Adsorption of antibiotics onto graphene oxide imparts their antagonistic effects on Synechocystis sp.: model development and proteomic analysis†
Received
18th August 2021
, Accepted 18th November 2021
First published on 19th November 2021
Abstract
The interactions between graphene oxide (GO) and antibiotics play an important role in determining their combined toxicity. However, the related information is lacking, especially for aquatic organisms. Here, we found that the combined toxicity of GO and antibiotics to a cyanobacterium Synechocystis sp. PCC 6803 was dependent on their interplays. GO exhibited an additive effect with florfenicol (FLO) but an antagonistic effect with erythromycin (ETM), ofloxacin (OFL), or chlorotetracycline (CTC). Furthermore, the adsorption of antibiotics onto GO resulted in their antagonistic effects. An adsorption–isobolographic model to predict the combined effect of GO and antibiotics (γ) was developed by combining the adsorption isotherm and isobolographic method. The model was validated by the good agreement between the theoretical and experimental values of γ, and can also be used to predict the combined toxicity of carbon nanotubes and tetracycline. Proteomic analysis further revealed that the exposure to antibiotics upregulated the pathway of the ribosome but downregulated the pathway of oxidative phosphorylation, which were attenuated by the addition of GO in the co-exposure groups. Compared with the single exposure groups of antibiotics, the addition of GO downregulated the proteins related to perceiving and transmitting the signals of hyperosmotic stress, explaining the increased membrane permeability in the combined exposure groups. This study highlights the significance of pollutant adsorption onto GO in determining their combined toxicity, and calls for further studies on other types of engineered or naturally occurring carbon-based nanomaterials.
Environmental significance
Although graphene oxide (GO) shows excellent adsorption capacity for various chemicals, how adsorption influences the combined toxicity of GO and chemicals is poorly understood. This study investigated the single and combined toxicity of GO and 4 antibiotics to Synechocystis sp. The results revealed an additive effect between GO and florfenicol but an antagonistic effect between GO and erythromycin, ofloxacin, or chlorotetracycline. Moreover, the adsorption of antibiotics onto GO induced their antagonistic effects. A model to predict the antagonistic effects between GO and antibiotics was developed, which was validated by the experimental results. Additionally, the toxicity mechanisms of GO and/or antibiotics were revealed by proteomic analysis. This study highlights the importance of pollutant adsorption onto GO in evaluating their combined toxicity.
|
1. Introduction
The increasing worldwide contamination of freshwater with a manifold of chemicals threatens aquatic organisms and human health.1 Pollutants in the environment elicit complex ecological hazards to various organisms, such as algae, fish, plants, animals, and humans by transforming and accumulating in the food chain and material circulation system.2,3 This raises considerable toxicological concerns, particularly the unknown and unpredictable prolonged effects of complex mixtures. Moreover, the omnipresent coexistence of multiple pollutants in the environment, including organic pollutants, nanomaterials, heavy metals and so on, also brings great challenges to ecological risk assessment.4,5 Therefore, it is extremely critical to explore the combined toxicity of various contaminants in the aquatic environment.6,7
The combined toxicity of multiple contaminants is inscrutable and contentious due to the complexity of co-contaminated systems. For the same type of contaminant, synergistic effects were observed between antibiotics,8,9 metals,10 or metal-based nanoparticles.11 However, distinct effects of different couples of antibiotics on A. fusiformis were found.12 The joint toxicity of different types of pollutants is more complex. Compared with the single groups, co-exposure of tetracycline (TC) and titanium dioxide demonstrated stronger toxicity to Scenedesmus obliquus,13 while the mixture of microplastics and lead had less effect on the survival rate of Microcystis aeruginosa.14 On the other aspect, the combined toxicity of binary pollutants may be dose-dependent or organism-dependent. For example, TC and titanium dioxide showed an additive effect at a low concentration and an antagonistic effect at a high concentration on Scenedesmus obliquus,15 and it is antithetical to Chlorella sp.16 Despite these developments, how the interactions of different types of pollutants influence their combined toxicity is still in its infancy.
As typical emerging contaminants, the toxicity of graphene oxide (GO) or antibiotics to algae has been profoundly investigated due to their wide occurrence in aquatic environments.17–20 Previous studies have shown that the toxicity mechanisms of GO to algae mainly include oxidative stress, shading effect, and physical damage.21–23 Antibiotics can affect algae growth by interfering with photosynthesis, affecting protein synthesis, destroying cell membranes or disrupting metabolic processes, etc.20,24 However, research on the combined toxicity of graphene and antibiotics to algae is scarce. It was reported that carbon nanotubes (CNTs) exhibited an antagonistic effect with TC due to the strong adsorption of TC onto CNTs.24 Regarding the excellent adsorption capacity of GO for various pollutants, it can be speculated that GO can influence the toxicity of antibiotics to aquatic organisms.
Therefore, in this study, Synechocystis sp. PCC 6803, a cyanobacterium, was selected as a model organism. The combined effects of GO and 4 antibiotics, including florfenicol (FLO), erythromycin (ETM), ofloxacin (OFL), and chlorotetracycline (CTC), on cell survival of Synechocystis sp. were investigated. These 4 antibiotics belong to 4 different classes of antibiotics, chloramphenicol, macrolides, quinolones, and tetracycline, respectively, and were widely detected in surface water.18 The toxic mechanisms were revealed by reactive oxygen species (ROS) quenching experiment, malondialdehyde (MDA) analysis, cell membrane permeability, and proteomic analysis. Particularly, a model describing and predicting how the adsorption of pollutants by GO influences their combined toxicity was developed by combining the adsorption isotherm and isobolographic method. This study provides new insights into the mechanisms of combined toxicity between carbon-based nanomaterials and antibiotics.
2. Materials and methods
Chemicals
Commercially available GO was obtained from Chengdu Organic Chemistry Co. Ltd., Chinese Academy of Sciences (China). FLO (>98%), ETM (>98%), OFL (>98%), and CTC (>98%) were obtained from Sigma-Aldrich (USA). The cyanobacterium Synechocystis sp. strain PCC 6803 was purchased from Fresh Algae Culture Collection at the Institute of Hydrobiology (China). Methanol and formic acid were of HPLC grade, and purchased from Thermo Fisher Scientific (USA). 2′,7′-Dichlorodihydrofluorescein diacetate (DCFH-DA) and propidium iodine (PI) were purchased from AAT Bioquest, Inc. (USA).
Characterization of GO
The microstructure of GO was observed by field-emission high resolution transmission electron microscopy (TEM) (JEM-2100F, JEOL, Japan). Thermal gravimetric analysis (TGA) of GO was conducted using a thermogravimetric analyzer Q50 (TA Instruments, New Castle, DE, USA). The Fourier transform infrared (FTIR) spectrum of GO was recorded using an FTIR spectrometer (Bruker Tensor27, Germany). The specific surface area of GO was measured using an accelerated surface area & porosimetry system (ASAP 2020 plus, Micrometer, USA).
Acute toxicity test
All 96 h acute toxicity tests on Synechocystis sp. were conducted in triplicate according to the OECD guideline.25 The composition of the BG-11 medium (pH ≈ 8) is listed in Table S1.† The test endpoint was mortality. All the toxicity tests were conducted using a light oscillation incubator (ZQZY-BGF8, Shanghai Zhichu instrument Co., Ltd., China). Algal cells with an initial cell density of 1 × 106 cells per mL were cultured at 28 ± 1 °C with continuous shaking (135 rpm) and alternating illumination (4000–6000 lux, light
:
dark of 14
:
10 h). After 96 h of exposure, direct microscopic counting of algal cell was performed using a counting chamber (Qiujing, XB-K-25, China) and an optical microscope (BX51, Olympus, Japan). The content of chlorophyll-a was determined according to the method described in our previous study.24
The GO and antibiotic stock solutions were prepared in the BG11 medium. In the exposure experiment of individual pollutants, antibiotics were added to a 250 mL flask according to the designed concentration. The GO stock solution (500 mg L−1) was sonicated (30 min) using an ultrasonic cell disruption system (Scientz Biotechnology, China, 25 kHz) for complete dispersion. An appropriate amount of GO stock solution was added into the flask (250 mL) with the BG-11 medium, followed by sonication for 10 minutes to achieve full dispersion. In the combined exposure experiment, the designed volume of antibiotic stock solution was added into the pre-dispersed GO solution and then mixed in the dark for 2 h to reach equilibrium. The concentration of GO was constant at sublethal concentrations of 5 mg L−1, 10 mg L−1, or 20 mg L−1 with various concentrations of antibiotics. Finally, 10 mL of algae prepared in advance were inoculated into each flask, followed by 96 h incubation.
Analysis of ROS, MDA, and membrane permeability
After 96 h exposure, 5 mL of the algae suspension was incubated with 50 μL of DCFH-DA (1 mmol L−1 in dimethyl sulfoxide) in the dark at 25 °C for 30 min. The supernatant was used for fluorescence detection after ultrasonic crushing (25 kHz, 5 min) and centrifugation (3600g, 10 min). The fluorescence intensity was measured using a fluorescence spectrophotometer (LS55, PerkinElmer, USA) with excitation and emission wavelengths of 485 nm and 530 nm, respectively. The measurement of the MDA content was conducted according to the method described in a previous study,26 and specific information is shown in Text S1.† The membrane permeability of Synechocystis sp. cells after 96 h exposure was observed by laser confocal scanning microscopy (LCSM) with probe PI,27 as described in our previous study.24
ROS quenching experiment
The ROS quenching experiment was performed to explore the role of ROS in the mortality of Synechocystis sp. Specifically, the ROS quencher thiourea solution (100 mg L−1) was prepared in the sterilized BG11 medium.28 The concentrations of antibiotics were approximately equal to their EC50 values: 0.05 mg L−1 for ETM, 0.3 mg L−1 for OFL, 1.2 mg L−1 for FLO and 10 mg L−1 for CTC. The non-lethal concentration of GO (10 mg L−1) was selected. In total, ten exposure groups were selected for ROS quenching experiments (Table S2†). The mixture of GO and antibiotics was incubated in the dark at 28 ± 1 °C for 2 h to reach equilibrium. Then, 5 mL of thiourea solution was added, followed by inoculation. The ROS content in each group was quantified after 96 h exposure.
Adsorption experiments
The adsorption of 4 antibiotics (FLO, ETM, OFL, and CTC) on GO was investigated at GO concentration of 5, 10, and 20 mg L−1, respectively. All the batch experiments were conducted for 24 h at 28 ± 1 °C in a light oscillation incubator (ZQZY-BGF8, Shanghai Zhichu instrument Co., Ltd., China) with continuous shaking (135 rpm) and alternating illumination (4000–6000 lux, light
:
dark of 14
:
10 h). Then, the suspension was filtered through a glass fiber membrane (GF/F 0.7 μm, Whatman, UK). The method for antibiotic qualification is given in Text S2.† To evaluate the adsorption effect of antibiotics on the stability of GO, sedimentation experiments were conducted using the suspension of GO only (10 mg L−1), GO + FLO (10 mg L−1 of GO and 1.2 mg L−1 of FLO), GO + ETM (10 mg L−1 of GO and 0.05 mg L−1 of ETM), GO + OFL (10 mg L−1 of GO and 0.3 mg L−1 of OFL), and GO + CTC (10 mg L−1 of GO and 10 mg L−1 of CTC) after adsorption equilibrium. The absorbance of the suspension at 300 nm was measured in a quartz cuvette using a UV-visible spectrophotometer (UV-1800, Shimadzu, Japan).
Proteomic analysis
Ten experimental groups were selected for proteomic analysis (Table S2†), including control samples (CTR), 10 mg L−1 of GO (GO), 0.05 mg L−1 of ETM (ETM), 0.3 mg L−1 of OFL (OFL), 1.2 mg L−1 of FLO (FLO), 10 mg L−1 of CTC (CTC), and 10 mg L−1 of GO with 0.05 mg L−1 of ETM (GO_ETM), 0.3 mg L−1 of OFL (GO_OFL), 1.2 mg L−1 of FLO (GO_FLO), or 10 mg L−1 of CTC (GO_CTC). After 96 h exposure, 100 mL of the algae suspension was centrifuged (3600g, 15 min) to collect algae cells. Algal cells were collected by centrifugation of 100 mL suspension at 3600g for 15 min, washed three times with phosphate-buffered saline (0.01 mol L−1, pH = 7.0), and quenched in liquid nitrogen. The protein was extracted and digested immediately (Text S3†). A UPLC system (EASY-nLC 1000) was used to separate peptides. Further analysis was performed by UPLC-tandem mass spectrometry (Q Exactive™ Plus, Thermo) coupled with a nano-electrospray ion source.
The differentially expressed proteins (DEPs) (fold change >1.5 or <0.67) were annotated according to the Kyoto Encyclopedia of Genes and Genomes (KEGG), gene ontology, subcellular localization, and domain annotation, respectively. Then functional enrichment was performed based on the KEGG annotation. The protein–protein interaction (PPI) analysis was conducted online (http://string-db.org/cgi/input.pl). More detailed information on sample preparation and proteomic analysis is described in Text S3.†
Data analysis
The statistical significance among treatments (p < 0.05) was assessed using one-way ANOVA (SPSS PASW Statistics v18.0). The dose response curve was used to calculate the median effect concentration (EC50).29 The joint toxicity of GO and antibiotics was evaluated by using the modified isobolographic method:24 |  | (1) |
where A and B are the EC50 of GO and antibiotics (mg L−1), respectively. a (mg L−1) and b (mg L−1) are the concentrations of GO and antibiotics in the co-exposure test, respectively, when the combined toxicity achieves EC50. The combined effects were classified into synergistic (γ < 1), additive (γ = 1), and antagonistic (γ > 1). The larger the value of γ, the stronger the antagonistic effect.3Eqn (1) can be derived from Fig. S1.†24
The adsorption data were fitted using Freundlich or linear isotherms.30
Freundlich model:
Linear model:
where
Qe is the equilibrium amount of antibiotics adsorbed by GO (mg g
−1),
Ce is the equilibrium concentration of antibiotics (mg L
−1),
KF (mg g
−1 (mg L
−1)
−1/n) and 1/
n are the distribution coefficient and empirical constant of the Freundlich model, and
Kd (L g
−1) is the distribution coefficient of the linear adsorption isotherm.
3. Results and discussion
Characterization of GO
The sheet structure of GO is clearly displayed by TEM (Fig. S2a and b†). The peaks at 1050, 1618, and 1720 cm−1 in the FTIR spectra were assigned to C–O, C
C, and C
O (Fig. S2c†).31,32 The BET surface area of GO was 6.1 m2 g−1. The relatively low BET surface area was due to the aggregation of GO layers.33 As shown in the TGA curve of GO (Fig. S2e and f†), a weight loss over the temperature range of 20–100 °C indicates the loss of water. The weight loss from 100 °C to 300 °C was attributed to water evaporation and removal of unstable oxygen functional groups.34 A great weight loss over the temperature range of 500–550 °C corresponded to the weight loss of GO at 535 °C.32
Individual and combined toxicity of GO and antibiotics
The EC50 of GO to Synechocystis sp. was 102 mg L−1 (Fig. S3a and Table S4†), which is comparable to the EC50 of reduced graphene oxide (34 mg L−1), GO (37 mg L−1), and multi-layer graphene (62 mg L−1) to Chlorella pyrenoidosa.22 The EC50 of antibiotics to Synechocystis sp. (Fig. 1a–d and Table S4†) followed the order of ETM (0.06 mg L−1) < OFL (0.39 mg L−1) < FLO (1.56 mg L−1) < CTC (11.2 mg L−1). These values are in the range of those obtained in the previous studies (Fig. S4†), 0.086–5.75 mg L−1 for ETM,8,35–37 5–227 mg L−1 for FLO, 4.77 mg L−1 for OFL,38,39 and 1.19–35.2 mg L−1 for CTC12,40 to various algae.
 |
| Fig. 1 Effects of GO and FLO (a), ETM (b), OFL (c), or CTC (d) on the survival rate of Synechocystis sp. (GO0, GO5, GO10, and GO20 represent the GO concentration of 0, 5, 10, and 20 mg L−1, respectively). | |
In the combined exposure groups, the addition of GO evidently enhanced the toxicity of FLO but alleviated the toxicity of ETM, OFL, and CTC (Fig. 1a–d and Table S4†). To be specific, the survival rate was only 26.6% when exposed to 0.1 mg L−1 of ETM alone, and increased to 58.0% when exposed to 0.1 mg L−1 of ETM and 10 mg L−1 of GO (Fig. 1b). Moreover, the combined toxicity of GO and ETM, OFL, or CTC decreased with increasing concentration of GO (Fig. 1b–d). Martín-de-Lucía et al. also found that the binary exposure of GO and wastewater had a weaker effect on Chlamydomonas reinhardtii than when exposed alone.41
The addition of GO led to a higher ROS content than FLO alone, which was more obvious at higher concentration of GO (Fig. S5e†). This accounts for the enhanced toxicity of FLO by GO (Fig. 1a). For ETM, OFL, or CTC, when the concentration of antibiotics is relatively low, the presence of GO led to an increase in the production of ROS (Fig. S5f–h†). However, at higher antibiotic concentrations, the presence of GO decreased the ROS levels due to the adsorption of antibiotics by GO (Fig. S5f–h†). This coincides with the antagonistic effect between GO and ETM, OFL, or CTC (Fig. 1b–d). The variation of MDA is consistent with those of ROS levels (Fig. S5i–l†), reflecting the lipid peroxidation caused by ROS.42
ROS quenching experiments (Fig. 2a and b) showed that the addition of thiourea significantly quenched the ROS in different exposure groups (p < 0.05). However, the survival rate did not increase significantly after addition of thiourea in all the exposure groups (p > 0.05, Fig. 2c and d) except for FLO. This indicates that ROS is not the major toxic mechanism for ETM, OFL, and CTC, but maybe one of the toxic mechanisms for FLO. The exposure to FLO causing oxidative stress and cell membrane damage has also been found by Zhang et al.,43 while You et al. found that ROS did not contribute to the mortality of Synechocystis sp. induced by chloramphenicol and TC.24 The role of ROS in the toxicity of antibiotics to algae is related to the type of antibiotic, which needs further investigation.
 |
| Fig. 2 ROS content (a and b) and survival rate (c and d) without and with addition of thiourea (THU) (*p < 0.05 and **p < 0.01 suggest significant differences between groups with and without thiourea, one-way ANOVA), and the proportion of cells with a permeable membrane (e) for different exposure groups (CTR: control sample, GO: 10 mg L−1 of GO, ETM: 0.05 mg L−1 of ETM, OFL: 0.3 mg L−1 of OFL, FLO: 1.2 mg L−1 of FLO, CTC: 10 mg L−1 of CTC, GO_ETM: 10 mg L−1 of GO with 0.05 mg L−1 of ETM, GO_OFL: 10 mg L−1 of GO with 0.3 mg L−1 of OFL, GO_FLO: 10 mg L−1 of GO with 1.2 mg L−1 of FLO, GO_CTC: 10 mg L−1 of GO with 10 mg L−1 of CTC). | |
The LCSM images of Synechocystis sp. cells showed that the membrane integrity was disturbed in all exposure groups (except for CTR) (Fig. S6†). The proportions of cells suffering from the disturbance of the membrane integrity were 59.5% for FLO, 71.4% for ETM, 43.8% for OFL, and 59.2% for CTC (Table S5† and Fig. 2e). ETM exhibited the highest membrane permeability among the 4 antibiotics, even though its mortality (survival rate 63.7%) was the lowest in the single exposure groups of antibiotics. The exposure to 10 mg L−1 GO alone caused a high proportion of cell membrane permeability (67.7%, Fig. 2e), although the mortality induced by GO was the lowest (survival rate 97.2%) among all the exposure groups (Table S5† and Fig. 2e). In the co-exposure groups of GO and antibiotics, the percentage of cells with a permeable membrane caused by GO_FLO, GO_ETM, GO_OFL, and GO_CTC was 79.4%, 81.3%, 49.3%, and 100% (Table S5† and Fig. 2e), respectively. Compared with the corresponding single exposure group of antibiotics, the addition of GO enhanced the permeability of the cell membrane in the co-exposure groups. This is possibly due to the fact that GO can act as “nano leaves”, causing direct mechanical damage to algal cell membrane.22,23
Adsorption–isobolographic model development for the combined toxicity
According to the modified isobolographic method (Fig. 3a–d), GO exhibited an additive effect with FLO (γ = 0.92–0.95) but an antagonistic effect with ETM (γ = 2.10–2.27), OFL (γ = 2.25–4.90), or CTC (γ = 1.42–2.30) on Synechocystis sp. Moreover, the antagonistic effects between GO and ETM/OFL/CTC increased with increasing GO concentration. This can be interpreted by the adsorption of antibiotics onto GO.24 Indeed, the adsorption of FLO onto GO was weak (Fig. 3e), so an additive effect was observed between them. Similarly, carbon nanotubes showed an additive effect with chloramphenicol (CAP) because of the absence of interactions between them.24 In contrast, the strong adsorption of ETM, OFL, and CTC onto GO (Fig. 3f–h) resulted in their antagonistic effects on Synechocystis sp. However, as shown in Fig. S8,† the adsorption of ETM, OFL and CTC onto GO alleviated the sedimentation of GO significantly compared with GO only. This means that GO was more stable in the presence of ETM, OFL and CTC, and thus may induce stronger toxicity to Synechocystis sp., which may decrease the antagonistic effects between GO and ETM/OFL/CTC to some extent. In contrast, the sedimentation of GO did not change significantly in the presence of FLO due to its insignificant adsorption onto GO.
 |
| Fig. 3 The combined toxicity of GO with FLO (a), ETM (b), OFL (c), or CTC (d) and adsorption isotherms of FLO (e), ETM (f), OFL (g), and CTC (h) onto GO (adsorption curve is the Freundlich model fit; GO0, GO5, GO10, and GO20 represent the GO concentration of 0, 5, 10, and 20 mg L−1, respectively). | |
The adsorption of antibiotics by GO led to their antagonistic toxic effects. We assumed that the adsorption was the major determinant to the antagonistic effect, and the antibiotics adsorbed by CNTs or GO are not bioavailable. Therefore, the effective concentration of antibiotics in the presence of GO is the equilibrium concentration of antibiotics after adsorption (Ce). When the adsorption of antibiotics by GO is negligible (Fig. 3a), an additive toxicity effect between antibiotics and GO was observed. Therefore, the combined toxicity of effective antibiotics (Ce) and GO is additive (γ = 1), which is expressed as:
|  | (4) |
The effective concentration of antibiotics (Ce) is then expressed as:
|  | (5) |
The relationship of C0 and Ce is expressed as:
|  | (6) |
where
m is the content of the adsorbent (g L
−1), which is the concentration of GO (
a, mg L
−1) in this study. Then,
C0 can be expressed as:
If the adsorption of antibiotics onto GO is fitted to the Freundlich isotherm (eqn (2)), the parameter Qe is substituted into eqn (7):
| C0 = 0.001aKFCe1/n + Ce | (8) |
where
Ce can be substituted by
eqn (5):
|  | (9) |
In eqn (1), the parameter, b, is the amount of antibiotics added in the combined exposure tests, that is, the initial amount of antibiotics (C0). Therefore, eqn (1) can be transformed to eqn (10):
|  | (10) |
Substituting the parameter C0 into eqn (10), the adsorption–isobolographic model can be obtained:
|  | (11) |
If the adsorption of antibiotics onto GO is fitted to the linear isotherm (eqn (3)), KF = KD and 1/n = 1. Then, eqn (11) is simplified to:
| 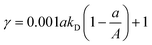 | (12) |
The adsorption–isobolographic model, eqn (11) and (12), can be used to calculate the value of γ, which reflects the combined toxicity of GO and antibiotics. In this study, the value of parameter A (EC50 of GO) was 102 mg L−1, and then eqn (11) is plotted in Fig. 4a. As shown in Fig. 4a, the value of γ first increased, reached the maximum, and then decreased with increasing values of a. The maximum value of γ declined with increasing 1/n at relatively lower B, and it is the opposite at relatively higher B. Moreover, γ always increased with increasing KF. On the other hand, the value of a, at which the value of γ reached the maximum, decreased with increasing 1/n. If the adsorption of antibiotics onto GO is fitted to the linear isotherm, the value of γ can be calculated according to eqn (12). As plotted in Fig. 4b, the value of γ first increased, reached the maximum, and then decreased with increasing values of a. Moreover, same as Fig. 4a, γ still increased with increasing Kd. However, different from Fig. 4a, the value of a corresponding to the maximum value of γ was constant and equal to 0.5A (0.5EC50 of GO).
 |
| Fig. 4 Plots of the theoretical values of γ calculated by using eqn (11) (a) and (12) (b) and comparison of the theoretical and experimental values of γ (c). | |
In this study, the adsorption isotherms of FLO, ETM, OFL, and CTC are fitted to the Freundlich isotherm (Fig. 3e–h). According to the fitting parameters given in Table S6,† the theoretical values of γ were calculated according to eqn (11), which are also given in Table S6.† In addition, our previous study also showed that the adsorption of TC onto CNTs also resulted in their antagonistic toxicity to Synechocystis sp.24 The adsorption data of TC onto CNTs were also used to predict the γ values of their combined toxicity (Table S7†). As shown in Fig. 4c, the theoretical values of γ agreed well with the experimental ones with R2 = 0.917, indicating the good performance of the model. The results showed that eqn (11) and (12) can be used to predict the value of γ, which reflects the degree of the antagonistic effect between GO and antibiotics. That is, if the adsorption behaviors of antibiotics onto GO were known, the antagonistic effects between GO and various antibiotics (γ value) can be predicted and compared using eqn (11) or (12).
Moreover, the good agreement between the theoretical and experimental γ for the combined toxicity of TC and CNTs in Fig. 4c also revealed that the model may be extrapolated to other types of carbon-based nanomaterials and organic chemicals. This also verified the applicability of eqn (11) and (12) to predict γ. However, it should be noted that there are two assumptions for the development of the adsorption–isobolographic model: (1) adsorption is the predominant factor inducing the antagonistic effect; (2) the antibiotics adsorbed by CNTs or GO are not bioavailable. In addition, the adsorption–isobolographic model may not be used for assessing the combined toxicity of carbon-based nanomaterials and organic chemicals to aquatic organisms that can take up nanomaterials through diet, because, in this case, the adsorbed pollutants may be bioavailable in the digestive tract of aquatic organisms.
Responses of proteins in Synechocystis sp.
Proteomic analysis showed that GO elicited the largest number of DEPs (1319) among all the single exposure groups of GO or antibiotics (531–888) (Fig. 5a–d and S7†), suggesting that the sublethal concentration of GO induced stronger disturbance of proteins in Synechocystis sp. than the lethal concentrations of antibiotics. Ouyang et al.44 found that GO induced hundreds of DEPs in Chlorella vulgaris. The co-exposure to GO and antibiotics triggered more DEPs than their corresponding single exposure groups of antibiotics (Fig. S7†), irrespective of their combined toxicity effect being additive or antagonistic. Moreover, the addition of GO decreased the number of upregulated DEPs but increased the number of downregulated DEPs compared with the corresponding single exposure groups of antibiotics (Fig. 5a–d and S7†). This indicates that more DEPs were downregulated with the addition of GO. In addition, the co-exposure to GO and FLO induced the largest number of DEPs (1782) (Fig. 5a), and more new DEPs different from their individual groups were observed in the exposure group of GO_FLO (333) compared with other co-exposure groups (53–168) (Fig. 5b–d). This coincides with the additive effect between GO and FLO.
 |
| Fig. 5 Venn plots (a–d) of DEPs in the single and combined groups of GO and antibiotics and radar charts (e–h) of the number of DEPs under different COG classifications in the single and combined groups of GO and antibiotics (GO: 10 mg L−1 of GO, ETM: 0.05 mg L−1 of ETM, OFL: 0.3 mg L−1 of OFL, FLO: 1.2 mg L−1 of FLO, CTC: 10 mg L−1 of CTC, GO_ETM: 10 mg L−1 of GO with 0.05 mg L−1 of ETM, GO_OFL: 10 mg L−1 of GO with 0.3 mg L−1 of OFL, GO_FLO: 10 mg L−1 of GO with 1.2 mg L−1 of FLO, GO_CTC: 10 mg L−1 of GO with 10 mg L−1 of CTC. The category description represented by the capital letter A–Z is given in Table S8†). | |
The radar chart showed that the number of DEPs in the co-exposure group of GO and FLO was higher than the exposure groups of GO or FLO alone (Fig. 5e), revealing an additive effect between GO and FLO (Fig. 3a). However, the numbers of DEPs in the co-exposure groups of GO and other three antibiotics (ETM, OFL and CTC) were similar and even lower than those in the single exposure group of GO (Fig. 5f–h), indicating the antagonistic effect between GO and ETM, OFL, or CTC (Fig. 3a–d). Also as shown in Fig. 5e–h, most of the DEPs induced by GO or the mixture of GO and antibiotics were related to energy production and conversion (C), transport and metabolism of amino acid and nucleotides (E and F), and translation, ribosomal structure and biogenesis (J) (Table S8†). This implies that the energy production, DNA replication, and protein synthesis were severely disturbed in Synechocystis sp. This is consistent with the bactericidal mechanisms of antibiotics where FLO, ETM, and CTC inhibit protein synthesis by interfering with ribosomes and OFL interferes with bacterial DNA replication.45 In addition, the DEPs related to cell wall/membrane/envelope biogenesis (M) were also observed, indicating the interference with the membrane integrity of Synechocystis sp. (Fig. 2e).
The KEGG pathway enrichment of DEPs revealed that the ribosome pathway was significantly upregulated by the 4 antibiotics (Fig. 6). The ribosome is the cellular structure that carries out protein synthesis.46 The upregulation of the ribosome pathway is the positive response to cope with the inhibition of protein synthesis induced by antibiotics.45,47 However, in the combined exposure groups, only the co-exposure to FLO and GO still upregulated the ribosome pathway. The phenomenon is consistent with the combined toxicity between GO and antibiotics, in which GO exhibited an additive effect with FLO but an antagonistic effect with ETM, OFL, or CTC (Fig. 3a–d). Specifically, the 4 antibiotics caused the upregulation of a large number ribosomal proteins, while a number of proteins were downregulated by GO (Fig. 7a). Correspondingly, most of the proteins related to the ribosome in the co-exposure groups of GO and antibiotics were downregulated compared with the single exposure groups of antibiotics (Fig. 7a). This reveals the downregulation-effect of GO on proteins related to the ribosome. ETM also caused the upregulation of ribosomal proteins in Bacillus thuringiensis.48
 |
| Fig. 6 KEGG pathway enrichment of DEPs in different exposure groups compared with the control group (a) and fold changes (FC) of proteins related to perceiving and transmitting the signals of hyperosmotic stress (b) (GO: 10 mg L−1 of GO, ETM: 0.05 mg L−1 of ETM, OFL: 0.3 mg L−1 of OFL, FLO: 1.2 mg L−1 of FLO, CTC: 10 mg L−1 of CTC, GO_ETM: 10 mg L−1 of GO with 0.05 mg L−1 of ETM, GO_OFL: 10 mg L−1 of GO with 0.3 mg L−1 of OFL, GO_FLO: 10 mg L−1 of GO with 1.2 mg L−1 of FLO, GO_CTC: 10 mg L−1 of GO with 10 mg L−1 of CTC). | |
 |
| Fig. 7 Changes of proteins related to the pathways of ribosomes (a), oxidative phosphorylation (b), and ABC transport (c) in different exposure groups (GO: 10 mg L−1 of GO, ETM: 0.05 mg L−1 of ETM, OFL: 0.3 mg L−1 of OFL, FLO: 1.2 mg L−1 of FLO, CTC: 10 mg L−1 of CTC, GO_ETM: 10 mg L−1 of GO with 0.05 mg L−1 of ETM, GO_OFL: 10 mg L−1 of GO with 0.3 mg L−1 of OFL, GO_FLO: 10 mg L−1 of GO with 1.2 mg L−1 of FLO, GO_CTC: 10 mg L−1 of GO with 10 mg L−1 of CT`C). | |
Oxidative phosphorylation, which produces ATP, is an important process of energy metabolism in cyanobacteria.49 As shown in Fig. 6, the pathway of oxidative phosphorylation was significantly downregulated by all 4 antibiotics, suggesting the hindrance of energy production by antibiotics. The disturbance of the photosynthetic apparatus and the mitochondrial function, which produce energy, by ETM was also observed for microalga Pseudokirchneriella subcapitata.50 Moreover, the dysregulation in the pathway of oxidative phosphorylation became insignificant in all the combined exposure groups, indicating alleviation of GO in the energy production process of Synechocystis sp. induced by antibiotics. As shown in the detailed pathway of oxidative phosphorylation (Fig. 7b), most of the proteins involved in the oxidative phosphorylation decreased in all the single and combined exposure groups.
The KEGG pathway enrichment of DEPs also showed that the pathway of ABC transporters was also interfered significantly by ETM, OFL, CTC, and GO_OFL (Fig. 6). The disorder of the ABC transport system is a typical response under environmental stress.51 For the ABC transport system, iron transport and carbon transport were downregulated by antibiotics (Fig. 7c), which was attenuated in the presence of GO except for the exposure group of GO and FLO. This agrees with their joint toxicity. The downregulation of iron transport was also elicited by TC and carbon nanotubes in Synechocystis sp.24 Differently, most of the proteins involved in the nitrate and phosphate transport were upregulated by the antibiotics but were downregulated in the co-exposure groups of GO and antibiotics (Fig. 7c).
Moreover, the change of the ABC transporter pathway will affect the ion distribution, change the permeability of the cell membrane, disturb the osmotic pressure and further cause cell membrane damage.52 A total of 24 proteins related to perceiving and transmitting the signals of hyperosmotic stress were identified in this study (Table S9†), and some of them were upregulated in the single exposure groups of antibiotics (Fig. 6b), indicating the occurrence of feedback on hyperosmotic stress due to the disturbance of membrane permeability.53 However, GO can interfere with the function of this feedback mechanism by downregulating the related proteins (Fig. 6b). Compared with single exposure groups of antibiotics, GO decreased the proteins related to perceiving and transmitting the signals of hyperosmotic stress in the combined exposure groups (Fig. 6b). This explains the increased membrane permeability in the combined exposure groups regardless of the change in the survival rate (Fig. 2e).
4. Conclusions
This study investigated the single and combined toxicity of GO and 4 antibiotics to Synechocystis sp. The results showed that GO exhibited an additive effect with FLO but an antagonistic effect with ETM, OFL, or CTC. The adsorption of ETM, OFL, and CTC onto GO induced the antagonistic effects between them. Compared with the single exposure groups of antibiotics, the addition of GO enhanced the permeability of the cell membrane in the co-exposure groups. An adsorption–isobolographic model describing the relationship between the antagonistic effect (γ) and the adsorption parameters was established. The theoretical values of γ calculated by the model agreed well with the experimental ones. Proteomic analysis revealed that the exposure to antibiotics upregulated the pathway of the ribosome but downregulated the pathway of oxidative phosphorylation. Moreover, the proteins involved in the ribosome pathway and the transport of nitrate and phosphate were upregulated by antibiotics, but were downregulated by the addition of GO in the co-exposure groups. Our findings underscore the importance of interactions between GO and antibiotics in evaluating their combined toxicity.
Conflicts of interest
There are no conflicts to declare.
Acknowledgements
This research was supported by the National Natural Science Foundation of China (Grant No. 51879001 and 51925901) and Qinghai Natural Science Foundation (Grant No. 2019-ZJ-923).
References
- E. S. Bernhardt, E. J. Rosi and M. O. Gessner, Synthetic chemicals as agents of global change, Front. Ecol. Environ., 2017, 15, 84–90 CrossRef.
- M. Adeel, X. Song, Y. Wang, D. Francis and Y. Yang, Environmental impact of estrogens on human, animal and plant life: a critical review, Environ. Int., 2017, 99, 107–119 CrossRef CAS PubMed.
- Y. Zhang, M. Liu, B. Peng, S. Jia, D. Koh, Y. Wang, H. S. Cheng, N. S. Tan, B. Warth, D. Chen and M. Fang, Impact of mixture effects between emerging organic contaminants on cytotoxicity: a systems biological understanding of synergism between tris (1, 3-dichloro-2-propyl) phosphate and triphenyl phosphate, Environ. Sci. Technol., 2020, 54, 10722–10734 CrossRef CAS PubMed.
- X. Q. You, N. Xu, X. Yang and W. L. Sun, Pollutants affect algae-bacteria interactions: a critical review, Environ. Pollut., 2021, 276, 116723 CrossRef CAS PubMed.
- R. Deng, D. Lin, L. Zhu, S. Majumdar, J. C. White, J. L. Gardea-Torresdey and B. Xing, Nanoparticle interactions with co-existing contaminants: joint toxicity, bioaccumulation and risk, Nanotoxicology, 2017, 11, 591–612 CrossRef CAS PubMed.
- O. M. Awoyemi, S. Subbiah, K. N. Thompson, A. Velazquez, A. Peace and G. D. Mayer, Trophic-level interactive effects of phosphorus availability on the toxicities of cadmium, arsenic, and their binary mixture in media-exposed Scenedesmus acutus and media and dietary-exposed Daphnia pulex, Environ. Sci. Technol., 2020, 54, 5651–5666 CrossRef CAS PubMed.
- J. Zhao, F. Ning, X. Cao, H. Yao, Z. Wang and B. Xing, Photo-transformation of graphene oxide in the presence of co-existing metal ions regulated its toxicity to freshwater algae, Water Res., 2020, 176, 115735 CrossRef CAS PubMed.
- G. Wang, Q. Zhang, J. Li, X. Chen, Q. Lang and S. Kuang, Combined effects of erythromycin and enrofloxacin on antioxidant enzymes and photosynthesis-related gene transcription in Chlorella vulgaris, Aquat. Toxicol., 2019, 212, 138–145 CrossRef CAS PubMed.
- Z. Ren, H. Xu, Y. Wang, Y. Li, S. Han and J. Ren, Combined toxicity characteristics and regulation of residual quinolone antibiotics in water environment, Chemosphere, 2021, 263, 128301 CrossRef CAS PubMed.
- R. C. Gebara, L. D. O. G. Alho, G. S. Rocha, A. da Silva Mansano and M. D. G. G. Melão, Zinc and aluminum mixtures have synergic effects to the algae Raphidocelis subcapitata at environmental concentrations, Chemosphere, 2020, 242, 125231 CrossRef CAS PubMed.
- Y. Liu, S. Wang, Z. Wang, N. Ye, H. Fang and D. Wang, TiO2, SiO2 and ZrO2 nanoparticles synergistically provoke cellular oxidative damage in freshwater microalgae, Nanomaterials, 2018, 8, 95 CrossRef PubMed.
- S. Carusso, A. B. Juárez, J. Moretton and A. Magdaleno, Effects of three veterinary antibiotics and their binary mixtures on two green alga species, Chemosphere, 2018, 194, 821–827 CrossRef CAS PubMed.
- B. Roy, P. K. Suresh, N. Chandrasekaran and A. Mukherjee, Antibiotic tetracycline enhanced the toxic potential of photo catalytically active P25 titanium dioxide nanoparticles towards freshwater algae Scenedesmus obliquus, Chemosphere, 2021, 267, 128923 CrossRef CAS PubMed.
- S. Wang, Q. Li, S. Huang, W. Zhao and Z. Zheng, Single and combined effects of microplastics and lead on the freshwater algae Microcystis aeruginosa, Ecotoxicol. Environ. Saf., 2021, 208, 111664 CrossRef CAS PubMed.
- V. Iswarya, V. Sharma, N. Chandrasekaran and A. Mukherjee, Impact of tetracycline on the toxic effects of titanium dioxide (TiO2) nanoparticles towards the freshwater algal species, Scenedesmus obliquus, Aquat. Toxicol., 2017, 193, 168–177 CrossRef CAS PubMed.
- V. Thiagarajan, L. Natarajan, R. Seenivasan, N. Chandrasekaran and A. Mukherjee, Tetracycline affects the toxicity of P25 n-TiO2 towards marine microalgae Chlorella sp, Environ. Res., 2019, 179, 108808 CrossRef CAS PubMed.
- Y. Zhao, Y. Liu, X. Zhang and W. Liao, Environmental transformation of graphene oxide in the aquatic environment, Chemosphere, 2021, 262, 127885 CrossRef CAS PubMed.
- S. Li, W. Shi, W. Liu, H. Li, W. Zhang, J. Hu, Y. Ke, W. L. Sun and J. Ni, A duodecennial national synthesis of antibiotics in China's major rivers and seas (2005–2016), Sci. Total Environ., 2018, 615, 906–917 CrossRef CAS PubMed.
- X. Hu and Q. Zhou, Health and ecosystem risks of graphene, Chem. Rev., 2013, 113, 3815–3835 CrossRef CAS PubMed.
- P. Välitalo, A. Kruglova, A. Mikola and R. Vahala, Toxicological impacts of antibiotics on aquatic micro-organisms: a mini-review, Int. J. Hyg. Environ. Health, 2017, 220, 558–569 CrossRef PubMed.
- X. Hu, S. Ouyang, L. Mu, J. An and Q. Zhou, Effects of graphene oxide and oxidized carbon nanotubes on the cellular division, microstructure, uptake, oxidative stress, and metabolic profiles, Environ. Sci. Technol., 2015, 49, 10825–10833 CrossRef CAS PubMed.
- J. Zhao, X. Cao, Z. Wang, Y. Dai and B. Xing, Mechanistic understanding toward the toxicity of graphene-family materials to freshwater algae, Water Res., 2017, 111, 18–27 CrossRef CAS PubMed.
- T. Malina, E. Maršálková, K. Holá, J. Tuček, M. Scheibe, R. Zbořil and B. Maršálek, Toxicity of graphene oxide against algae and cyanobacteria: Nanoblade-morphology-induced mechanical injury and self-protection mechanism, Carbon, 2019, 155, 386–396 CrossRef CAS.
- M. You, X. You, J. Hu, X. Yang and W. L. Sun, Carbon nanotubes influence the toxic effects of chloramphenicol and tetracycline on cyanobacterium Synechocystis sp. in different ways, Environ. Sci.: Nano, 2021, 8, 634–646 RSC.
-
Organization for Economic Co-operation and Development (OECD), Freshwater algae and cyanobacteria, growth inhibition test, Annex 5 of OECD guidelines for the testing of chemicals (OECD/OECDE 201), 2011.
- X. Q. You, X. Q. Cao, X. Zhang, J. H. Guo and W. L. Sun, Unraveling individual and combined toxicity of nano/microplastics and ciprofloxacin to Synechocystis sp. at the cellular and molecular levels, Environ. Int., 2021, 157, 106842 CrossRef CAS PubMed.
- M. L. Zhang, H. B. Wang, P. P. Liu, Y. X. Song, H. Huang, M. W. Shao, Y. Liu, H. Li and Z. H. Kang, Biotoxicity of degradable carbon dots towards microalgae Chlorella vulgaris, Environ. Sci.: Nano, 2019, 6, 3316–3323 RSC.
- I. Keren, Y. X. Wu, J. Inocencio, L. R. Mulcahy and M. K. Lewis, Killing by bactericidal antibiotics does not depend on reactive oxygen species, Science, 2013, 339, 1213–1216 CrossRef CAS PubMed.
- L. J. Feng, J. W. Li, E. G. Xu, X. D. Sun, F. P. Zhu, Z. J. Ding, H. Y. Tian, S. S. Dong, P. F. Xia and X. Z. Yuan, Short-term exposure to positively charged polystyrene nanoparticles causes oxidative stress and membrane destruction in cyanobacteria, Environ. Sci.: Nano, 2019, 6, 3072–3079 RSC.
- F. Wang, W. Sun, W. Pan and N. Xu, Adsorption of sulfamethoxazole and 17β-estradiol by carbon nanotubes/CoFe2O4 composites, Chem. Eng. J., 2015, 274, 17–29 CrossRef CAS.
- W. L. Sun, M. Li, W. Zhang, J. Wei, B. Chen and C. Wang, Sediments inhibit adsorption of 17β-estradiol and 17α-ethinylestradiol to carbon nanotubes and graphene oxide, Environ. Sci.: Nano, 2017, 4, 1900–1910 RSC.
- W. L. Sun, C. Wang, W. Pan, S. Li and B. Chen, Effects of natural minerals on the adsorption of 17β-estradiol and bisphenol A on graphene oxide and reduced graphene oxide, Environ. Sci.: Nano, 2017, 4, 1377–1388 RSC.
- H. Li, H. Ji, R. Zhang, W. Zhang, B. Pan, W. Liu and W. Sun, Hydrogen bonding rather than cation bridging promotes graphene oxide attachment to lipid membranes in the presence of heavy metals, Environ. Sci.: Nano, 2020, 7, 2240–2251 RSC.
- S. Park, J. An, J. R. Potts, A. Velamakanni, S. Murali and R. S. Ruoff, Hydrazine-reduction of graphite-and graphene oxide, Carbon, 2011, 49, 3019–3023 CrossRef CAS.
- M. González-Pleiter, S. Gonzalo, I. Rodea-Palomares, F. Leganés, R. Rosal, K. Boltes, E. Marco and F. Fernández-Piñas, Toxicity of five antibiotics and their mixtures towards photosynthetic aquatic organisms: implications for environmental risk assessment, Water Res., 2013, 47, 2050–2064 CrossRef PubMed.
- M. D. Machado and E. V. Soares, Sensitivity of freshwater and marine green algae to three compounds of emerging concern, J. Appl. Phycol., 2019, 31, 399–408 CrossRef CAS.
- M. Sendra, I. Moreno-Garrido, J. Blasco and C. V. Araújo, Effect of erythromycin and modulating effect of CeO2 NPs on the toxicity exerted by the antibiotic on the microalgae Chlamydomonas reinhardtii and Phaeodactylum tricornutum, Environ. Pollut., 2018, 242, 357–366 CrossRef CAS PubMed.
- A. Martins and L. Guilhermino, Influence of temperature on the toxicity of the veterinary antibiotic florfenicol to Chlorella vulgaris and Daphnia magna, Toxicol. Lett., 2015, 2, S131–S132 CrossRef.
- L. Fu, T. Huang, S. Wang, X. Wang, L. Su, C. Li and Y. Zhao, Toxicity of 13 different antibiotics towards freshwater green algae Pseudokirchneriella subcapitata and their modes of action, Chemosphere, 2017, 168, 217–222 CrossRef CAS PubMed.
- L. Lu, Y. Wu, H. Ding and W. Zhang, The combined and second exposure effect of copper (II) and chlortetracycline on fresh water algae, Chlorella pyrenoidosa and Microcystis aeruginosa, Environ. Toxicol. Pharmacol., 2015, 40, 140–148 CrossRef CAS PubMed.
- I. Martín-de-Lucía, M. C. Campos-Mañas, A. Agüera, F. Leganés, F. Fernández-Piñas and R. Rosal, Combined toxicity of graphene oxide and wastewater to the green alga Chlamydomonas reinhardtii, Environ. Sci.: Nano, 2018, 5, 1729–1744 RSC.
- D. Xu, X. Chen and B. Shao, Oxidative damage and cytotoxicity of perfluorooctane sulfonate on chlorella vulgaris, Bull. Environ. Contam. Toxicol., 2017, 98, 127–132 CrossRef CAS PubMed.
- Y. Zhang, X. Zhang, R. Guo, Q. Zhang, X. Cao, M. Suranjana and Y. Liu, Effects of florfenicol on growth, photosynthesis and antioxidant system of the non-target organism Isochrysis galbana, Comp. Biochem. Physiol., Part C: Toxicol. Pharmacol., 2020, 233, 108764 CAS.
- S. Ouyang, Q. Zhou, H. Zeng, Y. Wang and X. Hu, Natural nanocolloids mediate the phytotoxicity of graphene oxide, Environ. Sci. Technol., 2020, 54, 4865–4875 CrossRef CAS PubMed.
- P. Grenni, V. Ancona and A. Barra Caracciolo, Ecological effects of antibiotics on natural ecosystems: a review, Microchem. J., 2018, 136, 25–39 CrossRef CAS.
- P. Nissen, J. Hansen, N. Ban, P. B. Moore and T. A. Steitz, The structural basis of ribosome activity in peptide bond synthesis, Science, 2000, 289, 920–930 CrossRef CAS PubMed.
- X. Lin, L. Kang, H. Li and X. Peng, Fluctuation of multiple metabolic pathways is required for Escherichia coli in response to chlortetracycline stress, Mol. BioSyst., 2014, 10, 901–908 RSC.
- P. Zhou, Y. Chen, Q. Lu, H. Qin, H. Ou, B. He and J. Ye, Cellular metabolism network of Bacillus thuringiensis related to erythromycin stress and degradation, Ecotoxicol. Environ. Saf., 2018, 160, 328–341 CrossRef CAS PubMed.
- W. H. Nitschmann and G. A. Peschek, Oxidative phosphorylation and energy buffering in cyanobacteria, J. Bacteriol., 1986, 168, 1205–1211 CrossRef CAS PubMed.
- M. D. Machado and E. V. Soares, Impact of erythromycin on a non-target organism: Cellular effects on the freshwater microalga Pseudokirchneriella subcapitata, Aquat. Toxicol., 2019, 208, 179–186 CrossRef CAS PubMed.
- Y. Wang, B. Hu, S. Du, S. Gao, X. Chen and D. Chen, Proteomic analyses reveal the mechanism of Dunaliella salina Ds-26-16 gene enhancing salt tolerance in Escherichia coli, PLoS One, 2016, 11, e0153640 CrossRef PubMed.
- M. Yang and X. Wang, Interactions between Microcystis aeruginosa and coexisting bisphenol A at different phosphorus levels, Sci. Total Environ., 2019, 658, 439–448 CrossRef CAS PubMed.
- G. V. Novikova, I. E. Moshkov and D. A. Los, Protein sensors and transducers of cold and osmotic stress in cyanobacteria and plants, Mol. Biol., 2007, 41, 427–437 CrossRef CAS.
Footnote |
† Electronic supplementary information (ESI) available. See DOI: 10.1039/d1en00763g |
|
This journal is © The Royal Society of Chemistry 2022 |