Biodegradable polyurethane nerve guide conduits with different moduli influence axon regeneration in transected peripheral nerve injury†
Received
1st June 2021
, Accepted 3rd August 2021
First published on 5th August 2021
Abstract
Nerve guide conduits (NGCs) can replace autogenous nerve grafting in the treatment of peripheral nerve system (PNS) injury. However, the modulus of polyurethane NGCs that affects the outcome of PNS repair has been rarely elucidated in vivo. In this study, we developed biodegradable waterborne polyurethane (BWPU) NGCs with an outer BWPU membrane and an inner three-dimensional scaffold structure. The mechanical properties of BWPU NGCs can be modified by adjusting the molar content of polyethylene glycol (PEG) in the soft segments within the BWPU. Two types of BWPU NGCs with different moduli were prepared, containing 17% and 25% PEG in BWPU (termed as BWPU 17 NGCs and BWPU 25 NGCs, respectively). In rat sciatic nerves with 10-mm transected injury, mechanically stronger BWPU 17 NGCs exhibited superior nerve repair, which was similar to that obtained by the current gold standard autograft implantation, whereas weaker BWPU 25 NGCs displayed an unsatisfactory effect. Histological results revealed that both BWPU NGCs had anti-inflammatory effects and altered the activation state of macrophages to M2 phenotypes to enhance PNS regeneration. The analysis of growth-associated protein 43 expression, which regulates axon growth, revealed that the mechanical properties of BWPU NGCs influence the outcome of PNS regeneration by affecting the formation and extension of axons. These findings suggest that the mechanical properties of NGCs could play a key role in regulating PNS repair and should be considered in future biomaterial NGC designs.
1. Introduction
Peripheral nerve system (PNS) injury is a common disease worldwide.1,2 Annually, millions of patients suffer from this injury, resulting in motor and sensory disorders in the corresponding nerve distribution area.3 The development of methods to regenerate the PNS is urgently needed and represents a global social need. Fortunately, unlike the adult central nervous system, which is unable to spontaneously regenerate after injury,4 PNS has great potential to complete a self-repair and regeneration process.5 In clinical cases, small PNS defects can be repaired through spontaneous regeneration or by simply using fascicular neurorrhaphy.6 However, when a large injury (>10 mm) is encountered, the excessive tension and insufficient length of the residual nerve make it difficult to repair through neurorrhaphy alone.7 Moreover, misdirected axonal outgrowth, obstructive scar formation, and the resulting neuroma make it impossible to repair the nerve through spontaneous regeneration.8 Therefore, autologous nerve grafting is the gold standard for the repair of large PNS injury.9 In clinical practice, autologous nerve grafting is frequently limited by insufficient donor nerves and a mismatch between the injured and donor nerves.10 Therefore, nerve guide conduits (NGCs) were developed to replace donor nerves for PNS repair. NGCs can connect two stumps of the injured nerve, guiding axon regeneration from the proximal to the distal end of the nerve.
Nowadays, several natural and synthetic polymers have been tested to fabricate NGCs, and specific growth factors, modified cells, and physical stimuli have also been studied to construct diverse NGCs.11,12 NGCs based on collagen (NeuraGen), polyglycolic acid (Neurotube), and polylactide-ε-caprolactone (Neurolac) have obtained US Food and Drug Administration's approval for widespread production.13,14 These NGCs have shown good effects in PNS regeneration with functional recovery, indicating that artificial NGCs could be a robust solution for PNS repair. Polyurethane is a well-known FDA-approved soft and elastic polymeric biomaterial with high mechanical strength, which mimics the mechanical properties of soft and elastic tissues. Physicochemical properties can be finely adjusted to generate new functional polyurethanes. In the field of PNS regeneration, polyurethane-based biomaterials have also yielded series of achievements. It is reported that polyurethane NGCs can enhance rapid vascularization and promote the reconstruction of transected sciatic nerves, which makes them potential materials for NGC manufacturing.15,16
Stiffness is an intrinsic physical property of biomaterials, and plays a key role in regulating regeneration by influencing the cell adhesion, migration, and differentiation.17 The rigidity of the material–neuron interface significantly influences axon regeneration.18,19 Substrate stress relaxation on elastic substrates is also a key requirement for robust cell adhesion and migration.20 Matthew et al. reported that when cultured on a substratum with a Young's modulus of 907 kPa, human-induced pluripotent stem cell-derived neural stem cells showed extended longest neurites.21 It is reported that overextend PNS will cause severe damage to nerves, and moreover, mismatched moduli between biomaterials and host tissue will cause chronic inflammation.19 These studies have unquestionably indicated that the mechanical characteristic of NGCs is an important parameter that should be considered in the design of NGCs. However, the effects of the modulus of polyurethane NGCs on the outcome of PNS repair have, to the best of our knowledge, rarely been elucidated in animals. In vivo, the complex extracellular matrix (ECM) component and limb activity generate a more complicated ECM than that in cultured cells.22,23 Hence, in vivo experiments are needed to better understand how the modulus of NGCs influences PNS repair.
In this study, we designed biodegradable waterborne polyurethane (BWPU) NGCs with an outer BWPU film and an inner three-dimensional (3D) scaffold that simulates the nerve structure. The modulus of BWPU NGCs can be adjusted by changing the content of polyethylene glycol (PEG) in BWPU. We investigated the effect of BWPU NGCs with different moduli on nerve regeneration in a 10-mm-long transected sciatic nerve defect rat model. Additionally, we investigated the cell adhesion, ECM alteration, and axon regrowth induced by BWPU NGCs to reveal the underlying mechanisms through which BWPU NGCs with different moduli affect PNS regeneration.
2. Materials and methods
2.1. Preparation and characterization of BWPU NGCs
The BWPU emulsion was synthesized with poly ε-caprolactone, PEG, 1,3-propanediol, and L-lysine diisocyanate, which were prepared according to the methods of a previous study.24 The molecular weight of BWPU was measured by gel permeation chromatography (Breeze, USA). 1H NMR spectra were recorded using a Bruker AV II-400 MHz spectrometer (Germany) at 400 Hz (BBFO probe) and 25 °C with CDCl3, D2O and CD3OD as solvents and tetramethylsilane (TMS) as the internal standard. The molar contents of PEG in the soft segments of BWPU were 17% and 25% in the two synthesized BWPU NGCs. The outer lumen of the BWPU NGCs was molded using the freeze–drying method through a finger condenser pipe to form a cylinder appearance. Then, BWPU emulsion was added into the outer lumen, followed by freeze–drying again to form the inner 3D scaffold porous structure of BWPU NGCs. A contact angle analyzer equipped with a video recorder (DSA100, Kruss, Hamburg, Germany) was applied for the water contact angle (WCA) measurement with a droplet of 3 μL water. After measuring the volume of NGCs in the dry form, NGCs were soaked in PBS overnight to obtain the volume in the wet form, and then the swelling ratio was calculated as the ratio between the wet and dry forms. The compression modulus, bending modulus and tensile modulus measurements were performed using a universal tester (HZ-1004 Dongguan Lixian Instrument Scientific Co., Ltd). The inner NGC pore diameters were calculated by analyzing the scanning electron microscopy (SEM, Thermo scientific, USA) images using ImageJ software (USA). The porosity of the inner 3D scaffold was expressed according to the following equation:
where ρ*BWPU is the density of the scaffold and ρBWPU is the density of the film.
2.2. Surgical procedures
All animal experiments were approved by the ethics committee of Sichuan University. Forty adult female Sprague–Dawley rats (250–300 g) were divided into four groups (n = 10 per group) as follows: (1) BWPU 17 NGCs, (2) BWPU 25 NGCs, (3) autologous, and (4) control. All surgical devices were sterilized at 121 °C for 40 min before the operation. Animals were anesthetized with isoflurane. After disinfection, the skin was cut at the right posterior limb, and 10 mm of the sciatic nerve was excised after exposure. The autologous nerve and BWPU NGCs were anastomosed with the proximal and distal stumps using a 10-0 nylon suture. The muscle and skin were then closed using a 4-0 silk suture. Meloxicam was injected every 24 h until three days after surgery to alleviate pain. After the operation, the rats were freely housed at a temperature of 20–22 °C and relative humidity of 50–60% with standard laboratory chow and distilled water. Animals were euthanized 2 and 12 weeks after evaluating the functional score via CO2 asphyxiation (n = 4 for 2 weeks and n = 6 for 12 weeks).
2.3. Histological analysis of regenerated nerves
All samples were fixed in 4% paraformaldehyde for 24 h. The samples were cut into 10-μm-thick sagittal and transverse sections using a cryostat microtome (Leica, USA). Slides were permeabilized in 0.3% Triton X-100 (Sigma, USA) for 15 min, followed by blocking in immunostaining blocking buffer (B600060, Proteintech, USA) for 60 min at room temperature. The sections were then incubated with a primary antibody overnight at 4 °C. The following primary antibodies were used: anti-NF200 (Thermo Fisher, 13-1300), anti-S100 (Abcam, ab52642), and anti-SYP (Abcam, ab32594). The slides were then washed thrice, and incubated with the appropriate Alexa Fluor® 647- and Alexa Fluor® 488-conjugated secondary antibodies at 37 °C for 1 h, and the nuclei were stained with DAPI (0.5 g mL−1) for 10 min at room temperature. Images were acquired using a confocal laser-scanning microscope (Nikon A1R MP+).
2.4. Transmission electron microscopy analysis of regenerated nerves
The samples obtained at 12 weeks were fixed in 3% glutaraldehyde for 72 h, and then fixed with osmium tetroxide. After acetone dehydration, the samples were embedded in an EPIKOTE and cut into 50-nm-thick sections. A section of the nerves was examined under a transmission electron microscope (TEM, JEM-1400plus, Japan) at a voltage of 100 kV. The diameter of the myelinated nerve fibers and the thickness of the myelinated sheath were measured using ImageJ software (USA). The g-ratio was obtained by dividing the inner diameter by the outer diameter25 (n = 3 per group).
2.5. Histological analysis of axon regeneration and microenvironment
The samples were processed as described in Section 2.3. After transverse or sagittal cut, the sections were incubated with primary antibodies anti-68 (Abcam, ab201340), anti-CD86 (Invitrogen, PA5-88284), anti-mannose receptor (Abcam, ab52642), anti-growth-associated protein 43 (GAP 43; Abcam, ab12274), and anti-focal adhesion kinase (FAK; Abcam, ab81298). The sections were then incubated with secondary antibodies and DAPI, and images were captured using a confocal laser-scanning microscope (Nikon A1R MP +).
2.6. Western blot
Proteins were obtained from the samples using RIPA lysis buffer, and the total protein concentration was measured using a BCA kit (Thermo Fisher). Equivalent amounts of protein were separated using SDS-PAGE (180 V for 40 min). The protein was transferred to a PVDF membrane at 100 V for 1 h (Bio Rad). The membrane was blocked with milk for 1 h and then incubated with the corresponding primary antibodies listed above (in Section 2.4) overnight at 4 °C. The membrane was incubated with a secondary HRP-conjugated antibody (Abcam) for 1 h, and images were captured after incubation with an ECL luminous fluid (Millipore).
2.7. Functional analysis
The functional recovery of animals was evaluated by two experimenters from an experimental animal center who were not involved in animal surgery. The sciatic nerve index (SFI) was adopted to evaluate the functional recovery at 2 and 12 weeks post-surgery. The SFI was calculated using the following equation:
where PL is the distance from the third toe to the heel, TS is the distance between the first and the fifth toe, IT is the distance between the second and the fourth toe, E is the injured limb, and N is the contralateral limb. The SFI varies from 0 to 100, with lower numbers indicating worse limb function.26
2.8. Gastrocnemius muscle analysis
The gastrocnemius muscles obtained at 2 and 12 weeks in the injured and contralateral limbs were harvested and weighed. All samples were fixed in 4% paraformaldehyde for 24 h and embedded in paraffin, and 4 μm cross-sections of the muscles were obtained. Masson staining was conducted, and images were captured using a microscope (Olympus). The diameter of the muscle fibers and the proportion of fiber area were measured using ImageJ software (n = 4 per group).
2.9. Statistical analysis
Statistical evaluations were performed with one- or two-way ANOVA or repeated-measures ANOVA, as indicated in the figure legends. All statistical analyses were performed using GraphPad Prism software (GraphPad 8; GraphPad, Inc.). Statistical significance was set at p < 0.05.
3. Results
3.1. Morphology and mechanical characteristics of BWPU NGCs
We prepared BWPU emulsions with different contents of PEG (17% and 25%) to modulate the mechanical properties of the BWPU NGCs. The molecular weight data and 1H-NMR spectra of these BWPUs are shown in Fig. S1 (ESI†). These results verified that the BWPUs have been successfully synthesized. Structurally, the BWPU film on the outer surface of the NGCs acts as a conductor, and the inner tubular structure exhibits a porous structure that is similar to the tissue engineering scaffold applied in PNS regeneration. BWPU NGCs have a diameter of 5.43 mm, and the length of BWPU NGCs was cut to various sizes based on requirements (Fig. 1A). After coming into contact with water for 60 s, there was no significant difference between contact angles (Fig. 1B), indicating that different PEG levels did not significantly alter the hydrophilicity of BWPU NGCs. The swelling ratios of BWPU 17 NGCs and BWPU 25 NGCs were 122 ± 3% and 139 ± 2%, respectively, which could prevent excessive crush injury caused by NGCs when implanted in the lesion (Fig. 1B).
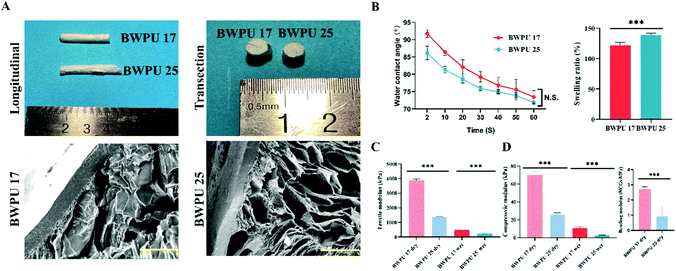 |
| Fig. 1 Characterization of BWPU NGCs. (A) Morphology of BWPU NGCs, and SEM images of transected BWPU NGCs (scale bar: 200 μm). (B) Hydrophilicity and swelling ratio of BWPU NGCs. (C) Tensile modulus of the BWPU films. (D) Compressive and bending modulus of BWPU NGCs. *p < 0.05, ***p < 0.01, N.S. p > 0.05, n = 3. | |
The different proportions of PEG successfully changed the mechanical properties of the BWPU NGCs to simulate the mechanical properties of autologous nerves.27 Because the outer surfaces of the BWPU NGCs were wrapped in the BPWU films, we applied the tensile modulus of the films to describe the mechanical properties of the BWPU NGCs. The tensile modulus of the BWPU 17 film was 3.890 ± 0.052 MPa in the dry state and 0.478 ± 0.030 MPa in the wet state, which were significantly higher than those of the BWPU25 film (1.384 ± 0.012 MPa in the dry state and 0.224 ± 0.004 MPa in the wet state) (Fig. 1C). The compression modulus of BWPU 17 NGCs was 69.8 ± 2.6 kPa (dry) and 10.8 ± 0.9 kPa (wet), which were significantly higher than those of BWPU 25 NGCs at 26.0 ± 1.0 kPa (dry) and 3.0 ± 0.1 kPa (wet) (Fig. 1D). In addition, the bending modulus of the dry BWPU 17 NGCs was 2.7 ± 0.2 MPa and that of the dry BWPU 25 NGCs was 0.9 ± 0.7 MPa (Fig. 1D).
The SEM results showed that BWPU NGCs have uniformly distributed pores wrapped in the BWPU film (Fig. 1A). The pore sizes of BWPU 17 NGCs and BWPU 25 NGCs were 102.4 ± 17.9 μm and 109.6 ± 15.8 μm, respectively. The density data of these BWPU films and scaffolds are shown in Fig. S1D (ESI†). The porosities of the BWPU 17 NGCs and BWPU 25 NGCs were 87.2 ± 2.3% and 89.6 ± 4.2%, respectively, which were close to the 80% optimal porosity for peripheral nerve regeneration.11,28
These results indicated that by modulating the content of PEG, we could successfully synthesize BWPU NGCs with an axial lumen, porous inner structure, and different mechanical properties for the application of peripheral nerve regeneration.
3.2. BWPU NGCs promote sciatic nerve regeneration
To evaluate the effect of BWPU NGCs on repairing sciatic nerves in vivo, BWPU 17 NGCs, BWPU 25 NGCs, or autologous nerves were implanted into a 10-mm gap defect of sciatic nerve injury, followed by successful anastomoses with proximal and distal stumps (Fig. S2, ESI†). The gross observation of BWPU 17 NGCs and autologous grafts showed obvious regenerated nerve tissues that bridged the two stumps and formed new nerves. BWPU 17 NGCs degraded completely at 12 weeks post-implantation. However, BWPU 25 NGCs, although degraded completely, exhibited incomplete regeneration of the sciatic nerve. At 12 weeks post-implantation, an apparent consecutive but incomplete regenerated sciatic nerve was found in the BWPU 25 NGC group (Fig. 2A). No obvious edema, adhesion, or infection was observed during the process. These results indicated that BWPU NGCs with different mechanical properties have different outcomes in sciatic nerve regeneration, and BWPU 17 NGCs are more capable of completely repairing the sciatic nerve.
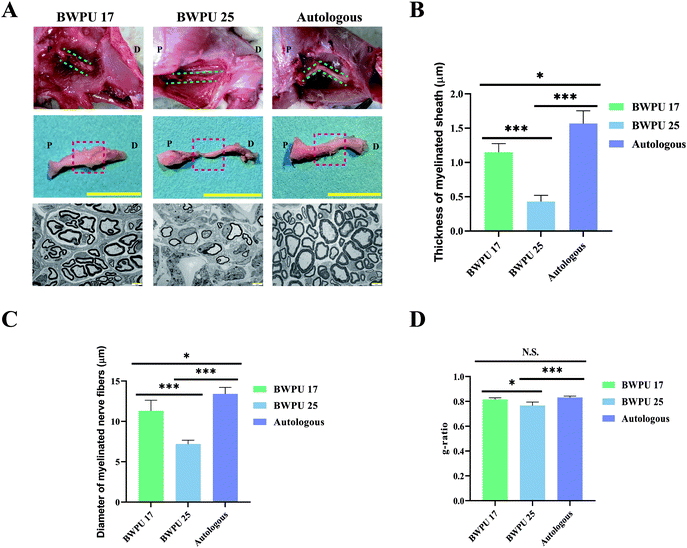 |
| Fig. 2 General observations and TEM of regenerated sciatic nerves at 12 weeks post-operation. (A) First line: sciatic nerve regeneration and BWPU NGC degradation after transplantation (green line: regenerated sciatic nerve). Second line: general observation of the sciatic nerve (red frame: sampling parts of TEM samples). Scale bar: 1 cm. P: proximal, D: distal. Third line: TEM of regenerated sciatic nerve. Scale bar: 5 μm. (B) Quantification analysis of the thickness of the myelinated sheath. (C) Quantification analysis of the diameter of the myelinated nerve fibers. (D) g-ratio *p < 0.05, ***p < 0.01, N.S. p > 0.05, n = 3. | |
We further studied the ultrastructure of the regenerated nerves. Midsections of the regenerated nerves were sampled to acquire TEM images. The results indicated that massive myelinated and unmyelinated nerve fibers were densely dispersed in the BWPU 17 NGC and autologous groups, in which the myelinated axon was surrounded by a clear, thick, and electron-dense myelin sheath. In contrast, in the BWPU 25 NGC group, few sparse and thin myelinated axons could be observed (Fig. 2A). Quantification statistics revealed that the BWPU 17 NGC and autologous groups had a significantly thicker myelin sheath than the BWPU 25 NGC group (p < 0.01, Fig. 2B). The BWPU 17 NGC and autologous groups also showed significantly larger myelinated nerve fibers than the BWPU 25 NGC group (p < 0.01, Fig. 2C). The analysis of the g-ratio, an optimal axonal myelination index, revealed that the BWPU 17 NGCs were not significantly different from the autologous group and were significantly better than the BWPU 25 NGCs in sciatic nerve regeneration (Fig. 2D). These results demonstrated that BWPU 17 NGCs have better capability than BWPU 25 NGCs for axon regeneration and myelination.
Immunofluorescence images were acquired to evaluate the axon regeneration induced by BWPU NGCs. We first analyzed the axon markers NF-200 and synaptophysin. In the BWPU 17 NGC group, continuous and high-density distributed NF-200 was observed, and regenerative axons showed a regular axial arrangement similar to that in the autologous group. The transverse images in the BWPU 17 NGC group also showed abundant compacts and regularly distributed axons. Synaptophysin, which represents axoplasmic transport,29 is highly correlated with nerve function and showed a morphology similar to that of NF-200 in the BWPU 17 NGC group. However, in the BWPU 25 NGC group, NF-200 and synaptophysin showed a curly arrangement in the proximal stump, and a tenuous regenerated nerve extended from the stump. At the distal end, evident shrunken nerve-bridged stumps with NF-200 surrounded them. The transverse images showed sparse and loosely arrayed axons and synaptophysin (Fig. 3A–C). S-100, which is closely tied to the functions of Schwann cells, formed the bands of Büngner for regenerating axons and secreting neurotrophic factors to promote axon regeneration, and the formation of the myelin sheath was also assessed.30 In the BWPU 17 NGC group, the distribution characteristics of S-100 were similar to those of NF-200 and synaptophysin, and abundant equally distributed S-100 was identified. The density of S-100 in the BWPU 17 NGC group was analogous to that in the autologous group. In the BWPU 25 NGC group, the expression and distribution of S-100 were sparse, as with NF-100 and synaptophysin. In the transverse images, significantly more S-100 can be observed in the BWPU 17 NGC group than in the BWPU 25 NGC group (Fig. 3D–F). These results further revealed that BWPU NGCs with different mechanical properties lead to significant differences in axon regeneration and remyelination in sciatic nerve repair.
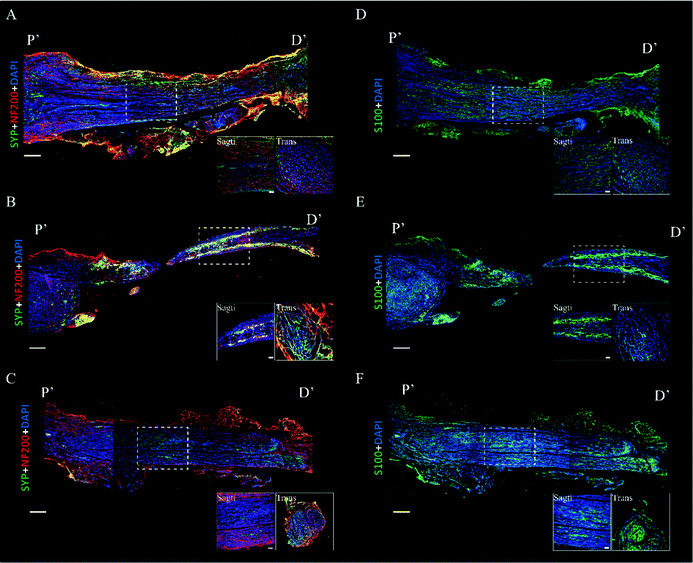 |
| Fig. 3 Histology evaluation of the regenerated sciatic nerve at 12 weeks post-operation. (A–C) Representative images of the regenerated sciatic nerve. Immunofluorescence staining of NF-200 and synaptophysin (SYP) indicates axon and axoplasmic transport. (D–F) Representative images of the regenerated sciatic nerve. S100 staining showed Schwann cells, indicating myelination. (A and D) In the BWPU 17 NGC group, complete sciatic nerve regeneration occurred. (B and E) The BWPU 25 NGC group showed incomplete sciatic nerve regeneration. (C and F) Autologous group. P′: proximal, D′: distal. Columns at the bottom right show magnified sagittal and transverse images marked with a white frame. Scale bar: 500 μm; scale bar (magnified): 100 μm. | |
3.3. BWPU NGCs enhanced the functional recovery of the sciatic nerve
We evaluated the SFI, which reflects the functional recovery of the sciatic nerve through analysis that targets the function of the distribution muscle. The sciatic nerve controls the infixion of the rat hind limbs and the movement of the feet. After injury, nerve dysfunction results in difficulties in plantar flexion of the ankles, impeding of ankle spread, and walking with a limp. Hence, by analyzing footprints, we estimated the recovery of the sciatic nerve (Fig. 4A). Our results indicated that two weeks after the operation, the SFI showed no difference among groups. At 12 weeks post-injury, a significantly improved SFI was observed in both the BWPU 17 NGC and autologous groups (p < 0.01), although the BWPU 25 NGC group also showed a significantly higher SFI than the control group (p < 0.05). However, the SFI of the BWPU 25 NGC group was remarkably lower than that of the BWPU 17 NGC and autologous groups (p < 0.01, Fig. 4B). The results of functional recovery indicated that different nerve regeneration and myelination resulting from varied NGCs are correlated with functional improvement, and BWPU 17 NGCs may lead to satisfactory sciatic nerve recovery as well as autologous groups.
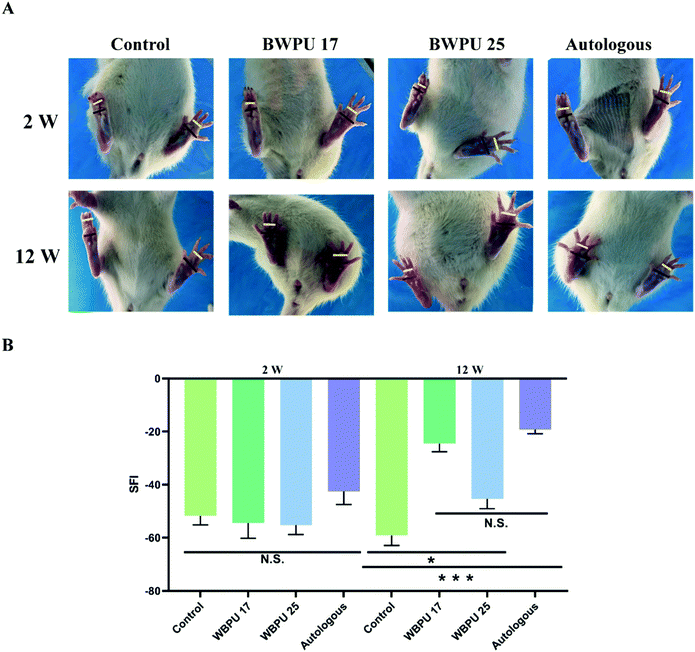 |
| Fig. 4 Functional recovery of the regenerated sciatic nerve at 12 weeks post-operation. (A) Footprint photographs of rats in corresponding groups. (B) Quantitative analysis of the sciatic function index (SFI) in rats. *p < 0.05, ***p < 0.01, N.S. p > 0.05, n = 4 for the 2-week analysis and n = 6 for the 12-week analysis. | |
3.4. Gastrocnemius muscle analysis
Denervated atrophy is a common complication of nerve injury, and axoplasmic transport is a crucial factor in maintaining the normal morphology and function of the target muscle.31 Hence, inspecting the anatomy and histological morphology of the gastrocnemius muscle is important to assess nerve regeneration (Fig. 5A). First, we analyzed the relative wet weight of the gastrocnemius muscle. The results showed that at 2 weeks post-injury, autologous groups exhibited better recovery than other groups (p < 0.01), and there was no significant difference among the other groups. At 12 weeks post-operation, the BWPU 17 NGC and autologous groups showed better recovery than the BWPU 25 NGC and control groups (p < 0.01), despite a better recovery for the BWPU 25 NGC group than for the control group (p < 0.05, Fig. 5C).
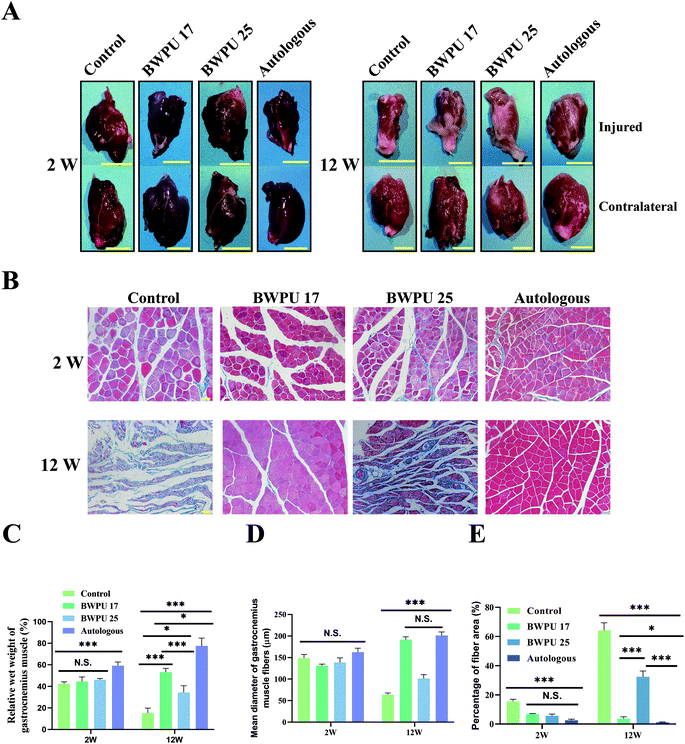 |
| Fig. 5 Gross observation and histological evaluation of gastrocnemius muscle at 2 and 12 weeks post-operation. (A) Macroscopic observation of gastrocnemius muscle exhibiting denervated atrophy of target gastrocnemius muscle. Scale bar: 1 cm. (B) Cross-sections with Masson trichrome staining of gastrocnemius muscles for the indicated groups. Scale bar: 200 μm. (C) Quantitative analysis of the gastrocnemius muscle weight. (D) Quantitative analysis of the diameter of gastrocnemius muscle fibers. (E) Quantitative analysis of the proportion of fiber area. *p < 0.05, ***p < 0.01, N.S. p > 0.05, n = 4. | |
Masson trichrome staining, which stains muscle in red and collagen fibers in blue, was used to reveal the histological morphology of the gastrocnemius muscle (Fig. 5B). Quantitative results indicated that at 2 weeks post-injury, no differences existed in the diameter of the gastrocnemius muscle fibers among all the groups, but at 12 weeks post-injury, significantly increased gastrocnemius muscle fibers appeared in the BWPU 17 NGC and autologous groups (p < 0.01), while BWPU 25 NGCs also showed larger gastrocnemius muscle fibers than the control group (p < 0.05, Fig. 5D). The proportion of the fiber area of the control group was the largest among the three groups at both time points. At 12 weeks post-injury, the fiber area proportion in the BWPU 25 NGCs was higher than that in the BWPU 17 NGC and autologous groups (p < 0.01), and the lowest proportion was found in the autologous group (Fig. 5E). These results suggested that BWPU NGCs with different moduli also influence gastrocnemius muscle reinnervation.
3.5. BWPU NGCs modulate the regeneration of the microenvironment
Based on the results of sciatic nerve regeneration and functional recovery, we investigated the mechanisms through which BWPU NGCs with different moduli influence sciatic nerve regeneration. Hence, we studied the activation state of the ECM microenvironment macrophages as well as their quantity and distribution in the proximal nerve stump. Based on the previous results of sciatic nerve regeneration, we suspected that nerve regeneration induced by BWPU NGCs could be enhanced at 2 weeks post-injury; therefore, we analyzed the samples from 2 weeks post-operation.32 The infiltration and distribution of macrophages did not differ between the BWPU 17 NGC and BWPU 25 NGC groups (Fig. 6A and B). Macrophages were observed at the NGC pores and the surrounding tissues, and numerous macrophages infiltrated NGCs. However, the number of macrophages was higher in the BWPU 17 NGC group than that in the BWPU 25 NGC group (p < 0.05) and was highest in the autologous group (p < 0.01) (Fig. 6C).
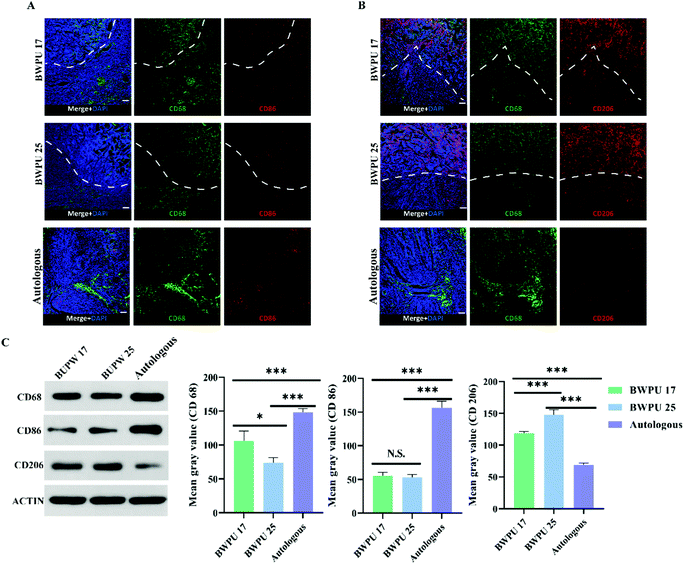 |
| Fig. 6 BWPU NGCs regulate the activation state of macrophages. Samples were obtained 2 weeks after surgery. (A and B) Infiltration of macrophages (CD68+) in lesions and pores of scaffolds (above the white line). More M1 macrophages were activated in the autologous group. Activated M2 macrophages were infiltrated into BWPU NGCs (above the white line), and more M2 macrophages were observed in the BWPU NGC group. (C) Western blot analysis of macrophage markers. *p < 0.05, ***p < 0.01, N.S. p > 0.05, n = 4. Scale bar: 100 μm. | |
Next, we investigated the activation state of macrophages by analyzing two phenotypes; CD86+ macrophages (M1, pro-inflammatory) and CD206+ macrophages (M2, anti-inflammatory), which presumably affect wound healing. A significant decrease in M1 macrophages was found in the BWPU 17 NGC and BWPU 25 NGC groups relative to the autologous group (p < 0.01, Fig. 6A and C). The M2 macrophage level was highest in the BWPU 25 NGC group (p < 0.01), and that in the BWPU 17 NGC group was also significantly higher than that in the autologous group (p < 0.01, Fig. 6B and C). The results of microenvironment histology showed that the implantation of BWPU NGCs with different moduli has anti-inflammatory effects and alters the activation state of macrophages to a pro-healing phenotype.
3.6. The BWPU NGC modulus influences cell adhesion and axon regeneration
To reveal the mechanisms behind the influence of the BWPU NGC modulus on sciatic nerve regeneration, we investigated the changes in cell adhesion and axon growth cone formation and elongation in the proximal nerve stump in each group, and samples were also obtained at 2 weeks post-operation. Cell adhesion was represented by the density and distribution of FAK. The BWPU 17 NGC and BWPU 25 NGC groups showed similar FAK expression, and both were significantly higher than that in the autologous group (p < 0.01, Fig. 7A). The distribution of cells marked by FAK was mostly located within the scaffold in BWPU 17 NGCs and homogeneously distributed in both nerves and scaffolds in BWPU 25 NGCs. Moreover, in BWPU 25 NGCs, FAK-positive cells seemed to form a noticeable curly arranged interface between the scaffold and the nerve.
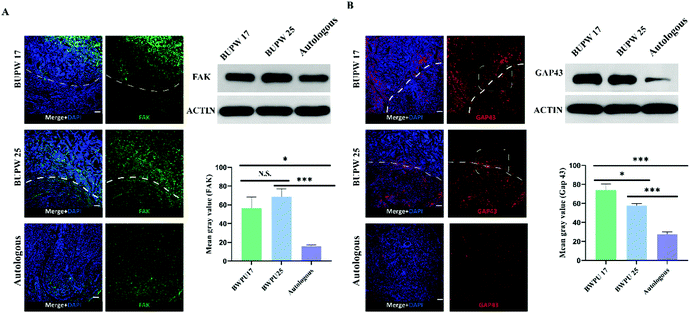 |
| Fig. 7 The BWPU NGC modulus regulates cell adhesion and axon regeneration. Samples were obtained 2 weeks after surgery. (A) Cell adhesion in scaffold pores (above the white line). More cells infiltrated BWPU 17 NGC and BWPU 25 NGC groups. (B) Formation and elongation of axons. The growth cone (GAP 43) showed the strongest expression in the BWPU 17 NGC group. In BWPU 17 NGCs, regenerated axons that grew through the interface of the nerve stump and BWPU NGCs (yellow frame) elongated and extended into the scaffold (above the white line). The contracted growth cone and axon were observed in BWPU 25 NGCs (yellow frame). *p < 0.05, ***p < 0.01, N.S. p > 0.05, n = 4, scale bar: 100 μm. | |
Furthermore, we investigated GAP 43, which represents the growth cone and is a key factor that mediates the formation and elongation of axons.33 A significantly increased GAP 43 expression was observed in BWPU 17 NGCs compared to that in BWPU 25 NGCs (p < 0.05) and GAP 43 density in both the BWPU NGC groups was higher than that in the autologous group (p < 0.01, Fig. 7B). Notably, we found that GAP 43 could grow across the interface of NGCs and nerves and extend to the deep part of the scaffold in the BWPU 17 NGC group. However, in the BWPU 25 NGC group, although GAP 43 grew across the interface between the NGC and the nerve, it seemed to “collapse” near the interface, and almost no extended GAP 43 was found in the deep part. GAP 43 in the proximal nerve stump also formed a ring arrangement in BWPU 25 NGCs, similar to that in FAK-positive cells. The results of cell adhesion and axon growth cone formation and elongation indicated that BWPU NGCs changed cell adhesion after injury. Moreover, different moduli of BWPU NGCs affected axon regeneration in animals implanted with NGCs.
4. Discussion
NGCs as a replacement strategy to substitute autologous nerve grafting for PNS repair have shown efficacy in numerous studies. Polyurethane elastomer is an effective material for PNS regeneration.15,34 In this study, we designed and manufactured BWPU-based NGCs. These NGCs were subjected to BWPU film application as the external conduct of NGC and porous BWPU scaffold as an internal component, which was expected to mimic the structure of the autograft. In these BWPU-based NGCs, the BWPU film may guide nerve regeneration in a stable direction to avoid misdirected axonal outgrowth. Moreover, the BWPU film not only made the conduit sufficiently flexible for surgical suturing, but also hampered the invasion of surrounding cells to avoid postoperative adhesions of the nerve and further prevent neuralgia that may be caused by these adhesions. The inner BWPU scaffold has a porous structure that can stimulate the ECM, which may provide a microenvironment for neuronal growth and facilitate nutrient exchange during nerve regeneration. Moreover, the BWPU NGCs developed here have the advantage of a tunable modulus by simplifying the adjustment of the PEG content in the soft segments within the BWPU. These factors make it possible to investigate the effect of mechanical properties on PNS regeneration in a relatively stable and homogeneous NGC system.
The basic requirement for PNS NGC is that it can facilitate PNS regeneration and promote functional recovery. Furthermore, the NGC should be biodegradable to prevent the potential risk of compression to the regenerated nerve and chronic inflammation, which may cause the long-term failure of nerve repair.35,36 Our in vivo results indicated that in the 10-mm transected injury, BWPU 17 NGCs showed complete sciatic nerve regeneration with axon regeneration and myelination, as well as significantly improved sciatic nerve function and gastrocnemius muscle reinnervation. In this study, the BWPU 17 NGCs showed stronger effects of sciatic nerve regeneration than those of autologous grafts. Both BWPU NGC groups were completely degraded at 12 weeks post-injury. However, BWPU 25 NGCs had better results for nerve repair than the control, but showed significantly poorer outcomes in sciatic nerve regeneration than BWPU 17 NGCs. The only difference between the BWPU 17 NGCs and BWPU 25 NGCs was their different moduli. Hence, we speculated that the mechanical properties may affect the outcome of PNS regeneration.
During PNS regeneration, key pathophysiological processes can be divided into the following three stages: (1) Wallerian degeneration, which starts 24 h after injury and is characterized by the disruption of the axon and myelin sheath;37 (2) ECM transformation, wherein within 2 weeks post-injury, macrophages migrate and infiltrate the lesion to clear cell debris and facilitate regeneration;38,39 (3) nerve regeneration, wherein at 4 to 20 days post-injury, Schwann cells proliferate to form bands of Büngner, the stump of axons forms growth cones, and sprouting neonatal axons elongated to bands of regenerated axons at a speed of 1–2.5 mm per day. Hence, the different mechanical properties of BWPU NGCs may affect PNS regeneration through ECM transformation and/or axon regeneration.40,41
Our results indicated that at 2 weeks post-injury, in both BWPU 17 NGC and BWPU 25 NGC groups, macrophages tended to be activated into M2 phenotypes, which promoted the nerve repair process and inhibited inflammation.42,43 Moreover, the BWPU 25 NGC group showed a higher activation proportion of M2 macrophages and stronger anti-inflammatory effects. The alteration of macrophages in the ECM indicates that ECM transformation and macrophage phenotype may not be the mechanisms of discrepant outcomes induced by different BWPU NGCs in PNS regeneration. Subsequently, we investigated cell adhesion and axon regeneration in the BWPU 17 NGC and BWPU 25 NGC groups. The results revealed that the density of FAKs, which triggers a cascade of phosphorylation reactions following injury leading to the generation of mechanical forces and the transcription and regulation of specific genes responsible for cell growth and differentiation,44,45 did not differ between the two groups. Finally, we found that GAP 43 growth cone and axon regeneration “collapsed” near the interface between the scaffold and the nerve in BWPU 25 NGCs, and almost no GAP 43 extended into the deep part of the scaffold. This finding indicated that the dysfunction of axon regeneration may be responsible for the different PNS regeneration outcomes of BWPU NGCs with different mechanical properties.
According to our results, BWPU NGCs with different moduli influence the sciatic nerve mainly through GAP 43 intensity and extension. The ECM mechanical properties play a key role in regulating development, homeostasis, regenerative processes, and disease progression.46–48 PNS is believed to show a non-linear stress–strain characteristic when placed under tension.27 The elasticity of nerves is not only for the protection of the nerve fascicles but also for the integrity of neurophysiological function.19 Healthy sciatic nerve collagens type I and IV, as major contributors, provide much of its resilience characteristics.49,50 We suspect that during sciatic nerve regeneration, the movement of animal BWPU NGCs will withstand forces from every direction. Moreover, the contraction of muscles and pressure of the soft tissue also directly acted on BWPU NGCs. Among these interactions, the most dynamic and direct might be the interface between the implant and the nerve stump during nerve regeneration induced by BWPU NGCs.51 We speculated that 2 weeks after grafting, BWPU NGCs altered the regeneration of ECM through transforming the macrophage phenotype as well as the expression of FAKs to promote nerve repair. As a consequence of the growth-promoting effect of BWPU NGCs, growth cones in the nerve stump proliferated and sprouted axons into the scaffold of BWPU NGCs. In the BWPU 17 NGCs, these newly generated axons grew smoothly across the interface between the NGCs and the nerve stump and elongated in the direction of the NGCs. During the whole process of nerve regeneration, the BWPU 17 NGCs provided powerful support to axons and were strong enough to resist the external force caused by movement or muscle contraction. However, the BWPU 25 NGCs, which have weaker mechanical properties, may not have sufficient strength to support axon growth. Hence, we observed “collapsed” axons because of the elastic recoil and atrophy of axons. Finally, these contracted axons, combined with fibrous tissues, formed curly arranged NF-200 at 12 weeks post-injury. In addition, due to poor mechanical properties, BWPU 25 NGCs cannot support growth cones and axons to bands of Büngner, which may further cause the atrophy of axons and failure of remyelination in the regenerated axons. As a consequence, a significant decrease in S-100 was observed in the BWPU 25 NGCs.
As mentioned previously, we have revealed how the mechanical properties of NGCs affect the results of PNS regeneration in an animal model. In addition, we discovered the changes in ECM macrophages and cell adhesion induced by BWPU NGCs during PNS regeneration. Our results indicate that the mechanical properties are likely to play an important role in PNS regeneration. We believe that the influence of mechanical properties on PNS regeneration should be considered in future biomaterial NGC designs.
5. Conclusions
In this study, we demonstrated the viability of BWPU NGCs in PNS regeneration and showed that BWPU NGCs are capable of facilitating PNS repair. BWPU NGCs exhibited a nerve-like structure with an outer membrane and an ECM-like porous inner structure. We synthesized two types of BWPU NGCs – BWPU 17 NGCs and BWPU 25 NGCs – with different moduli by modifying the molar content of PEG in the soft segments within the BWPU. The results of sciatic nerve repair showed that BWPU 17 NGCs could effectively repair PNS, which was comparable to the current gold standard autograft implantation. Both BWPU NGCs polarize ECM macrophages to M2 phenotypes and enhance FAK expression to promote PNS regeneration. First, we revealed that the modulus of BWPU NGCs could influence the outcome of PNS regeneration by affecting GAP 43, which forms a growth cone and regulates axon growth. Altogether, we have successfully developed BWPU NGCs with a tunable modulus to achieve effective peripheral nerve repair in long-gap transected injury and revealed the mechanism by which the mechanical properties of NGCs impact the outcome of PNS regeneration in this BWPU NGC system.
Author contributions
Conceptualization and methodology: Y. C. W., R. C. L., and H. T. Materials synthesis: J. J. L., J. H. L., J. L. C., H. T., and Q. F. Investigation, analysis, and validation: Y. C. W., J. J. L., Q. Z., M. J. W., and X. H. H. Writing – original draft: Y. C. W. Writing – review and editing: X. H. H. and H. T.
Conflicts of interest
There are no conflicts to declare.
Acknowledgements
We thank Ping Liao from the Translational Neuroscience Center for the technical support of the confocal laser scanning microscope, Xiaomei Zhang and Xu Peng from the laboratory animal center, Sichuan University, for raising animals. This work was financially supported by the National Natural Science Foundation of China key program grant 51733005, the National Natural Science Foundation of China young scientists fund grants 52003177 and 81902549, and the Post-Doctor Research Project, West China Hospital, Sichuan University grant 2020HXBH158.
References
- L. Padua, D. Coraci, C. Erra, C. Pazzaglia, I. Paolasso, C. Loreti, P. Caliandro and L. D. Hobson-Webb, Lancet, 2016, 15, 1273–1284 CrossRef PubMed.
- R. Brull, A. Hadzic, M. A. Reina and M. J. Barrington, Reg. Anesth. Pain Med., 2015, 40, 479–490 CrossRef CAS PubMed.
- A. Faroni, S. A. Mobasseri, P. J. Kingham and A. J. Reid, Adv. Drug Delivery Rev., 2015, 82–83, 160–167 CrossRef CAS PubMed.
- G. Yiu and Z. He, Nat. Rev. Neurosci., 2006, 7, 617–627 CrossRef CAS PubMed.
- M. Mahar and V. Cavalli, Nat. Rev. Neurosci., 2018, 19, 323–337 CrossRef CAS PubMed.
- A. Beris, I. Gkiatas, I. Gelalis, D. Papadopoulos and I. Kostas-Agnantis, Eur. J. Orthop. Surg. Traumatol., 2019, 29, 263–269 CrossRef PubMed.
- R. Li, Z. Liu, Y. Pan, L. Chen, Z. Zhang and L. Lu, Cell Biochem. Biophys., 2014, 68, 449–454 CrossRef CAS PubMed.
- D. W. Hewson, N. M. Bedforth and J. G. Hardman, Anaesthesia, 2018, 73(Suppl. 1), 51–60 CrossRef PubMed.
- A. Magaz, A. Faroni, J. E. Gough, A. J. Reid, X. Li and J. J. Blaker, Adv. Healthcare Mater., 2018, 7, e1800308 CrossRef PubMed.
- Y. C. Chang, M. H. Chen, S. Y. Liao, H. C. Wu, C. H. Kuan, J. S. Sun and T. W. Wang, ACS Appl. Mater. Interfaces, 2017, 9, 37623–37636 CrossRef CAS PubMed.
- X. Li, W. Yang, H. Xie, J. Wang, L. Zhang, Z. Wang and L. Wang, ACS Appl. Mater. Interfaces, 2020, 12, 36860–36872 CrossRef CAS PubMed.
- Z. Zhang, M. L. Jørgensen, Z. Wang, J. Amagat, Y. Wang, Q. Li, M. Dong and M. Chen, Biomaterials, 2020, 253, 120108 CrossRef CAS PubMed.
- X. Gu, F. Ding and D. F. Williams, Biomaterials, 2014, 35, 6143–6156 CrossRef CAS PubMed.
- Y. Hou, X. Wang, Z. Zhang, J. Luo, Z. Cai, Y. Wang and Y. Li, Adv. Healthcare Mater., 2019, 8, e1900913 CrossRef PubMed.
- Y. W. Chen, C. C. Chen, H. Y. Ng, C. W. Lou, Y. S. Chen and M. Y. Shie, Polymers, 2019, 11, 1962 CrossRef PubMed.
- Y. Niu and M. Galluzzi, J. Mater. Chem. B, 2020, 8, 11063–11073 RSC.
- O. Chaudhuri, J. Cooper-White, P. A. Janmey, D. J. Mooney and V. B. Shenoy, Nature, 2020, 584, 535–546 CrossRef CAS PubMed.
- H. Zhang, X. Zheng, W. Ahmed, Y. Yao, J. Bai, Y. Chen and C. Gao, Biomacromolecules, 2018, 19, 1746–1763 CrossRef CAS PubMed.
- H. M. Howarth, T. Alaziz, B. Nicolds, S. O'Connor and S. B. Shah, Neural Regen. Res., 2019, 14, 1280–1288 CrossRef PubMed.
- K. Adebowale, Z. Gong, J. C. Hou, K. M. Wisdom, D. Garbett, H. P. Lee, S. Nam, T. Meyer, D. J. Odde, V. B. Shenoy and O. Chaudhuri, Nat. Mater., 2021 DOI:10.1038/s41563-021-00981-w.
- M. C. Mosley, H. J. Lim, J. Chen, Y. H. Yang, S. Li, Y. Liu and L. A. Smith Callahan, J. Biomed. Mater. Res., Part A, 2017, 105, 824–833 CrossRef CAS PubMed.
- G. Rosso and J. Guck, APL Bioeng., 2019, 3, 036107 CrossRef PubMed.
- A. M. Stiller, M. A. González-González, J. M. Boothby, S. E. Sherman, J. Benavides, M. Romero-Ortega, J. J. Pancrazio and B. J. Black, J. Neural Eng., 2019, 16, 064001 CrossRef PubMed.
- X. Jiang, F. Yu, Z. Wang, J. Li, H. Tan, M. Ding and Q. Fu, J. Biomater. Sci., Polym. Ed., 2010, 21, 1637–1652 CrossRef CAS PubMed.
- T. Chomiak and B. Hu, PLoS One, 2009, 4, e7754 CrossRef PubMed.
- B. Sun, Z. Zhou, T. Wu, W. Chen, D. Li, H. Zheng, H. El-Hamshary, S. S. Al-Deyab, X. Mo and Y. Yu, ACS Appl. Mater. Interfaces, 2017, 9, 26684–26696 CrossRef CAS PubMed.
- M. K. Kwan, E. J. Wall, J. Massie and S. R. Garfin, Acta Orthop. Scand., 1992, 63, 267–272 CrossRef CAS PubMed.
- L. E. Kokai, Y. C. Lin, N. M. Oyster and K. G. Marra, Acta Biomater., 2009, 5, 2540–2550 CrossRef CAS PubMed.
- G. Thiel, Brain Pathol., 1993, 3, 87–95 CrossRef CAS PubMed.
- G. Fanò, S. Biocca, S. Fulle, M. A. Mariggiò, S. Belia and P. Calissano, Prog. Neurobiol., 1995, 46, 71–82 CrossRef.
- S. Schiaffino, K. A. Dyar, S. Ciciliot, B. Blaauw and M. Sandri, FEBS J., 2013, 280, 4294–4314 CrossRef CAS PubMed.
- Y. Wu, L. Wang, T. Hu, P. X. Ma and B. Guo, J. Colloid Interface Sci., 2018, 518, 252–262 CrossRef CAS PubMed.
- M. R. Holahan, Front. Cell. Neurosci., 2017, 11, 266 CrossRef PubMed.
- Y. Niu, K. C. Chen, T. He, W. Yu, S. Huang and K. Xu, Biomaterials, 2014, 35, 4266–4277 CrossRef CAS PubMed.
- T. A. Smith, C. L. Ghergherehchi, H. O. Tucker and G. D. Bittner, J. Neuroinflammation, 2020, 17, 287 CrossRef CAS PubMed.
- D. Wu, Y. Jin, T. M. Shapiro, A. Hinduja, P. W. Baas and V. J. Tom, Nat. Commun., 2020, 11, 6131 CrossRef CAS PubMed.
- P. Chen, X. Piao and P. Bonaldo, Acta Neuropathol., 2015, 130, 605–618 CrossRef CAS PubMed.
- R. E. Zigmond and F. D. Echevarria, Prog. Neurobiol., 2019, 173, 102–121 CrossRef CAS PubMed.
- M. P. Clements, E. Byrne, L. F. Camarillo Guerrero, A. L. Cattin, L. Zakka, A. Ashraf, J. J. Burden, S. Khadayate, A. C. Lloyd, S. Marguerat and S. Parrinello, Neuron, 2017, 96, 98–114 CrossRef CAS PubMed.
- K. R. Jessen, R. Mirsky and A. C. Lloyd, Cold Spring Harbor Perspect. Biol., 2015, 7, a020487 CrossRef PubMed.
- K. R. Jessen and R. Mirsky, J. Physiol., 2016, 594, 3521–3531 CrossRef CAS PubMed.
- T. C. Huang, H. L. Wu, S. H. Chen, Y. T. Wang and C. C. Wu, J. Neuroinflammation, 2020, 17, 240 CrossRef PubMed.
- N. Mokarram, K. Dymanus, A. Srinivasan, J. G. Lyon, J. Tipton, J. Chu, A. W. English and R. V. Bellamkonda, Proc. Natl. Acad. Sci. U. S. A., 2017, 114, E5077–E5084 CrossRef CAS PubMed.
- J. Wörthmüller and C. Rüegg, Int. J. Mol. Sci., 2020, 21, 9107 CrossRef PubMed.
- L. F. Castelnovo, V. Bonalume, S. Melfi, M. Ballabio, D. Colleoni and V. Magnaghi, Neural Regen. Res., 2017, 12, 1013–1023 CrossRef CAS PubMed.
- J. Swift, I. L. Ivanovska, A. Buxboim, T. Harada, P. C. Dingal, J. Pinter, J. D. Pajerowski, K. R. Spinler, J. W. Shin, M. Tewari, F. Rehfeldt, D. W. Speicher and D. E. Discher, Science, 2013, 341, 1240104 CrossRef PubMed.
- C. Yang, M. W. Tibbitt, L. Basta and K. S. Anseth, Nat. Mater., 2014, 13, 645–652 CrossRef CAS PubMed.
- A. C. de Luca, S. P. Lacour, W. Raffoul and P. G. di Summa, Neural Regen. Res., 2014, 9, 1943–1948 Search PubMed.
- S. Ugrenović, I. Jovanović and L. Vasović, Microsc. Res. Tech., 2011, 74, 1127–1133 CrossRef PubMed.
- G. Keilhoff, F. Stang, G. Wolf and H. Fansa, Biomaterials, 2003, 24, 2779–2787 CrossRef CAS PubMed.
- S. Patel, J. M. Caldwell, S. B. Doty, W. N. Levine, S. Rodeo, L. J. Soslowsky, S. Thomopoulos and H. H. Lu, J. Orthop. Res., 2018, 36, 1069–1077 CrossRef PubMed.
Footnote |
† Electronic supplementary information (ESI) available. See DOI: 10.1039/d1tb01236c |
|
This journal is © The Royal Society of Chemistry 2021 |