradable Nanosystems for Highly Efficient Magnetic Resonance Imaging-Guided Sonodynamic Cancer Therapy, J. Am. Chem. Soc., 2017, 139, 1275–1284 CrossRef CAS PubMed.
- X. Qian, Y. Zheng and C. Yu, Micro/Nanoparticle-Augmented Sonodynamic Therapy (SDT): Breaking the Depth Shallow of Photoactivation, Adv. Mater., 2016, 28, 8097–8129 CrossRef CAS.
- M. Trendowski, The promise of sonodynamic therapy, Cancer Metastasis Rev., 2014, 33, 143–160 CrossRef CAS PubMed.
- S. Liang, X. Deng, P. Ma, Z. Cheng and J. Lin, Recent Advances in Nanomaterial-Assisted Combinational Sonodynamic Cancer Therapy, Adv. Mater., 2020, e2003214 CrossRef.
- S. Son, J. H. Kim, X. Wang, C. Zhang, S. A. Yoon, J. Shin, A. Sharma, M. H. Lee, L. Cheng, J. Wu and J. S. Kim, Multifunctional Sonosensitizers in Sonodynamic Cancer Therapy, Chem. Soc. Rev., 2020, 49, 3244–3261 RSC.
- M. Trendowski, Using the Promise of Sonodynamic Therapy in the Clinical Setting against Disseminated Cancers, Chemother. Res. Pract., 2015, 2015, 316015–316031 Search PubMed.
- Y. Yin, X. Jiang, L. Sun, H. Li, C. Su, Y. Zhang, G. Xu, X. Li, C. Zhao, Y. Chen, H. Xu and K. Zhang, Continuous inertial cavitation evokes massive ROS for reinforcing sonodynamic therapy and immunogenic cell death against breast carcinoma, Nano Today, 2021, 36, 101009 CrossRef CAS.
- H. Chen, L. Liu, A. Ma, T. Yin, Z. Chen, R. Liang, Y. Qiu, M. Zheng and L. Cai, Noninvasively immunogenic sonodynamic therapy with manganese protoporphyrin liposomes against triple-negative breast cancer, Biomaterials, 2021, 269, 120639 CrossRef CAS.
- H. Mei, X. Zhang, S. Cai, X. Zhang, Y. Zhang, Z. Guo, W. Shi, R. Chu, K. Zhang, J. Cao and B. He, Fluorocarbon-Driven Photosensitizer Assembly Decodes Energy Conversion Pathway for Suppressing Breast Tumor, Nano Today, 2021, 41, 101305–101315 CrossRef.
- X. Chen, Nanotechnology for Multimodal Synergistic Cancer Therapy, Chem. Rev., 2017, 117, 13566–13638 CrossRef.
- J. Ge, M. Lan, B. Zhou, W. Liu, L. Guo, H. Wang, Q. Jia, G. Niu, X. Huang, H. Zhou, X. Meng, P. Wang, C. S. Lee, W. Zhang and X. Han, A graphene quantum dot photodynamic therapy agent with high singlet oxygen generation, Nat. Commun., 2014, 5, 4596–4604 CrossRef CAS PubMed.
- Q. Zhang, C. Bao, X. Cai, L. Jin, L. Sun, Y. Lang and L. Li, Sonodynamic Therapy-Assisted Immunotherapy: A Novel Modality for Cancer Treatment, Cancer Sci., 2018, 109, 1330–1345 CrossRef CAS PubMed.
- J. Chen, H. Luo, Y. Liu, W. Zhang, H. Li, T. Luo, K. Zhang, Y. Zhao and J. Liu, Oxygen-Self-Produced Nanoplatform for Relieving Hypoxia and Breaking Resistance to Sonodynamic Treatment of Pancreatic Cancer, ACS Nano, 2017, 11, 12849–12862 CrossRef CAS PubMed.
- X. Guan, H. H. Yin, X. H. Xu, G. Xu, Y. Zhang, B. G. Zhou, W. W. Yue, C. Liu, L. P. Sun, H. X. Xu and K. Zhang, Tumor Metabolism–Engineered Composite Nanoplatforms Potentiate Sonodynamic Therapy via Reshaping Tumor Microenvironment and Facilitating Electron–Hole Pairs’ Separation, Adv. Funct. Mater., 2020, 30, 2000326–2000335 CrossRef CAS.
- T. Luo, D. Wang, L. Liu, Y. Zhang, C. Han, Y. Xie, Y. Liu, J. Liang, G. Qiu, H. Li, D. Su, J. Liu and K. Zhang, Switching Reactive Oxygen Species into Reactive Nitrogen Species by Photocleaved O2-Released Nanoplatforms Favors Hypoxic Tumor Repression, Adv. Sci., 2021, 8, 2101065–2101073 CrossRef.
- N. Zhang, Y. Tan, L. Yan, C. Zhang, M. Xu, H. Guo, B. Zhuang, L. Zhou and X. Xie, Modulation of Tumor Hypoxia by pH-Responsive Liposomes to Inhibit Mitochondrial Respiration for Enhancing Sonodynamic Therapy, Int. J. Nanomed., 2020, 15, 5687–5700 CrossRef CAS.
- C. H. Hsieh, C. H. Lee, J. A. Liang, C. Y. Yu, W. C. Shyu, H. Hatzikirou, D. Basanta, M. Simon, K. Schaller and A. Deutsch, Exploiting tumour hypoxia in cancer treatment, Nat. Rev. Cancer, 2004, 4, 437–447 CrossRef.
- Z. Lu, J. Ma, B. Liu, C. Dai, T. Xie, X. Ma, M. Li, J. Dong, Q. Lan and Q. Huang, Hyperbaric oxygen therapy sensitizes nimustine treatment for glioma in mice, Cancer Med., 2016, 5, 3147–3155 CrossRef CAS.
- C. Yu-Liang, Z. Ya-Nan, W. Zhong-Zhuang, X. Wei-Gang, L. Run-Ping and Z. Jun-Dong, Effects of adenosine metabolism in astrocytes on central nervous system oxygen toxicity, Brain Res., 2016, 1635, 180–189 CrossRef.
- P. Zhang, L. Zhang, J. Wang, L. Zhu, Z. Li, H. Chen and Y. Gao, An intelligent hypoxia-relieving chitosan-based nanoplatform for enhanced targeted chemo-sonodynamic combination therapy on lung cancer, Carbohydr. Polym., 2021, 274, 118655–118667 CrossRef CAS PubMed.
- S. Xuejiao, F. Liangzhu, L. Chao, Y. Kai and L. Zhuang, Ultrasound Triggered Tumor Oxygenation with Oxygen-Shuttle Nanoperfluorocarbon to Overcome Hypoxia-Associated Resistance in Cancer Therapies, Nano Lett., 2016, 16, 6145–6153 CrossRef.
- F. Wenpei, B. Wenbo, S. Bo, H. Qianjun, C. Zhaowen, L. Yanyan, Z. Xiangpeng, Z. Kuaile and S. Jianlin, Intelligent MnO2 Nanosheets Anchored with Upconversion Nanoprobes for Concurrent pH–/H2O2−Responsive UCL Imaging and Oxygen–Elevated Synergetic Therapy, Adv. Mater., 2015, 27, 4155–4161 CrossRef.
- B. Yuheng, C. Jifan, Q. Huiqiang, Z. Cong, H. Pintong, M. Zhengwei and T. Weijun, Erythrocyte Membrane-Camouflaged PCN-224 Nanocarriers Integrated with Platinum Nanoparticles and Glucose Oxidase for Enhanced Tumor Sonodynamic Therapy and Synergistic Starvation Therapy, ACS Appl. Mater. Interfaces, 2021, 13, 24532–24542 CrossRef.
- P. Zhu, Y. Chen and J. Shi, Nanoenzyme-Augmented Cancer Sonodynamic Therapy by Catalytic Tumor Oxygenation, ACS Nano, 2018, 12, 3780–3795 CrossRef CAS PubMed.
- P. Xueting, W. Weiwei, H. Zhijun, L. Shuang, G. Juan, Z. Fengrong, Y. Hongjun, L. Xin, L. Fengyong and L. Huiyu, MOF-Derived Double-Layer Hollow Nanoparticles with Oxygen Generation Ability for Multimodal Imaging-Guided Sonodynamic Therapy, Angew. Chem., Int. Ed., 2020, 59, 13557–13561 CrossRef.
- G. Li, J. Li, B. Jin, W. Ji, Y. Huang, X. Luo and Z. Liang, Efficient Metal–Organic Framework-Derived Cu–Zr Oxygen Carriers with an Enhanced Reduction Reaction Rate for Chemical Looping Air Separation, ACS Sustainable Chem. Eng., 2020, 8, 14795–14806 CrossRef CAS.
- Z. Chen, L. Liu, R. Liang, Z. Luo, H. He, Z. Wu, H. Tian, M. Zheng, Y. Ma and L. Cai, Bioinspired Hybrid Protein Oxygen Nanocarrier Amplified Photodynamic Therapy for Eliciting Anti-tumor Immunity and Abscopal Effect, ACS Nano, 2018, 12, 8633–8645 CrossRef CAS PubMed.
- W. Tang, Z. Zhen, M. Wang, H. Wang, Y. J. Chuang, W. Zhang, G. D. Wang, T. Todd, T. Cowger and H. Chen, Red Blood Cell–Facilitated Photodynamic Therapy for Cancer Treatment, Adv. Funct. Mater., 2016, 26, 1757–1768 CrossRef CAS.
- I. N. Fleming, R. Manavaki, P. J. Blower, DPhil, CChem, FRSC, C. West, K. J. Williams, A. L. Harris and FMedSci, Imaging Tumour Hypoxia with Positron Emission Tomography Running title: Imaging Tumour Hypoxia with PET, Br. J. Cancer, 2015, 112, 238–250 CrossRef CAS PubMed.
- T. Li, X. Jing and Y. Huang, Polymer/Hemoglobin Assemblies: Biodegradable Oxygen Carriers for Artificial Red Blood Cells, Macromol. Biosci., 2011, 11, 865–875 CrossRef CAS PubMed.
- A. Ma, H. Chen, Y. Cui, Z. Luo, R. Liang, Z. Wu, Z. Chen, T. Yin, J. Ni, M. Zheng and L. Cai, Metalloporphyrin Complex-Based Nanosonosensitizers for Deep-Tissue Tumor Theranostics by Noninvasive Sonodynamic Therapy, Small, 2019, 15, 1804028–1804038 CrossRef PubMed.
- Z. Luo, H. Tian, L. Liu, Z. Chen, R. Liang, Z. Chen, Z. Wu, A. Ma, M. Zheng and L. Cai, Tumor-targeted hybrid protein oxygen carrier to simultaneously enhance hypoxia-dampened chemotherapy and photodynamic therapy at a single dose, Theranostics, 2018, 8, 3584–3596 CrossRef CAS.
- Y. Daijima and T. Komatsu, Haemoglobin Wrapped Covalently by Human Serum Albumin Mutants Containing Mn(III) Protoporphyrin IX: An O2 Complex Stable in H2O2 Solution, Chem. Commun., 2014, 50, 14716–14719 RSC.
- T. Komatsu, N. Ohmichi, A. Nakagawa, P. A. Zunszain, S. Curry and E. Tsuchida, O2 and CO Binding Properties of Artificial Hemoproteins Formed by Complexing Iron Protoporphyrin IX with Human Serum Albumin Mutants, J. Am. Chem. Soc., 2005, 127, 15933–15942 CrossRef CAS PubMed.
- H. Xiongyi and T. G. John, Oxygen Activation and Radical Transformations in Heme Proteins and Metalloporphyrins, Chem. Rev., 2017, 118, 2491–2553 Search PubMed.
- J. P. Perdew, K. Burke and M. Ernzerhof, Generalized Gradient Approximation Made Simple, Phys. Rev. Lett., 1996, 77, 3865–3868 CrossRef CAS PubMed.
- C. Liang, J. Xie, S. Luo, C. Huang, Q. Zhang, H. Huang and P. Zhang, A highly potent ruthenium(II)-sonosensitizer and sonocatalyst for in vivo sonotherapy, Nat. Commun., 2021, 12, 5001 CrossRef CAS PubMed.
Footnotes |
† Electronic supplementary information (ESI) available. See DOI: 10.1039/d1bm01710a |
‡ These authors contributed equally to this work. |
|
This journal is © The Royal Society of Chemistry 2022 |