DOI:
10.1039/D1AN01210J
(Paper)
Analyst, 2022,
147, 147-154
A rapid procedure for bacterial identification and antimicrobial susceptibility testing directly from positive blood cultures†
Received
6th July 2021
, Accepted 13th September 2021
First published on 15th November 2021
Abstract
There is an urgent need to develop a rapid procedure that can rapidly identify and obtain antimicrobial susceptibility testing (AST) results directly from positive blood cultures. Here, we report a semi-automatic bacterial diagnosis procedure, which includes (1) a bacterial concentration process to isolate bacteria from a positive blood culture bottle (PBCB), (2) an identification process using matrix-assisted laser desorption ionization time-of-flight mass spectrometry (MALDI-TOF MS), and (3) a rapid AST process based on stimulated Raman scattering imaging of deuterium oxide (D2O) incorporation in bacteria. A total of 105 samples were tested for bacterial identification, and a bacterial identification accuracy of 92.3% was achieved. AST takes about 2.5 h after identification. This semi-automatic procedure only takes 3.5 h, which is demonstrated to be the fastest process to obtain identification and AST results starting from PBCBs.
1. Introduction
Antimicrobial-resistant infections cause about 1 million deaths each year globally.1 In particular, bloodstream infections have high mortality rates, sequelae risks, and serious complications.1,2 One of the major reasons for this crisis is over-prescribing of antibiotics, which facilitates antimicrobial-resistance development. Bacterial identification and antimicrobial susceptibility testing (AST) are routinely performed in hospitals to prescribe appropriate antibiotics to patients. The whole process requires four steps: blood culture, subculture, identification, and AST, resulting in a total turnaround time of at least two to three days.2–4 Thus, the correct treatment can be delayed significantly.
To facilitate microbial diagnosis, researchers have developed various methods to target different aspects of this process.5,6 As a rapid bacterial identification technology, matrix-assisted laser desorption ionization time-of-flight mass spectrometry (MALDI-TOF MS) has made a revolutionary breakthrough in the clinical microbiology lab.7–9 This method had also been incorporated for direct and rapid bacterial identification from positive blood cultures,10–15 with an overall performance ranging from 60% to 99% concordance to a species level.16–19 As a rapid bacterial AST technology, Raman spectroscopy has been used to determine antimicrobial susceptibility by detecting metabolic incorporation of deuterium oxide (D2O), or heavy water, in bacteria.20–23 In this method, bacteria are incubated with a D2O-containing medium. The metabolic activity of bacteria can be gauged by quantifying the conversion of D2O to C–D bonds of biomolecules. However, Raman scattering is a weak process due to its small vibrational cross-section, hindering its application for rapid and high-throughput measurement. These limitations are overcome by the coherent Raman scattering technology, which enhances the Raman signal significantly and receives wide applications.24–26 Coherent Raman scattering microscopy, a rising molecular imaging technique in which the excitation energy is focused on the molecular vibration band,24,25 includes coherent anti-Stokes Raman scattering (CARS)24–26 and stimulated Raman scattering (SRS) microscopy.24–28 Specifically, SRS microscopy, which does not have a nonresonant background like CARS, has been used for rapid and accurate AST by quantifying the C–D bond within a single bacterium.27–31 It is demonstrated that the coherent Raman scattering microscopy-based AST process can generate single-cell metabolism inactivation concentration (SC-MIC) results within 2 h with ∼94% categorical agreement for bacterial isolates.27,31
In this study, we integrate the MALDI-TOF MS and SRS imaging as a platform for rapid bacterial identification and AST directly from positive blood culture bottles (PBCBs). Our procedure consists of 3 steps. In the first step, we applied a semi-automatic centrifugation chip to isolate bacteria from the PBCBs. This method eliminates the need for subculture and therefore saves 12–24 hours for the procedure. In the second step, half of the isolated bacteria were sent to MALDI-TOF MS for identification. Once the identification results were known, another half of the isolated bacteria sample was cultured with D2O medium and different serially diluted antibiotics for about 1.5 h. The bacteria were then imaged by SRS to measure their metabolic activity for SC-MIC results. We have tested a set of 105 samples, with an identification rate of 92.3%. The total turnaround time of the whole procedure is averaged to be about 3.5 h. This procedure is demonstrated to be the fastest process to obtain identification and AST results starting from PBCBs.
2. Materials and methods
2.1 Fabrication of the microfluidic chip and the automatic machinery
The microfluidic chip was prepared using polymethyl methacrylate (PMMA). Each disk, measuring 60 mm (radius) × 8 mm (thickness), was prepared by laser cutting (HiComp Microtech, China). The disks were sealed using single-sided pressure-sensitive adhesives (PSA, Adhesive Research, Inc., China). Each unit consists of a sample loading chamber, a mixing and resuspension chamber, a sample collection chamber, and a waste chamber. The depth of each part, sample loading chamber, mixing and resuspension chamber, sample collection chamber and waste chamber, is 4 mm. The diameters of the sample collection chamber are 3 mm. The chip was placed on top of a centrifugal servo motor, which can be programmed to stop at a desired angle and position.
2.2 SRS setup
The schematic setup of the SRS imaging system is shown in Fig. S1.† In brief, a dual output femtosecond pulsed laser (InSight DeepSee X3, Spectra-Physics) with a repetition rate of 80 MHz was used for the SRS microscopy. A 120 fs laser with a tunable from 680–1100 nm was used as the pump beam. The other 220 fs laser, centered at 1045 nm and which served as the Stokes beam, was modulated using an acoustic-optical modulator (AOM, 1205-C, Isomet) at ∼1.85 MHz. The pump and Stokes beams were combined and guided into a lab-built laser scanning microscope. A 60× oil objective (NA = 1.3, UPlanApo, Olympus) focused the two lasers on samples, and an oil condenser (NA = 1.4, U-AAC, Olympus) was used to gather signals from the samples. Two filters (HQ 825/150 nm, Chroma) were used to filter out the Stokes beam. The pump beam was detected using a photodiode (S3994-01, Hamamatsu), and the stimulated Raman loss signal was extracted using a lock-in amplifier (HF2L1, Zurich Instruments).
For SRS imaging at the C–D vibrational frequency, the pump wavelength was tuned to 852 nm, and the power at the sample was about 10 mW; the Stokes power at the sample was about 60 mW. Each image contains 200 × 200 pixels, and the pixel dwell time is 50 μs. All the laser powers were measured after the dichroic mirror for all experiments.
2.3 Imaging process and data analysis
The SRS images were analyzed using ImageJ software (ImageJ, NIH). SRS images opened in ImageJ were first converted into 8-bit type images with inverted color. The images were then filtered with Gaussian blur, followed by threshold adjustment. The threshold was adjusted so that the selected bacterial sizes match those in the original SRS images. Next, particle analysis was applied to label and determine the area of bacteria. Small particles were eliminated by adjusting the size threshold. By applying the same label and area of bacteria to the original SRS images, the average intensity of each data point was determined after subtraction of the background.
2.4 Procedures of blood cultures
Bacterial isolates cultured overnight were diluted and inoculated into a blood bottle (BacT/ALERT FA Plus, bioMérieux, France) with an initial concentration of 102 colony-forming units (CFU) per mL. After that, 8–10 mL horse blood was added into the target bottles to simulate blood cultures. The blood bottles were incubated in an automatic incubator until the system was alarmed, indicating a positive blood culture. The horse blood was purchased from Sbjbio Life Sciences, China.
2.5 Bacterial sample preparation
To prepare 70% D2O containing Luria-Bertani broth (LB) (Sigma Aldrich) media, D2O was mixed with purified water, and then LB powder was added to the solution. To prepare bacterial samples for SRS imaging, bacterial cells were collected from the microfluidic chip and diluted to ∼106 CFU mL−1 in the D2O-containing medium. After centrifuged and washed twice with purified water, the sample was fixed by 10% formalin solution (Thermo Fisher Scientific) and deposited on a poly-L-lysine coated coverglass.
2.6 Conventional identification with MALDI-TOF MS and AST using a VITEK-2 Compact system
Subcultures of clinical strains were inoculated on agar plates and cultured at 35 °C until visible colonies appeared. Then, the pick-up colonies were adjusted to 0.5 McFarland. For the conventional identification procedure, bacterial colonies from the plates were smeared onto a steel target plate. When bacteria were dried, 1 μL of α-cyano-4-hydroxycinnamic acid (HCCA) and MALDI matrix solution (a saturated solution of α-cyano-4-hydroxycinnamic acid with 50% acetonitrile and 2.5% trifluoroacetic acid) was added for the subsequent MALDI-TOF MS (VITEM MS, bioMérieux, France) identification. For AST with the VITEK-2 Compact system (based on a microdilution broth method), the bacterial colony was diluted with 0.45% saline and adjusted to a density of 0.5 McFarland. Then 145 μL or 280 μL of the bacterial solution was diluted to 3 mL and inoculated into VITEK cards and loaded into a VITEK-2 Compact automated reader-incubator. VITEK cards AST-GN09 and AST-GP67 were used.
3. Results and discussion
3.1 Workflow of rapid identification and AST procedure
The workflow of our rapid bacterial identification and AST is shown in Fig. 1. Specimens from PBCB, which contain bacterial cells with an average concentration of 108 CFU mL−1,4 were first filtered with 5 μm filters to remove large impurities. A red blood cell lysis buffer that contains ammonium chloride was then added to the specimens to lyse the blood cells. After about 15 min lysis, the specimens were transferred to a semi-automatic centrifugation device to wash and concentrate bacterial cells. The semi-automatic centrifugation device contains a microfluidic chip and a sample adding device. It can centrifuge and resuspend six specimens simultaneously. After this bacterial concentration process, parts of the specimens in the centrifugation chip were used for bacterial identification with MALDI-TOF MS. The total time of bacterial identification is about 0.5 h. Once bacterial species were identified, parts of the specimens were treated with selected antibiotics of serial dilutions and D2O, and SRS was performed for AST. The process of AST took about 2.5 h. The whole process of bacterial identification and AST from PBCBs to report was about 3.5 h. For comparison, we performed traditional bacterial identification and AST with the VITEK-2 Compact system, and the whole process took on average 48 h starting from PBCBs.
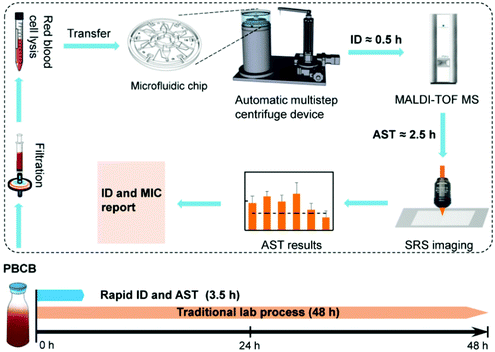 |
| Fig. 1 The workflow of rapid bacterial identification and AST from PBCBs. | |
3.2 Semi-automatic centrifugation device for separation of bacteria from PBCBs
Our previous study demonstrated rapid AST of bacteria separated from blood samples with SRS imaging.27 Bacterial identification directly from positive blood cultures was also demonstrated in many studies.32,33 However, the bacterial concentration process requires many manual steps. To reduce the manual steps in separating bacteria from blood, we developed a semi-automatic centrifugation device that can centrifuge and add a resuspension medium automatically (Fig. 2A and B and Fig. S2†). The device has two parts: a centrifugal microfluidic chip that centrifuges and resuspends samples and a sample loading device that adds resuspension media to the chip. The microfluidic chip has six units and, therefore, can process six specimens simultaneously. Each unit consists of 4 chambers, a sample loading chamber, a mixing and resuspension chamber, a sample collection chamber, and a waste chamber (Fig. 2A). The sample loading device has an electrical machinery, an actuating motor, a controller, and a computer (Fig. 2B). The procedures and corresponding illustrations and images are shown in Fig. 2C and D, respectively. The whole procedure from loading the blood–bacteria mixture to sampling for identification and AST was executed automatically with eight steps. The blood–bacteria mixture (200 μL) was injected into the sample loading chamber using the sample loading device (1). The samples were then centrifuged at a speed of 3000 revolutions-per-minute (rpm) for 300 s (2). After centrifugation, the sample pellets were in the sample collection chamber while the supernatant was collected in the waste chamber. Then, a resuspension solution was added to the sample loading chamber (3). The sample pellets were mixed with the resuspension solution in the mixing and resuspension chamber by repeated clockwise and counter-clockwise centrifugation at 330 rpm, with 10 s for each centrifugation and ten repetitions (4). Then, the samples were centrifuged again to remove the resuspension solution (5).
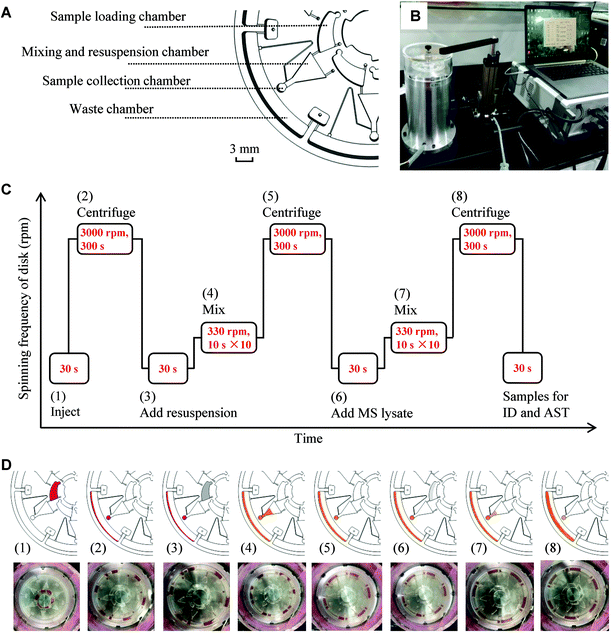 |
| Fig. 2 Experimental setup of the semi-automatic centrifugation device and procedures of sample handling. (A) Illustration of the centrifugal microfluidic chip. (B) Photo of the semi-automatic centrifugation device. (C) The procedures of bacterial samples processing from PBCBs with the centrifugation device. (D) The corresponding illustrations and photos of each step. | |
To prepare specimens for MALDI-TOF MS analysis, we further lysed the samples in three channels by adding lysis reagents containing formic acid and acetonitrile to the sample loading chamber (6). In steps (7) and (8), the mixing and centrifugation processes were repeated to remove the supernatant solution. The samples in the sample collection chamber were collected for MALDI-TOF MS analysis, and the rest of the samples were collected for AST. The total time for the sample processing is about 20 min from adding samples to making the samples ready for identification and AST.
3.3 Direct identification of bacteria from PBCBs with MALDI-TOF MS
To explore whether the concentrated bacterial samples from the centrifugation device can be used for direct identification with MALDI-TOF MS, we collected bacterial samples from the centrifugation chip and spotted them onto a steel target plate with duplicates. After being air dried, HCCA matrix solution was added to the samples. Then, the plate was loaded to MALDI-TOF MS for subsequent identification (Fig. 3A).
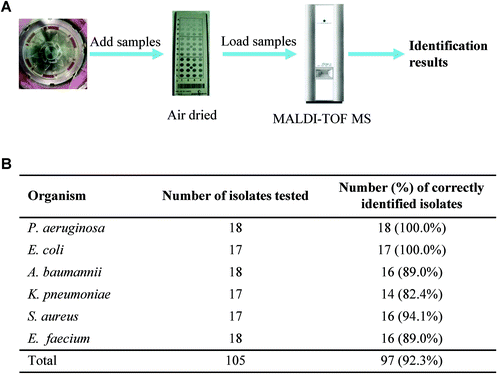 |
| Fig. 3 Procedures and identification rates of bacterial identification from PBCBs with MALDI-TOF MS. (A) Procedures of bacterial identification from PBCBs with MALDI-TOF MS. (B) Results of bacterial identification with MALDI-TOF MS. | |
We tested six bacterial species commonly encountered in the clinic: P. aeruginosa, E. coli, A. baumannii, K. pneumoniae, S. aureus, and E. faecium, each with 17 or 18 bacterial strains. For those bacterial species whose identification accuracy is greater than 90.0%, the system judges that the identification is correct. With a total of 105 bacterial samples tested, 97 of them were correctly identified. The correct identification rates are 100.0% for P. aeruginosa and E. coli, 94.1% for S. aureus, 89.0% for A. baumannii and E. faecium, and 82.4% for K. pneumoniae. The remaining 8 samples were classified as failures because the identification accuracy was less than 90.0%.
On average, the correct identification rates are 91.4% for Gram-positive bacteria and 92.9% for Gram-negative bacteria at the species level. The identification rate is higher in Gram-negative bacteria than that in Gram-positive bacteria because the cell walls of Gram-positive bacteria, with tetrapeptide side chains and pentapeptide cross-linking bridges, are relatively thick, while the cell walls of Gram-negative bacteria are thinner. When the bacteria are at a low concentration, the cell wall breaking efficiency can affect ribosomal protein exposure, which in turn affects the efficiency of mass spectrometry identification. Collectively, these results demonstrate that this semi-automatic bacterial concentrate system achieves a high identification rate and can effectively replace a subculture process when pre-cultured to positive blood cultures.
To test the detection limit of bacterial identification from PBCBs with MALDI-TOF MS, we made bacterial broth from the bottles by 10 times dilution and set the initial concentration to 108 CFU mL−1. The results show that when the concentration of the pathogen is 105 CFU mL−1, MALDI-TOF MS can identify all kinds of target pathogens.
3.4 Rapid AST of bacteria from PBCB with SRS imaging
The procedures for rapid and direct AST from PBCB are shown in Fig. 4A. Bacterial samples collected from the microfluidic chip were diluted and added to a 96-well plate, in which a culture medium containing antibiotics at different concentrations was pre-added. After 1 h culture, the D2O culture medium was added to each sample at the plate to a final concentration of D2O of 70%. After another 1 h culture, the samples were centrifuged and deposited on glass slides for SRS imaging. AST results were determined based on the C–D intensity of bacteria that reflects the bacterial metabolic activities (Fig. 4A).
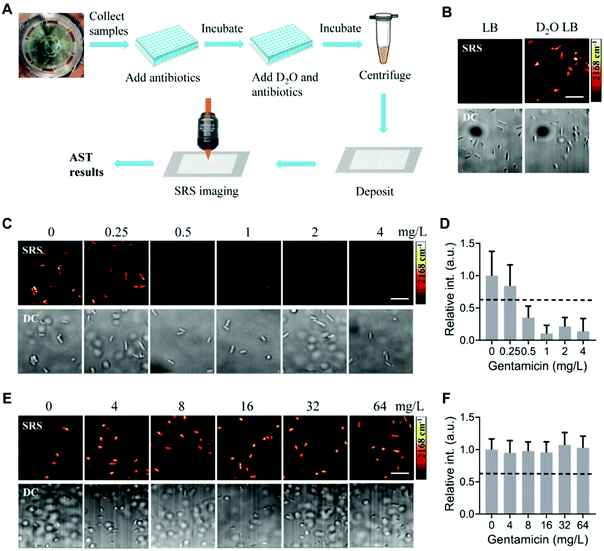 |
| Fig. 4 Rapid AST directly from PBCBs using SRS metabolic imaging. (A) The workflow of rapid AST of bacterial samples from the centrifugation device using SRS imaging. (B) SRS and corresponding transmission images of E. coli after culture in a normal and a D2O-containing LB medium for 1 h. DC, transmission images. (C) SRS at C–D and corresponding transmission images of E. coli ATCC 25922 after separation from PBCBs and culture in the D2O medium with gentamicin. (D) Statistical analysis of the normalized average C–D intensity of bacteria at different gentamicin concentrations in (C). Number of cells analyzed >10 at each concentration. (E) SRS at C–D and corresponding transmission images of E. coli 360 after separation from PBCBs and culture in the D2O medium with gentamicin. (F) Statistical analysis of the normalized average C–D intensity of bacteria at different gentamicin concentrations in (E). Number of cells analyzed >10 at each concentration. | |
To explore whether this protocol works for the automatic separated bacterial samples, we first tested the detection of D2O metabolism with SRS imaging. After culturing the separated samples in normal Luria broth (LB) medium and 70% D2O-containing LB medium for 1 h, we imaged them at the C–D vibrational region by tuning the Raman shift to 2168 cm−1. Bacterial samples cultured in the normal LB medium show no or negligible C–D signals. In contrast, bacterial samples cultured in the D2O-containing medium show clear C–D signals (Fig. 4B). The corresponding transmission images show the presence of bacterial cells. We did not observe blood cells in the transmission images, although we observed debris, probably from lysed blood cells. Nevertheless, with SRS imaging at the C–D vibrational region, the debris did not show strong C–D signals. Therefore, the debris did not interfere with the detection of bacteria with SRS imaging. These results collectively demonstrate that the bacterial samples processed with the centrifugation device can be used for SRS imaging of the D2O metabolism of bacteria.
Next, we determined SC-MIC with the automatic separated bacterial samples. Our previous study demonstrated the determination of SC-MIC with SRS imaging of D2O metabolism for bacteria in blood after manual centrifugation and separation. Here, we used the automatically processed sample from a PBCB and followed the same protocol as our previous study.27 In brief, samples were first diluted and cultured in a normal medium with antibiotics at different concentrations for 1 h. Then, a D2O medium containing antibiotics at the same concentration was added to each sample. After 1 h culture in the D2O medium, samples were centrifuged, deposited on glass slides, and imaged with SRS at the C–D vibrational region. Using the same threshold determined in our previous study that C–D signal intensity drops to 0.62 of that in the control group,27 the SC-MIC of E. coli ATCC 25922 against gentamicin was determined to be 0.5 mg L−1 (Fig. 4C and D). This value is within the Clinical Laboratory Standard Institute (CLSI) MIC quality control range (0.25–1 mg L−1).34 For another bacterial strain, E. coli 360, its SC-MIC against gentamicin was determined to be >64 mg L−1 (Fig. 4E and F), which also agreed with the MIC results (≥16 mg L−1) determined with the VITEK-2 Compact system. These results collectively showed that the SRS metabolic imaging method could determine SC-MIC for bacterial samples processed with the automatic centrifugation device.
Conclusions
We integrated MALDI-TOF and SRS imaging as a platform for rapid bacterial identification and AST directly from PBCBs. Our procedure consists of 3 steps. In the first step, we applied a semi-automatic centrifugation device to isolate and concentrate bacterial samples from PBCB. This method eliminates the need for a subculture process. In the second step, half of the isolated bacterial samples were loaded to a MALDI-TOF MS system for bacterial identification. With the identification results, another half of the bacterial samples were cultured with D2O medium and antibiotics at different concentrations for ∼2.5 h. SRS was then used to image the bacteria to measure their metabolic activity for SC-MIC determination. We have tested 105 samples for identification. The correct identification rate is 92.3%. The total turnaround time of the whole procedure is averaged to be ∼3.5 h. This procedure is demonstrated to be the fastest process to obtain bacterial identification and AST results directly from positive blood cultures.
Author contributions
W. H., G. Z., P. W. and X. K. conceptualized the work. L. L. and R. L. developed the centrifugation chip. B. S. acquired the data. C. C., W. Z. and S. H. analyzed the data. B. S., W. H., P. W., and G. Z. wrote the manuscript. Y.F., S.Y. and C.Z. reviewed the manuscript.
Conflicts of interest
There are no conflicts to declare.
Acknowledgements
This research was supported by the Natural Science Foundation of Beijing (7204274) and the National Natural Science Foundation of China (81901790).
References
-
J. O'Neill, Tackling drug-resistant infections globally: final report and recommendations. The review on antimicrobial resistance, 2016 Search PubMed.
- O. Opota, A. Croxatto, G. Prod'hom and G. Greub, Blood culture-based diagnosis of bacteraemia: state of the art, Clin. Microbiol. Infect., 2015, 21, 313–322 CrossRef CAS.
- J. H. Jorgensen and M. J. Ferraro, Antimicrobial susceptibility testing: a review of general principles and contemporary practices, Clin. Infect. Dis, 2009, 49, 1749–1755 CrossRef CAS PubMed.
- J. Choi,
et al., Direct, rapid antimicrobial susceptibility test from positive blood cultures based on microscopic imaging analysis, Sci. Rep., 2017, 7, 1148 CrossRef PubMed.
- D. C. Spencer, T. F. Paton, K. T. Mulroney, T. J. Inglis, J. M. Sutton and H. Morgan, A fast impedance-based antimicrobial susceptibility test, Nat. Commun., 2020, 11, 1–11 Search PubMed.
- A. van Belkum, C. A. D. Burnham, J. W. Rossen, F. Mallard, O. Rochas and W. M. Dunne, Innovative and rapid antimicrobial susceptibility testing systems, Nat. Rev. Microbiol., 2020, 18, 299–311 CrossRef CAS PubMed.
- Y. Tian, B. Zheng, B. Wang, Y. Lin and M. Li, Rapid identification and multiple susceptibility testing of pathogens from positive-culture sterile body fluids by a combined MALDI-TOF mass spectrometry and vitek susceptibility system, Front. Microbiol., 2016, 7, 523 Search PubMed.
- S. Angeletti, Matrix assisted laser desorption time of flight mass spectrometry (MALDI-TOF MS) in clinical microbiology, J. Microbiol. Methods, 2017, 138, 20–29 CrossRef CAS PubMed.
- G. Dubourg, B. Lamy and R. Ruimy, Rapid phenotypic methods to improve the diagnosis of bacterial bloodstream infections: meeting the challenge to reduce the time to result, Clin. Microbiol. Infect., 2018, 24, 935–943 CrossRef CAS PubMed.
- A. Bizzini and G. Greub, Matrix-assisted laser desorption ionization time-of-flight mass spectrometry, a revolution in clinical microbial identification, Clin. Microbiol. Infect., 2010, 16, 1614–1619 CrossRef CAS PubMed.
- P. Seng,
et al., Ongoing revolution in bacteriology: routine identification of bacteria by matrix-assisted laser desorption ionization time-of-flight mass spectrometry, Clin. Infect. Dis, 2009, 49, 543–551 CrossRef CAS PubMed.
- N. G. Morgenthaler and M. Kostrzewa, Rapid identification of pathogens in positive blood culture of patients with sepsis: review and meta-analysis of the performance of the sepsityper kit, Internet J. Microbiol., 2015, 2015, 827416 Search PubMed.
- M. Drancourt, Detection of microorganisms in blood specimens using matrix-assisted laser desorption ionization time-of-flight mass spectrometry: a review, Clin. Microbiol. Infect., 2010, 16, 1620–1625 CrossRef CAS PubMed.
- G. Prod'hom, A. Bizzini, C. Durussel, J. Bille and G. Greub, MALDI-TOF mass spectrometry for direct bacterial identification from positive blood culture pellets, J. Clin. Microbiol., 2010, 48, 1481–1483 CrossRef.
- B. La Scola and D. Raoult, Direct identification of bacteria in positive blood culture bottles by matrix-assisted laser desorption ionisation time-of-flight mass spectrometry, PLoS One, 2009, 4, e8041 CrossRef.
- M. L. Faron, B. W. Buchan and N. A. Ledeboer, Matrix-assisted laser desorption ionization–time of flight mass spectrometry for use with positive blood cultures: methodology, performance, and optimization, J. Clin. Microbiol., 2017, 55, 3328–3338 CrossRef CAS.
- A. M. Bazzi,
et al., Comparison among four proposed direct blood culture microbial identification methods using MALDI-TOF MS, J. Infect. Public Health, 2017, 10, 308–315 CrossRef.
- L. Ferreira,
et al., Microorganisms direct identification from blood culture by matrix-assisted laser desorption/ionization time-of-flight mass spectrometry, Clin. Microbiol. Infect., 2011, 17, 546–551 CrossRef CAS.
- A. Fothergill,
et al., Rapid identification of bacteria and yeasts from positive-blood-culture bottles by using a lysis-filtration method and matrix-assisted laser desorption ionization–time of flight mass spectrum analysis with the SARAMIS database, J. Clin. Microbiol., 2013, 51, 805–809 CrossRef CAS PubMed.
- Y. W. Y. Tao, S. Huang, P. Zhu, W. E. Huang, J. Ling and J. Xu, Metabolic-activity based assessment of antimicrobial effects by D2O-labeled single-cell Raman microspectroscopy, Anal. Chem., 2017, 89, 4108–4115 CrossRef CAS PubMed.
- K. Yang,
et al. Rapid antibiotic susceptibility testing of pathogenic
bacteria using heavy-water-labeled single-cell Raman spectroscopy in clinical samples, Anal. Chem., 2019, 91, 6296–6303 CrossRef CAS PubMed.
- D. Bauer,
et al., Heteroresistant bacteria detected by an extended Raman-based antibiotic susceptibility test, Anal. Chem., 2020, 92, 8722–8731 CrossRef CAS PubMed.
- Y. Song,
et al., Raman-deuterium isotope probing for in-situ identification of antimicrobial resistant bacteria in Thames River, Sci. Rep., 2017, 7, 1–10 CrossRef.
- J. X. Cheng and X. S. Xie, Vibrational spectroscopic imaging of living systems: An emerging platform for biology and medicine, Science, 2015, 350, aaa8870 CrossRef PubMed.
- C. Zhang, D. Zhang and J. X. Cheng, Coherent Raman scattering microscopy in biology and medicine, Annu. Rev. Biomed. Eng., 2015, 17, 415–445 CrossRef CAS PubMed.
- C. L. Evans and X. S. Xie, Coherent anti-stokes Raman scattering microscopy: chemical imaging for biology and medicine, Annu. Rev. Anal. Chem., 2008, 1, 883–909 CrossRef CAS PubMed.
- M. Zhang,
et al., Rapid determination of antimicrobial susceptibility by stimulated Raman scattering imaging of D2O metabolic incorporation in a single bacterium, Adv. Sci., 2020, 7, 2001452 CrossRef CAS PubMed.
- W. Hong,
et al., Antibiotic susceptibility determination within one cell cycle at single-bacterium level by stimulated Raman metabolic imaging, Anal. Chem., 2018, 90, 3737–3743 CrossRef CAS PubMed.
- W. Hong,
et al., In situ detection of a single bacterium in complex environment by hyperspectral CARS imaging, ChemistrySelect, 2016, 1, 513–517 CrossRef CAS.
- C. W. Karanja,
et al., Stimulated Raman imaging reveals aberrant lipogenesis as a metabolic marker for azole-resistant Candida albicans, Anal. Chem., 2017, 89, 9822–9829 CrossRef CAS PubMed.
- Z. Weifeng,
et al., Rapid antimicrobial susceptibility testing by stimulated Raman scattering metabolic imaging and morphological deformation of bacteria, Anal. Chim. Acta, 2021, 1168, 338622 CrossRef PubMed.
- H. W. Pan,
et al., Simple sample preparation method for direct microbial identification and susceptibility testing from positive blood cultures, Front. Microbiol., 2018, 9, 481 CrossRef PubMed.
- M. Zhou,
et al., An improved in-house MALDI-TOF MS protocol for direct cost-effective identification of pathogens from blood cultures, Front. Microbiol., 2017, 8, 1824 CrossRef PubMed.
-
Performance standards for antimicrobial susceptibility testing, 30th ed CLSI supplement M100 Clinical and Laboratory Standards Institute, Clinical and Laboratory Standards Institute, Wayne, PA, 2020 Search PubMed.
Footnote |
† Electronic supplementary information (ESI) available. See DOI: 10.1039/d1an01210j |
|
This journal is © The Royal Society of Chemistry 2022 |