Insights into the binding manners of an Fe doped MOF-808 in high-performance adsorption: a case of antimony adsorption†
Received
22nd September 2021
, Accepted 24th November 2021
First published on 26th November 2021
Abstract
The full utilization of adsorption sites is crucial for adsorption technology. Metal–organic frameworks (MOFs), which are hotspot materials in environmental remediation, are not satisfactory in many cases because of their high specific surface area and relatively low adsorption capacity. In this work, a series of zirconium–iron bimetallic MOFs (ZrxFe(1−x)-MOF-808) was prepared. The optimal material (Zr0.8Fe0.2-MOF-808) possesses superb adsorption capacities of 524 and 310 mg g−1 for antimonate and antimonite, respectively. The carboxyl and hydroxyl groups were identified as the main adsorption sites by FTIR and XPS. The role of Fe in the adsorption process of Zr0.8Fe0.2-MOF-808 was further explored by DFT simulations, and four binding manners were discovered, consistent with the adsorption capacity data. Subsequently, application potential tests proved that the Zr0.8Fe0.2-MOF-808 shows good anti-interference ability, wide pH adaptability, and recyclability. Overall, this high-performance bimetallic MOF has been analyzed from the macrocosmic to the microcosmic, which provides certain support for the development of MOF modifications.
Environmental significance
With the rapid development of modern industry, a large amount of wastewater polluted by heavy metals is a thorny issue in the environment. From the cost-effectiveness and recovery point of view, adsorption is a suitable technology. Lots of adsorbents have been developed, including metal–organic frameworks (MOFs), which are hotspot materials in environmental remediation. However, it is challenging to find the direction of modification to tap the adsorption potential of MOFs and get insights into the mechanism of adsorption improvements. This work designed a bimetallic MOF (Zr0.8Fe0.2-MOF-808) based on the compatibility of metal nodes and ligands. As expected, Zr0.8Fe0.2-MOF-808 exhibits a superb adsorption performance (capacity and rate) for antimony. FTIR and XPS were carried out and it was found that the carboxyl and hydroxyl groups were the main adsorption sites. The role of doped Fe was further clarified with the aid of theoretical calculation. The binding manners were simulated and were consistent with the experimental results.
|
1. Introduction
Heavy metals, as a kind of common, unbiodegradable, and highly migratable pollutant, pose thorny issues in the environment, especially in water.1–3 Adsorption is an ordinary and important method for heavy metal ion removal and recycling, which has been extensively studied by many scientists owing to its simplicity, high efficiency, low cost, and recoverable features.4–7 How to maximize the adsorption capacity of adsorbents to the extreme based on their structure is a long-term research object in adsorption technology. Therefore, various synthetic and modification methods have been used to promote the adsorption capacity of materials, according to the following principles. (i) Specific surface area (SSA). The higher the SSA the greater the adsorption site exposure and the greater contact area, which lead to a higher adsorption capacity. High SSA can be achieved by reducing particle size, improving dispersion, increasing surface roughness, etc.8,9 Feng et al.10 successfully synthesized high-SSA Fe3O4 nanoparticles by ascorbic acid modification. The diameter of the as-synthesized material is less than 10 nm, resulting in a high SSA (179 m2 g−1), which gives it better adsorption performance for arsenic. Luo et al.11 prepared a new zirconium oxide carbon nanofiber material which makes a complementary combination of the zirconium oxide's adsorption performance on antimony and the ultra-high SSA of carbon nanofibers. The adsorbents possess good adsorption capacity for both Sb(III) and Sb(V). (ii) Creating specific adsorption sites. The high SSA causes the exposure of more adsorption sites, but the binding capabilities are different for diverse functional groups toward a certain pollutant. Specific adsorption sites will improve the efficiency of adsorption site, which increases the adsorption capacity. Shao et al.12 functionalized the surface of SiO2 with different groups (i.e., –EDTA (ethylenediamine triacetic acid), –COOH, –SO3H, –SH, and –NH2). The results showed that the adsorption capacity of SiO2 for Pb(II) was significantly promoted by EDTA functionalization. Obviously, the EDTA acted as the specific adsorption sites in this case, and there are many similar examples.13–15 (iii) Synergistic effects of adsorption sites. A synergistic effect may appear in an adsorbent with two or more adsorption sites. Guo et al.16 using 3D hierarchical Mg/Fe-LDH supported nanoscale hydroxyapatite materials to remove U(VI), found the excellent removal performance was achieved by a synergistic effect of the two combined materials.
Based on this background, we screened adsorbent materials by the above principles for the capture of metal oxyanions from aqueous solution. As a zirconium-based MOF material, MOF-808 (Zr6O4(OH)4(BTC)2(HCOO)6)17 has attracted more and more attention due to its good chemical stability, high SSA, simple synthesis method, and diversified adsorption sites. The octahedral structure of the SBU (secondary building units) and exposed carboxyl adsorption sites make MOF-808 an excellent material for the adsorption of heavy metals.17,18 Li et al.19 used MOF-808 to remove arsenic, where the adsorption capacity was 24.83 mg g−1. However, the adsorption ability of this raw MOF material for heavy metal ions is not satisfactory, and therefore many researchers are working on device fabrication for MOFs to improve them, e.g. MOF-based membranes.20,21 As a great potential material, another approach to promote the capture ability of MOF-808 is modification. Researchers have put a lot of effort into modifying MOFs, including ligand exchange,22 functional group grafting,23 doping,24 and hybrid materials,25 some of which have achieved good results. However, although they often involved one or two of the principles mentioned above, it is very difficult to integrate all three principles.
In order to tap the adsorption potential of MOF-808, Fe, which forms an SBU of MIL-100 was selected, as a binding partner for MOF-808 to form bimetallic MOFs in this study. There are three reasons: (1) from the perspective of structural composition, MOF-808 and MIL-100(Fe) are made up of the same organic ligand (benzenetricarboxylic acid), which indicates that the two MOFs may be compatible with each other and lead to an ideal structure with a high SSA.26 (2) MIL-100(Fe) shows good adsorption ability towards heavy metals in oxyanionic form. Wang et al.27 synthesized an MIL-100(Fe) filter for arsenic removal; the bimetallic MOFs may generating specific adsorption sites for oxyanions. (3) The Fe atom may substitute Zr in the SBU of MOF-808, which may give rise to a synergistic effect in heavy metal adsorption.28 Combining all these desirable features, we selected Fe doping on MOF-808 to form a new bimetallic MOF, and the hypothesis mentioned above will be verified.
As a harmful and typical oxyanion pollutant, antimony has gradually attracted widespread attention, and was chosen to be the target oxyanion pollutant in this work.29 Herein, a series of bimetallic MOF materials (ZrxFe(1−x)-MOF-808) were synthesized and their adsorption performance for antimony evaluated. The material with the optimal Zr
:
Fe ratio was selected for further investigation. We used XRD, BET, XPS etc. to explore the adsorption mechanism of bimetallic MOF. The microscopic adsorption process was further studied by DFT calculations. Finally, we verified the environmental application potential of this material by interfering ion tests, pH tests, and repeatability experiments.
2. Materials and methods
2.1 Chemicals
Details of the relevant chemicals and materials are listed in section S1.†
2.2 Synthesis of MOF-808 and ZrxFe(1−x)-MOF-808
The details of MOF-808 synthesis are provided in section S2.† A series of bimetallic MOFs were prepared through modification of the method for producing MOF-808. For example, H3BTC (0.8405 g, 4 mmol), ZrOCl2·8H2O (1.293 g, 4 mmol) and FeCl3(0.6488 g, 4 mmol) in a solvent mixture of DMF/formic acid (60 mL/60 mL) were placed in a 200 mL Teflon-lined stainless steel autoclave, which was heated at 135 °C for 2 days. Shallow orange crystals were collected and washed three times with 20 mL of fresh DMF. The obtained products were denoted as ZrxFe(1−x)-MOF-808. Five bimetallic MOF materials were prepared in this paper, x = 0.2, 0.4, 0.5, 0.8, 0.9, respectively.
2.3 Characterizations
Details of the characterizations are given in section S3.†
2.4 Adsorption properties tests
These included a study of adsorption isotherms and adsorption kinetics. The procedures are provided in section S4.†
2.5 Application potential tests
These included interfering ion tests, pH tests, and repeatability tests. The details are provided in section S5.†
2.6 Theoretical computation
The processes of Sb(V) and Sb(III) adsorption on MOF-808 and Zr0.8Fe0.2-MOF-808 were explored using density functional theory (DFT) calculations. The Vienna ab initio simulation package (VASP)30 and the projector augmented wave (PAW) method were employed. The van der Waals diameters of Sb(OH)6− and Sb(OH)3 were calculated by Multiwfn.31 More computational details are described in section S6.†
The adsorption energy (Ead) was calculated using
Ead = E(total) − E(Sb) − E(MOF) |
where
E(total),
E(MOF),
E(Sb) are respectively the total energy of the adsorption complex, the adsorbent, and the adsorbate.
3. Results and discussion
3.1 Characterization of bimetallic MOFs
In this paper, six kinds of MOFs were synthesized. Fig. 1a shows the powder X-ray diffraction (PXRD) patterns of ZrxFe(1−x)-MOF-808 (x = 0.2, 0.4, 0.5, 0.8, 0.9) and pristine MOF-808. In the angle range of 5–30°, it is obvious that the characteristic peaks of ZrxFe(1−x)-MOF-808 and MOF-808 are almost the same, which suggests that the crystal structure of the material is negligibly changed after Fe doping. PXRD confirmed that the structure of MOF-808 is retained after Fe doping, but the role of Fe is unclear. It possibly exists as amorphous Fe-oxides or in the SBU. To further explore the materials, BET analysis was conducted to obtain data about SSA and pore size, which are shown in Fig. 1b and c. The SSAs of these six materials are 711.9, 741.3, 923.4, 1530.6, 1176.6, 500.2 m2 g−1, respectively. With an increase in amount of Zr, the SSA of this bimetallic MOFs first increases and then decreases, with the maximum specific surface area being 1530.6 m2 g−1 for Zr0.8Fe0.2-MOF-808. It can be seen that the SSA of the original material is greatly expanded through the doping with Fe. In addition, from the nearly uniform pore size of 4–5 nm of all the materials, it can be inferred that the configuration of the materials is basically unchanged through doping, which matches the XRD results. Therefore, it can be speculated that Fe exists in the SBU instead of as amorphous Fe-oxides. With the best crystalline structure and the largest SSA, Zr0.8Fe0.2-MOF-808 was expected to exhibit the best adsorption performance.
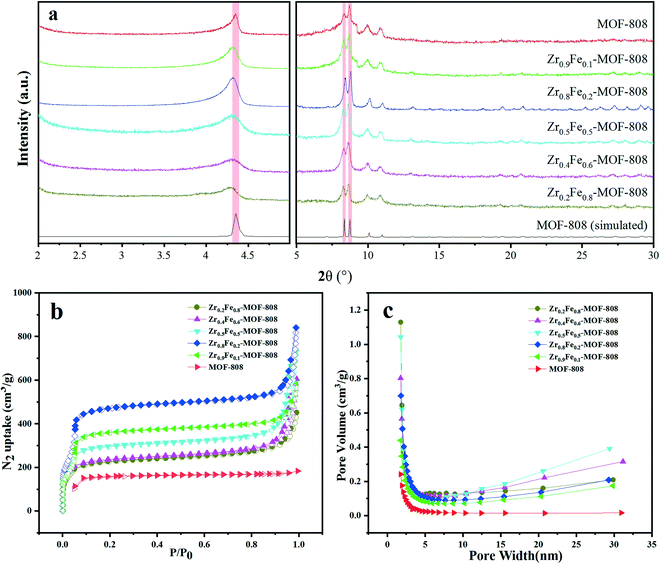 |
| Fig. 1 Characterization of ZrxFe(1−x)-MOF-808 (x = 0.2, 0.4, 0.5, 0.8, 0.9) and MOF-808. (a) PXRD patterns. (b) Specific surface areas. (c) Pore sizes. | |
3.2 Adsorption properties for Sb(V) and Sb(III)
The adsorption isothermals of all the ZrxFe(1−x)-MOF-808 to Sb(V) and Sb(III) are shown in Fig. 2. Fig. 2a shows that all bimetallic MOFs are superior to pristine MOF-808 in adsorption capacity for Sb(V), among which Zr0.8Fe0.2-MOF-808 shows a significantly superior adsorption capacity to the other materials, and its adsorption capacity reaches as high as 524 mg g−1. This indicates that the doping of Fe in MOF-808 can greatly enhance its adsorption capacity against Sb(V). In the case of Sb(III), Zr0.8Fe0.2-MOF-808 is still outstanding, but the adsorption capacity of the other materials is not much different (Fig. 2b). The adsorption capacity of Zr0.8Fe0.2-MOF-808 is 310 mg g−1, which is far beyond those of MOF-808 (282 and 204 mg g−1) and is relatively rare in previous reports32–47 (Fig. 2c).
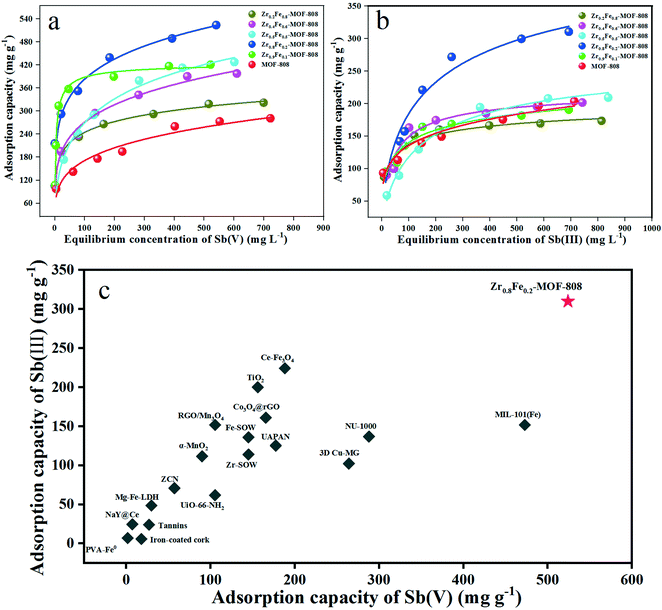 |
| Fig. 2 Adsorption isotherms of (a) Sb(V) and (b) Sb(III) on all materials; (c) Sb removal performance of various adsorbents. | |
Langmuir and Freundlich models were used to fit the isothermal adsorption data (Fig. S1†), and the fitting parameters are shown in Table S1.† The correlation coefficient (R2) suggests that the adsorption process was better described by the Langmuir model than by the Freundlich model. This indicates a monolayer adsorption process. The theoretical adsorption capacities of Zr0.8Fe0.2-MOF-808 were 526.3 and 343.6 mg g−1 for Sb(V) and Sb(III), respectively.
In practical engineering, the adsorption rate is also an important factor to measure the utilization potentiality. Adsorption kinetic parameters are essential for designing treatment units and determining operating parameters. It can be seen from kinetic experiments that Zr0.8Fe0.2-MOF-808 is superior to MOF-808, in terms of both adsorption capacity and adsorption rate. The adsorption quantities of Sb(V) and Sb(III) by Zr0.8Fe0.2-MOF-808 increased rapidly for the initial 20 min. After that, the adsorption rates slowed down to equilibrium in about 60 min (Fig. S2†). Pseudo-first-order and pseudo-second-order models were used to fit the kinetic data, and all the parameters are shown in Table S2.† The results indicate that the adsorption process is more consistent with the pseudo-second-order model.
3.3 Exploring the adsorption mechanism by characterization
Owing to the Fe doping, Zr0.8Fe0.2-MOF-808 exhibits ultrahigh removal efficiency toward Sb(V) and Sb(III), which is superior to most existing adsorbents. It can be further speculated that the Zr in the SBU was replaced by Fe and subsequently changed the bonding conditions of MOF-808 to Sb(V) and Sb(III).
To explain this unpredictable phenomenon and the role of Fe in the adsorption process, FT-IR was carried out on Zr0.8Fe0.2-MOF-808 and MOF-808 before and after adsorption of antimony, as shown in Fig. 3a. Comparing MOF-808 and Zr0.8Fe0.2-MOF-808 before adsorption, it can be seen that the IR spectra of both are basically the same, indicating that the doping with Fe did not change the general structure and the intensity of the functional groups of MOF-808. This situation also indirectly reflects that Fe enters into the SBU of MOF-808 in a small amount. The lower part of Fig. 3a is an infrared comparison before and after adsorption by MOF-808. It can be seen that the change in peak shape is basically the same after antimony adsorption, mainly appearing at 2876 cm−1 and 1600 cm−1, corresponding to the vibration peaks of hydroxyl and carboxyl groups, respectively. According to all possible adsorption sites of MOF-808, it mainly uses hydroxyl as an adsorption site and ligand exchange in the process of adsorbing antimony.48,49 The upper part is the infrared comparison before and after adsorption by Zr0.8Fe0.2-MOF-808. The position of the changed peak is similar to MOF-808, which indicates that Zr0.8Fe0.2-MOF-808 also adsorbs antimony mainly by the hydroxyl functional group and ligand exchange. This result indicates that the doping with Fe in the bimetallic MOF did not change the adsorption mode of MOF-808, but only enhanced the original adsorption mode.
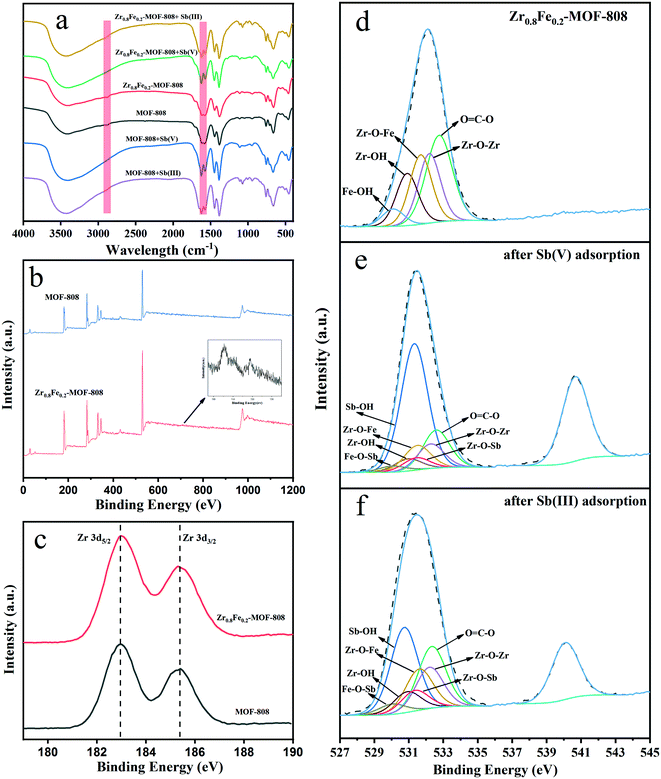 |
| Fig. 3 (a) FT-IR spectra of adsorbents before and after antimony adsorption. (b–f) XPS characterization of Zr0.8Fe0.2-MOF-808 and MOF-808 before and after antimony adsorption. | |
FT-IR provided some information about the doping position of Fe and the antimony adsorption process, but it is beneficial to use XPS to confirm the results and dig deeper. The peaks corresponding to Zr, O, C, and Fe can be identified in the survey scan spectra (Fig. 3b). Zr0.8Fe0.2-MOF-808 and MOF-808 have similar element peaks except there is an extra Fe peak in Zr0.8Fe0.2-MOF-808, as expected. It worth noting that the binding energy of Zr has slightly changed in Zr0.8Fe0.2-MOF-808: the binding energy of Zr5/2 increases by 0.1 eV, while that of Zr3/2 decreases by 0.1 eV, as shown in Fig. 3c. This must be ascribed to the replacement of Fe in the SBU, which affects the Zr binding circumstance. Compared with raw Zr0.8Fe0.2-MOF-808 (Fig. 3d), the peaks of O 1s after Sb(V) (Fig. 3e) and Sb(III) (Fig. 3f) adsorption have changed. It can be seen that there are significant shifts in O
C–O, Zr–OH, and Fe–OH. The binding energies of Sb(V) have changes of 0.23, 0.32, 0.22 eV, and Sb(III) has changes of 0.11, 0.33, 0.21 eV, respectively. This shift indicated that hydroxyl and carboxyl play leading roles in antimony adsorption. According to the above-mentioned results, hydroxyl binding and ligand exchange (carboxyl which binds with the Zr6-cluster is replaced by antimony oxyanions) occurred during the process of antimony adsorption in Zr0.8Fe0.2-MOF-808. Comparing the O 1s peaks of MOF-808 before and after adsorption of antimony (Table S3†), it can be seen that the main change lies in O
C–O and Zr–OH, further demonstrating that the original MOF-808 also removes antimony through ligand exchange and hydroxyl complexation.
3.4 Exploring the binding manners by DFT calculation
FTIR and XPS produced a series of qualitative results, which proved our hypothesis. However, the exact binding structure and adsorption energy are still unknown. According to the topology of MOF-808 obtained from CCDC, one of the SBU of MOF-808 was obtained after separating and optimizing. The element ratio of Zr to Fe in this material is about 5
:
1, measured from SEM-EDS analysis (Fig. S3†). Based on the Zr6-cluster of MOF-808, it can be inferred that one Fe atom replaces one Zr atom of the six in the Zr6-cluster. Therefore, the SBU of Zr0.8Fe0.2-MOF-808 was constructed by replacing one of the Zr atoms with Fe, and this optimized structure is shown in Fig. S4a.† The optimized structures of Sb(OH)6− and Sb(OH)3 are shown in Fig. S4c and d.† In order to ensure that the pore size of MOF-808 is large enough for the adsorbate to diffuse, we calculated the van der Waals diameters of Sb(OH)6− and Sb(OH)3 (7.22 and 6.64 Å, Fig. S5 and S6†), and they are both smaller than the internal pore diameter (18.4 Å) of MOF-808.17
According to the characterization data and the structures we built, there were three sites of MOF-808 for antimony adsorption: (1) the hydroxyl groups in the SBU can bind to antimony with monodentate or bidentate form (Fig. 4, manner 1).35,48 (2) The electronegative O in Zr–O–Zr can attract antimony (Fig. 4, manner 2).49 (3) Ligand exchange: carboxylic groups of benzenetricarboxylic acid, which were linked to zirconium atoms can be replaced by antimony (Fig. 4, manner 3).49 For Zr0.8Fe0.2-MOF-808, besides the three adsorption sites, an additional site may exist. The adsorption mechanism of MIL-100 for heavy metal ions is mainly to use hydroxyl groups existing in the framework and oxyacid radicals of metal ions to achieve a binding effect under the condition of losing one molecule of water.27 Therefore, the Fe atom in the SBU of Zr0.8Fe0.2-MOF-808 may still adsorb antimony in the same way (Fig. 4, manner 4). Combining all the above approaches, it can be assumed that the remarkable increase in adsorption performance of Zr0.8Fe0.2-MOF-808 is due to the adsorption sites of the original MOF-808 and the adsorption sites of the Fe atoms doped in MOF-808 simultaneously acting on antimony adsorption.
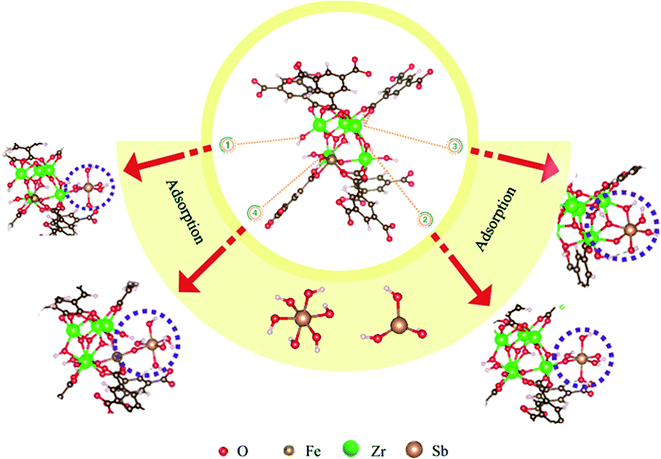 |
| Fig. 4 Schematic diagram of adsorption mechanisms. | |
To verify the reasonableness of this conclusion in terms of binding modes and adsorption energy, the adsorption energies (Ead) of antimony on MOF-808 and Zr0.8Fe0.2-MOF-808 were evaluated by DFT calculation. As shown in Fig. 5, based on the speculation about the adsorption mode discussed above, four adsorption methods were calculated. The higher the absolute value, the more stable the combination. As one of the most conventional adsorption sites in Zr-MOFs, Zr-OH in MOF-808 adsorbs one molecule of Sb(V) in the absence of one molecule of water through monodentate and mononuclear binding. The adsorption energy is −1.27 eV (Fig. 5a). For Zr0.8Fe0.2-MOF-808, this adsorption mode is somewhat enhanced by doping with Fe, with an adsorption energy of −1.69 eV (Fig. 5b). The bond lengths are shown in Table S4.† O in the Zr–O–Zr of the SBU serves as a possible second adsorption site. According to the results of the DFT calculation, if O in Zr–O–Zr is to be used as an adsorption site, Zr–OH is needed as a support site to form a tetrameric ring for the Sb(V) adsorption. This adsorption method will have a higher priority than that of Zr–OH for a much higher adsorption energy (−3.15 eV, Fig. 5a). This adsorption method is different for Zr0.8Fe0.2-MOF-808. If O in Zr–O–Zr is used as an adsorption site, two Zr–OHs are required to interact with an antimony root to form two spliced four-membered rings, which have the highest absolute value of adsorption energy (−3.40 eV, Fig. 5a) and provide the most stable binding method. The third adsorption method is based on ligand exchange. The optimization of the model after adsorption shows that one molecule of Sb(V) replaces one carboxylic group of the ligand (H3BTC) in MOF-808 or Zr0.8Fe0.2-MOF-808 to form a stable structure. The adsorption energies of MOF-808 and Zr0.8Fe0.2-MOF-808 are −2.73 eV and −2.88 eV, respectively, and the bond lengths are shown in Table S4.† The fourth adsorption mode is unique to Zr0.8Fe0.2-MOF-808. Due to the existence of an Fe atom in the SBU, the Fe atom in the SBU can use its own –OH to adsorb Sb(V) one to one. The adsorption energy of Fe–O–Sb (−1.99 eV) is higher than that of Zr–O–Sb (1.69 eV) in mode 1, which means that this adsorption mode is more stable and direct. In a previous report, Li et al.35 utilized another common Zr-MOF, named NU-1000, for the adsorption of antimony. During speculation about the mechanism, the authors proposed a two-dentate mononuclear adsorption, i.e., the adsorption of one antimony by two Zr–OHs.
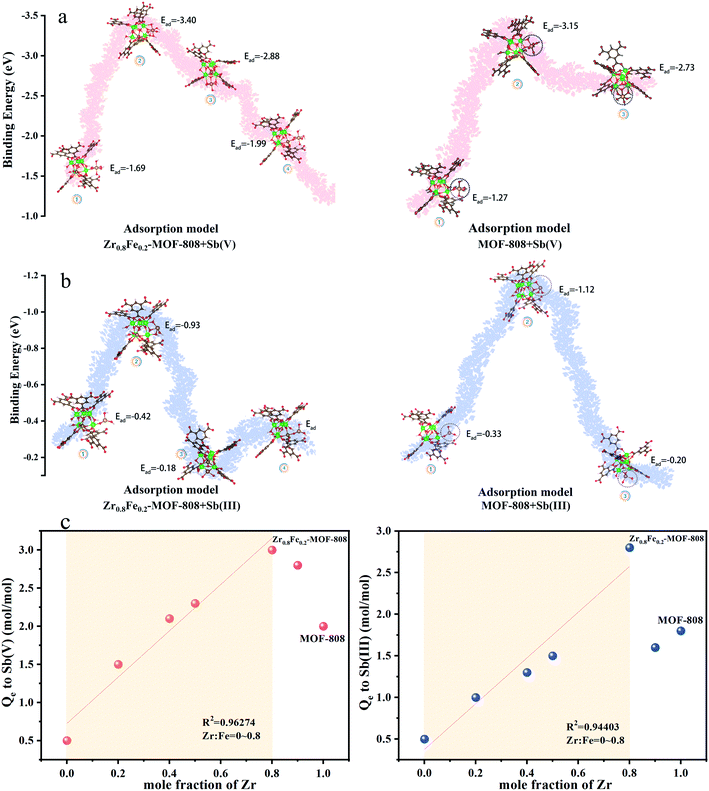 |
| Fig. 5 Adsorption energies of the four adsorption methods and the adsorption amounts of (a) Sb(V) and (b) Sb(III); (c) relationship between adsorption capacity and mole fraction of Zr in each material. | |
In summary of the adsorption modes, Zr0.8Fe0.2-MOF-808 relies on adsorption methods 2, 3, and 4 in the adsorption process; while MOF-808 mainly uses adsorption modes 2 and 3. The adsorption energies of the four adsorption modes of Sb(III) adsorption (Fig. 5b) show a generally consistent trend with Sb(V). The adsorption models and energies for all adsorption methods are shown in Fig. S5 and S6.† The results of the bond length are shown in Table S4.†
In order to validate the consistency of the theoretical calculation results and the actual adsorption behavior, the antimony adsorption capacities of all the adsorbents were normalized, which were converted (mg Sb)/(g MOF) to (mol Sb)/(mol SBU), as shown in Fig. 5c and d. The molar ratio of Zr
:
Mtotal (Mtotal = Fe + Zr) and the adsorption amount of the material showed a positive proportion trend in the range of 0.2–0.8 (R2Sb(V) = 0.994, R2Sb(III) = 0.933). When the Zr proportion is 0.8, the synergistic effect of Fe and Zr reaches an optimum point. When the Zr proportion exceeds 0.8, the adsorption amount of the material shows a downward trend. This may be ascribed to the Fe content being too low to produce synergistic effects. The normalized molar adsorption amounts of Zr0.8Fe0.2-MOF-808 and MOF-808 are consistent with the trend in isothermal adsorption. Most important of all, it can be seen that the mole ratio of adsorbed antimony to SBU of MOF-808 can be increased from 2
:
1 to 3
:
1 after Fe doping, which means that 1 mol per SBU of Zr0.8Fe0.2-MOF-808 can bind 3 mol of antimony, while MOF-808 can only bind 2 mol per SBU.
3.5 Application potential tests
High adsorption capacity is not enough for engineering applications. Interfering ions are another important factor for the application potential of adsorbents. The influence of PO43−, SO42−, NO3−, and F− as co-existing anions on the antimony adsorption by Zr0.8Fe0.2-MOF-808 was further investigated. Fig. 6a indicates that NO3− can promote the adsorption performance for Sb(V). However, Sb(V) adsorption was suppressed by PO43− and F−, which is ascribed to competitive adsorption, because Zr-MOF has good affinity to phosphate50 and fluoride.51 For the case of Sb(III), the adsorption capacity of Zr0.8Fe0.2-MOF-808 is little affected and shows good stability in Fig. 6b.
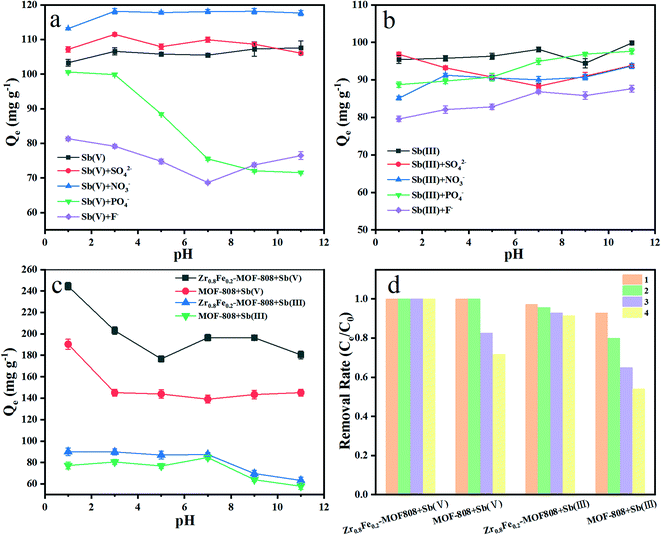 |
| Fig. 6 Competitive adsorption of Sb(V) (a) Sb(III) (b); (c) effect of pH; (d) four times recycling of adsorbents. | |
The pH will impact the surface charge characteristics and decide the species of adsorbate anions. The adsorption capacity of Zr0.8Fe0.2-MOF-808 for Sb(V) and Sb(III) is greater than that of MOF-808 in the pH range from 1 to 11 (Fig. 6c).
The desorption and recyclability of Zr0.8Fe0.2-MOF-808 and MOF-808 were also studied. As shown in Fig. 6d, the removal efficiency by Zr0.8Fe0.2-MOF-808 of Sb(V) can still reach 100% after four adsorption cycles. However, the removal efficiency of MOF-808 dropped to about 80% after the third adsorption. In the case of Sb(III), the removal efficiency of Zr0.8Fe0.2-MOF-808 is more than 90% after the four adsorption cycles. While the removal efficiency of MOF-808 dropped to about 65% after the third adsorption. Therefore, Zr0.8Fe0.2-MOF-808 exhibits a superb reuse capability compared to pristine MOF-808.
4. Conclusions
MOFs have been hotspot materials for environmental remediation in recent years, so it is significant to find a way to harness the potential of MOFs. In this work, a new bimetallic MOF was successfully prepared, and after adjusting the ratio of Zr and Fe, 8:2 (Zr0.8Fe0.2-MOF-808) was found to be the best proportion. By taking advantage of the substitution of Fe atoms on the SBU of MOF-808, Zr0.8Fe0.2-MOF-808 exhibits an ultra-high adsorption capacity for antinomy. The material also possesses excellent properties in terms of adsorption rate and regeneration ability. The adsorption mechanism was explored by XRD, FT-IR, XPS, etc. The adsorption model was further illustrated through theoretical calculations, and the adsorption energy and bond length were also obtained. The adsorption mode of MOF-808 to antimony is ligand exchange and complexation. However, Fe doping in Zr0.8Fe0.2-MOF-808 brings about a new adsorption site, which maximizes the adsorption performance of the material. Environmental application factors such as selectivity, pH, and repeatability were further tested and showed satisfactory results. In practical applications, this material can be applied in the form of dosing-filtration, fixed bed, or MOF-membrane, and the latter is the most promising. Through this work, we not only obtained an excellent adsorbent for antimony but also offer a feasible and effective way to maximize the adsorption performance of MOFs.
Conflicts of interest
There are no conflicts of interest to declare.
Acknowledgements
This study was financially supported by the National Science Fund for Distinguished Young Scholars (No. 52125002), the National Key Research and Development Program of China (No. 2019YFC1907900), the National Science Foundation of China (No. 41877115, 52060018), Jiangxi Provincial Natural Science Foundation (No. 20202BABL204061), the Key Project of Science and Technology Department of Jiangxi Province (No. 20171ACG70021), and the Key Research and Development Program of Inner Mongolia Autonomous Region (No. 2019ZD001).
References
- Q. Zhou, N. Yang, Y. Li, B. Ren, X. Ding and H. Bian,
et al., Total concentrations and sources of heavy metal pollution in global river and lake water bodies from 1972 to 2017, Glob. Ecol. Conserv., 2020, 22, e925 Search PubMed.
- D. Xu, R. Fu, H. Liu and X. Guo, Current knowledge from heavy metal pollution in Chinese smelter contaminated soils, health risk implications and associated remediation progress in recent decades: A critical review, J. Cleaner Prod., 2020, 124989 Search PubMed.
- J. P. Vareda, A. J. M. Valente and L. Durães, Assessment of heavy metal pollution from anthropogenic activities and remediation strategies: A review, J. Environ. Manage., 2019, 246, 101–118 CrossRef CAS PubMed.
- N. Zhang, A. Ishag, Y. Li, H. Wang, H. Guo and P. Mei,
et al., Recent investigations and progress in environmental remediation by using covalent organic framework-based adsorption method: A review, J. Cleaner Prod., 2020, 277, 123360 CrossRef CAS.
- J. Ru, X. Wang, F. Wang, X. Cui, X. Du and X. Lu, UiO series of metal-organic frameworks composites as advanced sorbents for the removal of heavy metal ions: Synthesis, applications and adsorption mechanism, Ecotoxicol. Environ. Saf., 2021, 208, 111577 CrossRef CAS PubMed.
- K. C. Lai, L. Y. Lee, B. Y. Z. Hiew, S. Thangalazhy-Gopakumar and S. Gan, Environmental application of three-dimensional graphene materials as adsorbents for dyes and heavy metals: Review on ice-templating method and adsorption mechanisms, J. Environ. Sci., 2019, 79, 174–199 CrossRef PubMed.
- J. Guo, X. Xu, J. P. Hill, L. Wang, J. Dang and Y. Kang,
et al., Graphene-carbon 2D heterostructures with hierarchically-porous P,N-doped layered architecture for capacitive deionization, Chem. Sci., 2021, 12(30), 10334–10340 RSC.
- K. A. G. Katsogiannis, G. T. Vladisavljević, S. Georgiadou and R. Rahmani, Assessing the Increase in Specific Surface Area for Electrospun Fibrous Network due to Pore Induction, ACS Appl. Mater. Interfaces, 2016, 8(42), 29148–29154 CrossRef CAS PubMed.
- A. E. A. Aboubakr, W. M. A. El Rouby, M. D. Khan, A. A. Farghali and N. Revaprasadu, ZnCr-CO3 LDH/ruptured tubular g-C3N4 composite with increased specific surface area for enhanced photoelectrochemical water splitting, Appl. Surf. Sci., 2020, 508, 145100 CrossRef CAS.
- L. Feng, M. Cao, X. Ma, Y. Zhu and C. Hu, Superparamagnetic high-surface-area Fe3O4 nanoparticles as adsorbents for arsenic removal, J. Hazard. Mater., 2012, 217-218, 439–446 CrossRef CAS PubMed.
- J. Luo, X. Luo, J. Crittenden, J. Qu, Y. Bai and Y. Peng,
et al., Removal of Antimonite (Sb(III)) and Antimonate (Sb(V)) from Aqueous Solution Using Carbon Nanofibers That Are Decorated with Zirconium Oxide (ZrO2), Environ. Sci. Technol., 2015, 49(18), 11115 CrossRef CAS PubMed.
- P. Shao, D. Liang, L. Yang, H. Shi, Z. Xiong and L. Ding,
et al., Evaluating the adsorptivity of organo-functionalized silica nanoparticles towards heavy metals: Quantitative comparison and mechanistic insight, J. Hazard. Mater., 2019, 121676 Search PubMed.
- A. Bhatnagar, W. Hogland, M. Marques and M. Sillanpää, An overview of the modification methods of activated carbon for its water treatment applications, Chem. Eng. J., 2013, 219, 499–511 CrossRef CAS.
- R. M. Rego, G. Kuriya, M. D. Kurkuri and M. Kigga, MOF based engineered materials in water remediation: Recent trends, J. Hazard. Mater., 2021, 403, 123605 CrossRef CAS PubMed.
- Z. Yao, P. Shao, D. Fang, J. Shao, D. Li and L. Liu,
et al., Thiol-rich, porous carbon for the efficient capture of silver: Understanding the relationship between the surface groups and transformation pathways of silver, Chem. Eng. J., 2022, 427, 131470 CrossRef CAS.
- Y. Guo, Z. Gong, C. Li, B. Gao, P. Li and X. Wang,
et al., Efficient removal of uranium (VI) by 3D hierarchical Mg/Fe-LDH supported nanoscale hydroxyapatite: A synthetic experimental and mechanism studies, Chem. Eng. J., 2020, 392, 123682 CrossRef CAS.
- H. Furukawa, F. Gándara, Y. Zhang, J. Jiang, W. L. Queen and M. R. Hudson,
et al., Water adsorption in porous metal-organic frameworks and related materials, J. Am. Chem. Soc., 2014, 136(11), 4369–4381 CrossRef CAS PubMed.
- Y. Bai, Y. Dou, L. Xie, W. Rutledge, J. Li and H. Zhou, Zr-based metal-organic frameworks: design, synthesis, structure, and applications, Chem. Soc. Rev., 2016, 45(8), 2327–2367 RSC.
- Z. Li, J. Yang, K. Sui and N. Yin, Facile synthesis of metal-organic framework MOF-808 for arsenic removal, Mater. Lett., 2015, 160, 412–414 CrossRef CAS.
- J. E. Efome, D. Rana, T. Matsuura and C. Q. Lan, Insight studies on metal-organic framework nanofibrous membrane adsorption and activation for heavy metal ions removal from aqueous solution, ACS Appl. Mater. Interfaces, 2018, 10(22), 18619–18629 CrossRef CAS PubMed.
- X. Li, Y. Liu, J. Wang, J. Gascon, J. Li and B. Van der Bruggen, Metal-organic frameworks based membranes for liquid separation, Chem. Soc. Rev., 2017, 46(23), 7124–7144 RSC.
- W. Zhang, A. Bu, Q. Ji, L. Min, S. Zhao and Y. Wang,
et al., pKa-Directed incorporation of phosphonates into MOF-808 via ligand exchange: stability and adsorption properties for uranium, ACS Appl. Mater. Interfaces, 2019, 11(37), 33931–33940 CrossRef CAS PubMed.
- R. Marlow, S. Kuriyakose, N. Mesaros, H. H. Han, R. Tomlinson and S. N. Faust,
et al., A phase III, open-label, randomised multicentre study to evaluate the immunogenicity and safety of a booster dose of two different reduced antigen diphtheria-tetanus-acellular pertussis-polio vaccines, when co-administered with measles-mumps-rubella vaccine in 3 and 4-year-old healthy children in the UK, Vaccine, 2018, 36(17), 2300–2306 CrossRef CAS PubMed.
- W. Xiao, X. Jiang, X. Liu, W. Zhou, Z. N. Garba and I. Lawan,
et al., Adsorption of organic dyes from wastewater by metal-doped porous carbon materials, J. Cleaner Prod., 2020, 124773 Search PubMed.
- Z. Wang, X. Xu, J. Kim, V. Malgras, R. Mo and C. Li,
et al., Nanoarchitectured metal–organic framework/polypyrrole hybrids for brackish water desalination using capacitive deionization, Mater. Horiz., 2019, 6(7), 1433–1437 RSC.
- P. Horcajada, S. Surble, C. Serre, D. Y. Hong, Y. K. Seo and J. S. Chang,
et al., Synthesis and catalytic properties of MIL-100(Fe), an iron(III) carboxylate with large pores, Chem. Commun., 2007, 27, 2820–2822 RSC.
- D. Wang, S. E. Gilliland, X. Yi, K. Logan, D. R. Heitger and H. R. Lucas,
et al., Iron mesh-based metal organic framework filter for efficient arsenic removal, Environ. Sci. Technol., 2018, 52(7), 4275–4284 CrossRef CAS PubMed.
- S. Yuan, J. Peng, Y. Zhang and Y. Shao-Horn, Stability trend of metal–organic frameworks with heterometal-modified hexanuclear Zr building units, J. Phys. Chem. C, 2019, 123(46), 28266–28274 CrossRef CAS.
- X. Long, X. Wang, X. Guo and M. He, A review of removal technology for antimony in aqueous solution, J. Environ. Sci., 2020, 90, 189–204 CrossRef PubMed.
- G. Kresse and J. Furthmüller, Efficient iterative schemes for ab initio total-energy calculations using a plane-wave basis set, Phys. Rev. B: Condens. Matter Mater. Phys., 1996, 54(16), 11169–11186 CrossRef CAS PubMed.
- T. Lu and F. Chen, Multiwfn: a multifunctional wavefunction analyzer, J. Comput. Chem., 2012, 33(5), 580–592 CrossRef CAS PubMed.
- X. Zhao, X. Dou, D. Mohan, C. U. Pittman, Y. S. Ok and X. Jin, Antimonate and antimonite adsorption by a polyvinyl alcohol-stabilized granular adsorbent containing nanoscale zero-valent iron, Chem. Eng. J., 2014, 247, 250–257 CrossRef CAS.
- X. He, X. Min and X. Luo, Efficient Removal of Antimony (III, V) from contaminated water by amino modification of a zirconium metal–organic framework with mechanism study, J. Chem. Eng. Data, 2017, 62(4), 1519–1529 CrossRef CAS.
- L. Yan, J. Song, T. Chan and C. Jing, Insights into antimony adsorption on {001} TiO2: XAFS and DFT study, Environ. Sci. Technol., 2017, 51(11), 6335–6341 CrossRef CAS PubMed.
- J. Li, X. Li, T. Hayat, A. Alsaedi and C. Chen, Screening of zirconium-based metal–organic frameworks for efficient simultaneous removal of antimonite (Sb(III)) and antimonate (Sb(V)) from aqueous solution, ACS Sustainable Chem. Eng., 2017, 5(12), 11496–11503 CrossRef CAS.
- Y. Tu, L. Ren, Y. Lin, J. Shao, Y. He and X. Gao,
et al., Adsorption of antimonite and antimonate from aqueous solution using modified polyacrylonitrile with an ultrahigh percentage of amidoxime groups, J. Hazard. Mater., 2020, 388, 121997 CrossRef CAS PubMed.
- R. Yan, Z. Qiu, X. Bian, J. Yang, S. Lyu and A. Zhou, Effective adsorption of antimony from aqueous solution by cerium hydroxide loaded on Y-tape molecular sieve adsorbent: Performance and mechanism, Colloids Surf., A, 2020, 604, 125317 CrossRef CAS.
- B. K. Biswas, J. Inoue, H. Kawakita, K. Ohto and K. Inoue, Effective removal and recovery of antimony using metal-loaded saponified orange waste, J. Hazard. Mater., 2009, 172(2–3), 721–728 CrossRef CAS PubMed.
- H. Jiang, L. Tian, P. Chen, Y. Bai, X. Li and H. Shu,
et al., Efficient antimony removal by self-assembled core-shell nanocomposite of Co3O4@rGO and the analysis of its adsorption mechanism, Environ. Res., 2020, 187, 109657 CrossRef CAS PubMed.
- D. You, X. Min, L. Liu, Z. Ren, X. Xiao and S. G. Pavlostathis,
et al., New insight on the adsorption capacity of metallogels for antimonite and antimonate removal: From experimental to theoretical study, J. Hazard. Mater., 2018, 346, 218–225 CrossRef CAS PubMed.
- H. Bacelo, B. R. C. Vieira, S. C. R. Santos, R. A. R. Boaventura and C. M. S. Botelho, Recovery and valorization of tannins from a forest waste as an adsorbent for antimony uptake, J. Cleaner Prod., 2018, 198, 1324–1335 CrossRef CAS.
- W. Zhang, N. Li, T. Xiao, W. Tang and G. Xiu, Removal of antimonite and antimonate from water using Fe-based metal-organic frameworks: The relationship between framework structure and adsorption performance, J. Environ. Sci., 2019, 86, 213–224 CrossRef PubMed.
- A. M. A. Pintor, B. R. C. Vieira, R. A. R. Boaventura and C. M. S. Botelho, Removal of antimony from water by iron-coated cork granulates, Sep. Purif. Technol., 2020, 233, 116020 CrossRef CAS.
- Y. Cao, Q. Guo, M. Liang and W. Sun, Sb(III) and Sb(V) removal from water by a hydroxyl-intercalated, mechanochemically synthesized Mg-Fe-LDH, Appl. Clay Sci., 2020, 196, 105766 CrossRef CAS.
- Z. Qi, T. P. Joshi, R. Liu, H. Liu and J. Qu, Synthesis of Ce(III)-doped Fe3O4 magnetic particles for efficient removal of antimony from aqueous solution, J. Hazard. Mater., 2017, 329, 193–204 CrossRef CAS PubMed.
- J. Zou, H. Liu, J. Luo, Q. Xing, H. Du and X. Jiang,
et al., Three-dimensional reduced graphene oxide coupled with mn3o4 for highly efficient removal of Sb(III) and Sb(V) from water, ACS Appl. Mater. Interfaces, 2016, 8(28), 18140–18149 CrossRef CAS PubMed.
- J. Luo, C. Hu, X. Meng, J. Crittenden, J. Qu and P. Peng, Antimony removal from aqueous solution using novel α-MnO2 Nanofibers: Equilibrium, kinetic, and density functional theory studies, ACS Sustainable Chem. Eng., 2017, 5(3), 2255–2264 CrossRef CAS.
- A. J. Howarth, M. J. Katz, T. C. Wang, A. E. Platero-Prats, K. W. Chapman and J. T. Hupp,
et al., High Efficiency adsorption and removal of selenate and selenite from water using metal–organic frameworks, J. Am. Chem. Soc., 2015, 137(23), 7488–7494 CrossRef CAS PubMed.
- C. Wang, X. Liu, J. P. Chen and K. Li, Superior removal of arsenic from water with zirconium metal-organic framework UiO-66, Sci. Rep., 2015, 5(1), 16613 CrossRef CAS PubMed.
- K. A. Lin, S. Chen and A. P. Jochems, Zirconium-based metal organic frameworks: Highly selective adsorbents for removal of phosphate from water and urine, Mater. Chem. Phys., 2015, 160, 168–176 CrossRef CAS.
- T. L. Tan, P. A. P. Krusnamurthy, H. Nakajima and S. A. Rashid, Adsorptive, kinetics and regeneration studies of fluoride removal from water using zirconium-based metal organic frameworks, RSC Adv., 2020, 1(32), 1874–18752 Search PubMed.
Footnotes |
† Electronic supplementary information (ESI) available. See DOI: 10.1039/d1en00878a |
‡ Co-first author. |
|
This journal is © The Royal Society of Chemistry 2022 |
Click here to see how this site uses Cookies. View our privacy policy here.