One-pot, self-catalyzed synthesis of self-adherent hydrogels for photo-thermal, antimicrobial wound treatment†
Received
7th September 2020
, Accepted 30th October 2020
First published on 31st October 2020
Abstract
Self-adhering hydrogels are promising materials to be employed as wound dressings, because they can be used for wound healing without the necessity of additional stitching. However, micro-organisms can easily adhere to these hydrogels as well, which usually causes wound infections. Therefore, adhesive hydrogels are often combined with antibiotics. However, this introduces a risk of drug resistance, cytotoxicity and poor cell affinity. Consequently, recently, there has been great interest in developing non-antibiotic, antibacterial adhesive hydrogels. In this article, we present a simple one-pot synthesis procedure to prepare self-adhesive hydrogels composed of poly(acrylamide) (PAM), naturally derived chitosan (CS) and tannic acid/ferric ion chelates (TA@Fe3+). TA@Fe3+ enables self-catalysis of the polymerization reaction. In addition, due to its near infrared (NIR) photothermal responsiveness, TA@Fe3+ allows for eliminating the bacterial activity with up to 91.6% and 94.7% effectivity against Escherichia coli and Staphylococcus aureus, respectively. Mechanical and adhesion testing shows that the hydrogels are tough as well as flexible and will adhere repeatedly to many types of biological tissues, which can be attributed to the combination of physical and chemical bonding between TA@Fe3+ and PAM and CS, respectively. Moreover, in vitro and in vivo tests indicate that the NIR photothermally active hydrogel can effectively prevent bacterial infection and accelerate tissue regeneration, which demonstrates that these hydrogels are promising functional materials for wound healing applications.
1. Introduction
Skin is the largest organ of the human body, and protects the internal tissue and organs. Due to trauma or diseases, (open) skin wounds can be formed such that sensitive tissue is exposed to physical, chemical and pathogenic invasion. Additionally, without appropriate treatment, these wounds can get infected by microorganisms which delays wound healing and can even cause tissue necrosis.1–3 Therefore, wound dressings are needed to cover and help recover the injured skin. There are numerous forms of commercially available dressings on the market, such as hydrogels, cryogels and fibers. Among these, hydrogels are the most popular wound dressings, as they are moist and composed of three-dimensional network structures that mimic the extracellular matrix microenvironment, which is in favor of wound healing and skin tissue regeneration.4–7
Compared to clinically employed hydrogel dressings, self-adhering hydrogels have clear advantages, as they can be conveniently applied on the damaged body tissues/organs without the need for additional stitches or glue.8–10 However, hydrogels with self-adhesiveness can also easily adhere to microorganisms, which can induce wound infection.11 Thus, it is essential to develop adhesive hydrogels with antibacterial properties. Currently, immobilization of antimicrobial antibiotics in hydrogels is the most applied antibacterial strategy.12–14 However, overuse of antibiotics may cause long-term cytotoxicity and there is a risk that the bacteria become drug-resistant, which will worsen the wound infection and can even result in death.15–17 Therefore, it is critical to design antibacterial and adhesive hydrogels for wound dressing without the need for antibiotics.
In recent years, near infrared (NIR) photothermal treatment has been extensively explored as a potential technique to eliminate bacteria due to several key advantages, such as its broad antibacterial spectrum,18 opportunity for remote control19 and for avoiding the risk of drug resistance.20 Various NIR photosensitizers, such as gold nanoparticles,21 semiconductor nanoparticles,22 carbon-based nanomaterials23 and polymer composites,24,25 have been developed for NIR photothermal therapy in battling tumors and bacteria. Nevertheless, most of the NIR photosensitizers have their own disadvantages, including difficult biodegradation, poor cell affinity and high cost, and are not conducive for clinical applications.
As naturally derived NIR photosensitizers, tannic acid/ferric ion chelates (TA@Fe3+) have gained a lot of attention, due to their good NIR photothermal conversion efficiency, biocompatibility and biodegradability.26,27 TA is a natural polyphenol of plants and it shows an excellent cell affinity on biomaterial surfaces.28,29 The good availability of catechol groups allows TA to adhere to many different substrates through hydrogen bonding and electrostatic and π–π interaction.30–32 Moreover, the incorporation of TA@Fe3+ in hydrogels can effectively enhance their flexibility and recoverability due to the dynamic physical crosslinking networks.33,34 Very recently, it has been reported that catechol–metal ion complexes can also accelerate free radical polymerization, because the formed redox pairs can act as a self-catalytic system to activate free radicals.35 The photothermal TA@Fe3+ agents also belong to redox pairs, and thus we anticipate them to be able to self-catalyze free radical polymerization as well.
Herein, we report a simple synthesis route to prepare non-antibiotic and NIR photothermally active self-adhering, biocompatible hydrogels, which accelerate skin wound healing. As shown in Scheme 1, the hydrogels were prepared by a one step in situ self-catalyzed polymerization via TA@Fe3+. The formed hybrid chemically and physically crosslinked network endows the hydrogels with good flexibility and recoverability. In addition, the good availability of catechol groups of TA allows the hydrogels to adhere repeatedly to different types of tissues. Under NIR light irradiation, the hydrogels exhibit notable photothermal antibacterial activity to prevent microbial infection, as shown in both in vitro and in vivo experiments. Moreover, the biocompatible TA@Fe3+ can upregulate the gene expression of smooth muscle α-actin and collagen type III (COL III) of fibroblasts to accelerate wound healing. Therefore, biocompatible and photothermally responsive adhesive hydrogels are promising candidates to be utilized in clinical applications as functional platforms for skin wound healing.
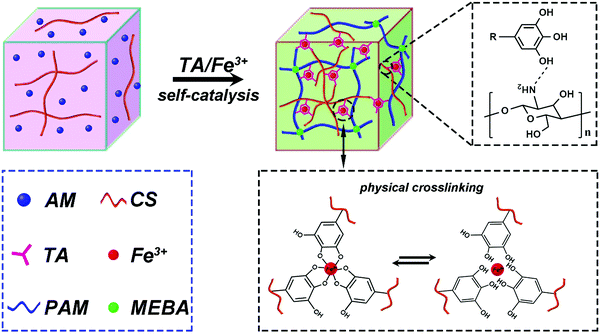 |
| Scheme 1 Preparation of the PAM/CS hydrogel incorporated with TA@Fe3+. | |
2. Materials and methods
2.1. Preparation of TA@Fe3+ incorporated hydrogels
All hydrogels were prepared by a one-step free radical polymerization reaction. First, CS powder was dissolved in acetic acid (0.2 v/v%) to obtain CS solution (0.5 wt%). Then, acrylamide (AM), TA and FeCl3·6H2O were dissolved in the prepared CS solution separately. After that, the initiator (ammonium persulphate, APS), crosslinker (N,N′-methylene bisacrylamide, MEBA) and catalyzer (N,N,N′,N′-tetramethylethylenediamine, TMEDA) were dissolved into the above mixture to obtain a homogeneous prepolymer solution. Finally, the prepolymer solution was added to various reaction molds and kept for 10 min to obtain the TA@Fe3+ incorporated hydrogels. The component mass of the hydrogels with various TA@Fe3+ concentrations is listed in Table S1 (ESI†).
2.2. Characterization of hydrogels
Morphologies of the hydrogels were characterized using a scanning electron microscope (SEM, JSM 6390, JEOL, Japan). First, the hydrogels (Φ 10 × 12 mm) were frozen in refrigerators at −20 °C for 24 h. Then the frozen hydrogels were freeze-dried using a freeze dryer. To investigate the swelling performance, all the hydrogels (Φ 10 × 1 mm) were immersed in PBS solutions to swell until an equilibrium state was reached at 25 °C. Mechanical property analysis and adhesion tests of the hydrogels were performed by using a universal test machine (Instron 5567, USA). The details are described in the ESI.†
2.3. NIR photothermal properties of hydrogels
The NIR photothermal activity of the hydrogels was determined by irradiating them with a NIR laser. The hydrogels (Φ 10 × 1 mm) were placed in a tube with distilled water (1 mL) and irradiated with a NIR laser (808 nm, 2 W cm−2) for 15 min. Every 0.5 min, the temperature of distilled water in the tube was measured with a thermal imager (XINTEST, China). To evaluate the photothermal stability of the TA-1500 hydrogel, we repeatedly measured the temperature cycle curves of the TA-1500 hydrogel five times. In each repeat cycle, the TA-1500 hydrogel was irradiated for 10 min with a NIR laser. Then, it was cooled to room temperature naturally.
2.4. Antibacterial activity in vitro
The antibacterial activity of the hydrogels was evaluated in vitro using the turbidimetric method and the flat colony counting method. Staphylococcus aureus (S. aureus) and Escherichia coli (E. coli) were cultured for seven groups of samples (Φ 10 × 1 mm), respectively, including a blank and PAM, PAM/CS, TA-500, TA-1000, TA-1500 and TA-2000 hydrogels. Then, each group was randomly divided into two groups for NIR/NIR free (2 W cm−2, 15 min) irradiation. Finally, the antibacterial properties of various hydrogels were evaluated by the turbidimetric method and the flat colony counting method. The details of the antibacterial tests are described in the ESI,† following the procedures reported in ref. 36.
2.5. Cell experiment in vitro
The cytocompatibility of the hydrogels, including the PAM hydrogel, PAM/CS hydrogel, TA-500 hydrogel, TA-1000 hydrogel, TA-1500 hydrogel and TA-2000 hydrogel, was evaluated by culturing fibroblasts (3T3 cells) on their surfaces. 3T3 cells (5 × 104 cells per sample) were allowed to spread on the hydrogels, and their morphologies were examined by a confocal laser scanning microscopy (CLSM) after being stained with calcein-AM and propidium iodide on day 3. In addition, after 3 and 5 days of culture, proliferation of the cells seeded on the hydrogels was evaluated by 3-(4,5-dimethylthiazol-2-yl)-2,5-diphenyltetrazolium bromide (MTT) assay. The relative gene expression levels of smooth muscle α-actin and COL III were analyzed quantitatively by quantitative real-time polymerase chain reaction (qRT-PCR). The detailed evaluation is described in the ESI,† as also reported in ref. 18.
2.6. Antibacterial activity in vivo
The in vivo antibacterial activity of the hydrogels was evaluated by using Sprague-Dawley (SD) rats as subcutaneous models. Briefly, the hydrogels (Φ 5 × 1 mm), including PAM/CS hydrogel and TA-1500 hydrogel, were implanted in subcutaneous pockets on the back of the SD rats. After implantation of the hydrogels, an E. coli suspension (0.1 mL, 108 CFU mL−1) was dropped on the subcutaneous pocket. Then, the SD rats were randomly divided into two groups, NIR (2 W cm−2, 15 min) and non-NIR irradiation groups. After 7 days of implantation, the rats were sacrificed for hematoxylin–eosin (H&E) staining and Giemsa staining. The detailed steps can be found in the ESI,† as also reported in ref. 37.
2.7. Animal experiment in vivo
To evaluate the effect of the hydrogels on skin wound recovery, they were covered on the wounds to repair full-thickness skin defects of the SD rats. Briefly, skin wound defects (diameter of 8 mm) were created on the back of each rat. Next, three hydrogel groups (Φ 8 × 1 mm), including PAM/CS hydrogel, TA-1500 hydrogel and epidermal growth factor (EGF) loaded TA-1500 hydrogel, were covered on the defects, and the groups without any treatment were set as a control. Lastly, skin tissues around the hydrogels were harvested for H&E staining after 15 days of implantation. The detailed process for the animal experiment is described in the ESI,† as also reported in ref. 2 and 38.
3. Results and discussion
3.1. Fabrication of hydrogels
The hydrogels were prepared by in situ self-catalysis by simply mixing all the components in one pot. Polymerization of AM usually requires a toxic catalyst (TMEDA) and heating to accelerate the decomposition of APS to produce free radicals in a traditional synthesis process. In contrast, the self-catalytic system based on TA@Fe3+ shows a more convenient and green process of preparation because of avoiding the use of TMEDA and heating. The mechanism of self-catalytic polymerization based on TA@Fe3+ is shown in Fig. 1a. The redox pairs of TA@Fe3+ can effectively activate APS to generate free radicals, which further induced the polymerization of AM.35 For verifying the self-catalytic function of TA@Fe3+, the systems with only TA or Fe3+ were applied for triggering the polymerization. As shown in Fig. 1b, no hydrogels could be formed in these systems even after 60 mins, whereas, once TA@Fe3+ was added, fast polymerization happened to form hydrogels in less than 10 mins. The results indicate that TA@Fe3+ can replace the toxic catalyst (TMEDA) to self-catalyze free radical polymerization in situ, which offers a simple and green method for the preparation of biomedical hydrogels.
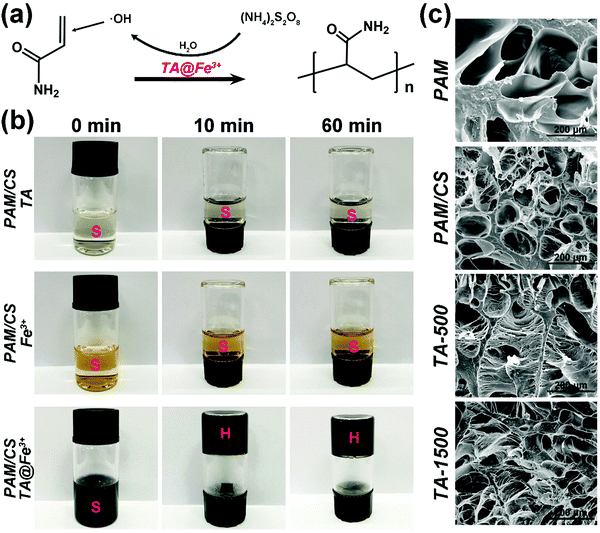 |
| Fig. 1 (a) Mechanism of self-catalytic gelation based on TA@Fe3+. (b) Photographs of polymerization of AM into the hydrogel catalyzed by TA@Fe3+. (c) SEM micrographs of the hydrogels, including PAM hydrogel, PAM/CS hydrogel, TA-500 hydrogel and TA-1500 hydrogel. S: solution; H: hydrogel. | |
3.2. Morphological structures of hydrogels
As shown in Fig. 1c, the SEM micrographs reveal that all the hydrogels show rich microporous structures, which is in favor of cell adhesion, proliferation and tissue growth.39,40 Moreover, the average pore aperture of the CS incorporated hydrogels is smaller than that of the pure PAM hydrogel, which indicates that interpenetrating networks are more tightly entangled than single polymer networks.41 In addition, the introduction of CS is needed for TA@Fe3+ precipitation (see also Fig. S1, ESI†) and it reduces the equilibrium swelling ratio of the hydrogels (Fig. S3c, ESI†). Interestingly, after the addition of TA@Fe3+, plenty of microfibrils appeared uniformly to form well-connected networks in the micropore of the TA-500 hydrogel and the TA-1500 hydrogel, which is similar to the ultrastructure of the byssal adhesive plaque.42 As a key feature of the TA@Fe3+ incorporating hydrogels, the microfibrils are constructed via interactions between TA (polyphenols) and polymer chains.43,44
3.3. Mechanical properties of hydrogels
As shown in Fig. 2a and d, the TA-1500 hydrogels can be compressed significantly to a strain of 80% and stretched to 14.2 times of their original shapes without breaking. After removal of the external force, compared to the PAM/CS hydrogel (Fig. S3a, ESI†), the TA-1500 hydrogel could recover to its original shape immediately. In addition, it is easy to tie knots in the TA-1500 hydrogels (Fig. 2g), which indicates good flexibility. The compressive strength image (Fig. 2b) shows that the compressive strength of TA-1500 hydrogel is around 371 kPa at a strain of 80%, which is higher than that of the PAM hydrogels (196 kPa) and lower than that of the PAM/CS hydrogels (516 kPa). As presented in Fig. 2e, the tensile strength was tested as well. The TA-1500 hydrogels display a maximum tensile strength of 22 kPa, which is lower than that of the PAM/CS hydrogels (34 kPa). The compression and tension cycle tests are shown in Fig. 2c and f, respectively. After 5 cycles, the compression and tension loading–unloading curves for the TA-1500 hydrogels almost overlapped with the original ones. All the results above can be explained as follows: first, the introduction of CS significantly increases the mechanical strength of the hydrogels, because the interpenetrating PAM and CS networks are more tough than the single PAM network. Second, the flexibility and recoverability of the hydrogels improved after TA@Fe3+ was incorporated, which might be attributed to the introduction of hybrid physical and chemical crosslinking points. On the one hand, the chemical crosslinking points are stable enough to avoid the destruction of the polymer network by external forces due to their high bond energies. On the other hand, the physically crosslinked TA@Fe3+ can dynamically dissociate and recombine for energy dissipation and avoid stress concentration.33,45
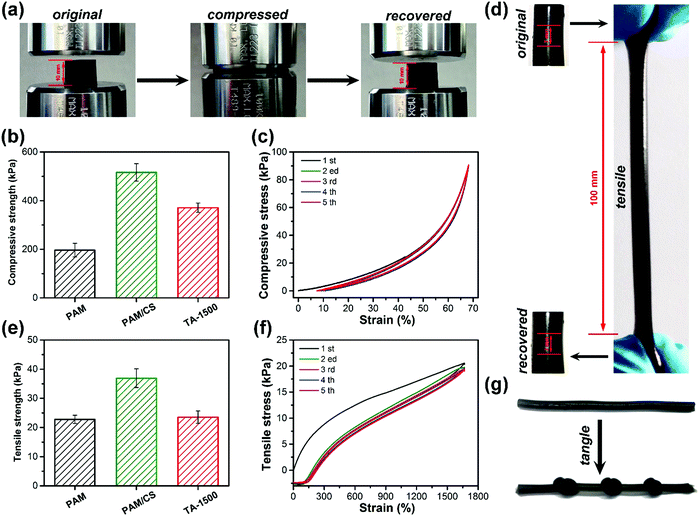 |
| Fig. 2 Mechanical properties of the hydrogels. (a) Compression recovery performance of a piece of TA-1500 hydrogel. (b) Compressive strength of hydrogels. (c) Cyclic compression performance of TA-1500 hydrogels. (d) Tensile recovery performance of TA-1500 hydrogels. (e) Tensile strength of hydrogels. (f) Cyclic tensile properties of TA-1500 hydrogels. (g) Flexibility of the TA-1500 hydrogel. | |
3.4. Adhesive properties of hydrogels
Next, the adhesive strength of the prepared hydrogel was determined. As shown in Fig. 3a, the TA-1500 hydrogel can adhere to various organs of SD rats in air, including the heart, liver, spleen, lung and kidney. In addition, the adhesion strength of the hydrogels in air was evaluated by using a porcine skin adhesive model (Fig. 3b). As shown in Fig. 3c, we find that the adhesive strength of the TA@Fe3+ incorporated hydrogels is higher than that of the PAM and PAM/CS hydrogels, because they benefit from the rich catechol groups of TA. Compared to commercial Cosmopor® E dressings, the TA-1500 hydrogel shows about 2.5 times higher adhesive strength (Fig. S3b, ESI†). Moreover, the adhesion cycle tests (Fig. 2d) demonstrate that the adhesion strength of the TA-1500 hydrogels does not significantly decrease after 20 cycles, which indicates that the performance of these hydrogels is repeatable and durable.
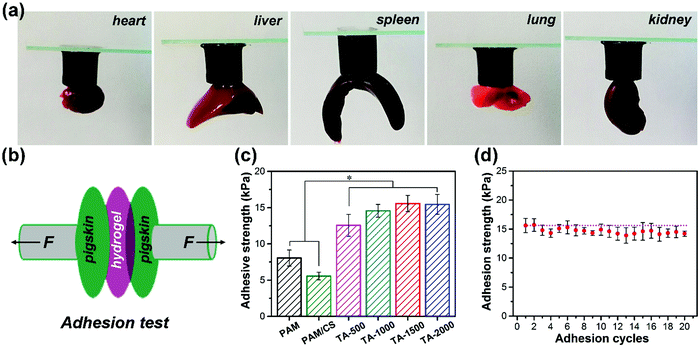 |
| Fig. 3 Adhesive properties of hydrogels. (a) TA-1500 hydrogel adhered to various organs of SD rats. (b) Adhesion test model. (c) Adhesive strength of the hydrogels. (d) Cyclic adhesion strength of the TA-1500 hydrogel. * indicates the significant difference (p < 0.05). | |
3.5. NIR photothermal efficiency and antibacterial properties in vitro
The photothermal properties of the prepared hydrogels were investigated by recording the temperature changes and thermal images at specific time intervals under NIR laser irradiation. Fig. S5 (ESI†) shows that the temperature of the TA-1500 hydrogel increases from 33 °C to 70 °C under NIR laser irradiation for 15 min, whereas no significant temperature change is detected for the PAM/CS hydrogels. Fig. 4a illustrates that the photothermal activity of various hydrogels depends strongly on the concentration of TA@Fe3+. Higher content of TA@Fe3+ in the hydrogels results in higher temperatures, because the absorption at 808 nm (NIR) was enhanced with the increase of TA@Fe3+ concentration as well (see also Fig. S2, ESI†). Furthermore, Fig. 4b demonstrates that the TA-1500 hydrogels are photothermally stable, as the photothermal effect of the TA-1500 hydrogel does not attenuate for at least five cycles of NIR irradiation. All these results indicate that the hydrogels have a remarkable photothermal conversion ability, which is important for photothermal antibacterial treatment.
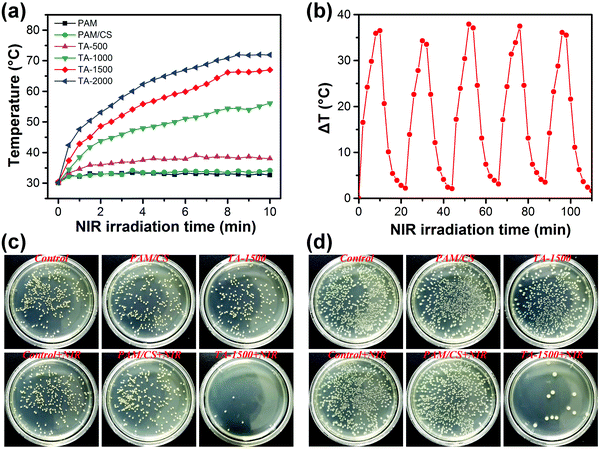 |
| Fig. 4 NIR assisted photothermal antibacterial ability in vitro. (a) Temperature elevation of the hydrogels measured every 0.5 min using a thermometer. (b) Temperature change of the TA-1500 hydrogel during five cycles of laser on/off under NIR laser irradiation. Representative images of bacterial colonies for S. aureus suspensions (c) and E. coli suspensions (d) by spreading their media on agar plates following 24 h of incubation. | |
The antibacterial properties of all the prepared hydrogels were evaluated with or without NIR laser irradiation in vitro. The turbidimetry results in Fig. S5b and c (ESI†) show that the hydrogels have weak antibacterial efficiency against E. coli and S. aureus without NIR laser irradiation. A slight antibacterial activity of the hydrogels might originate from the inherent nature of CS and TA.46,47 After NIR laser irradiation for 15 min, there was no significant difference in the antibacterial ratio of the PAM hydrogel, PAM/CS hydrogel, TA-500 hydrogel and TA-1000 hydrogel. In contrast, the antibacterial ratio of the TA-1500 hydrogel and the TA-2000 hydrogel was more than 90%, which is consistent with the corresponding photothermal results showing that the hydrogels are most photothermally active at higher TA@Fe3+ concentrations.
The colony counting results further confirmed the good NIR photothermally antibacterial activity of TA-1500 (Fig. 4c and d). The number of colonies on the Luria-Bertani (LB) plates of the TA-1500 hydrogel was slightly less than that on the LB plate of the controls and PAM/CS hydrogels without NIR laser irradiation. After NIR laser irradiation for 15 min, only a few colonies could be observed on the LB plate of the TA-1500 hydrogel treated group. Based on these in vitro antibacterial results, it can be concluded that the presence of TA@Fe3+ is a key factor considering the antibacterial activity of the hydrogels under NIR irradiation. Good photothermal efficiency results in high temperatures, which allows for destroying the proteins and enzymes in bacteria.48 In addition, the NIR photothermal effect of the TA-1500 hydrogels on human normal cells was also evaluated (Fig. S6, ESI†). The results showed that the photothermal effect of the TA-1500 hydrogel indeed could cause apoptosis of the fibroblasts to some extent. However, after irradiation, the fibroblasts around the treatment site could quickly proliferate and reach almost the same number as that of the control group. In a word, NIR photothermal therapy showed local toxicity and damaged the normal cells, but the cells could be rapidly recovered by proliferation and migration of the surrounding cells. Briefly, all the above results indicate that the TA-1500 hydrogels display good photothermal antibacterial performance with few effects on the normal cells.
3.6. Cell culture in vitro
In order to test the cell affinity and cytocompatibility of the hydrogels, fibroblasts were first cultured on the surfaces of the hydrogels to investigate cell attachment and proliferation. The CLSM images in Fig. 5a show that the fibroblasts can attach and spread on the surfaces of all the prepared hydrogels. In addition, the number of living cells on the surfaces of the TA@Fe3+ incorporated hydrogels is higher than that of the hydrogels without TA@Fe3+, including PAM and PAM/CS hydrogels, which reveals that the incorporation of TA@Fe3+ increases the cell affinity of the hydrogels. Moreover, the cell proliferation on various hydrogels was evaluated by MTT assay (Fig. 5b). After 5 days of incubation, the cell proliferation of the TA@Fe3+ incorporated hydrogel was faster than that of the hydrogels without TA@Fe3+, which indicated that the introduction of TA@Fe3+ improves the cytocompatibility of hydrogels to promote cell proliferation.
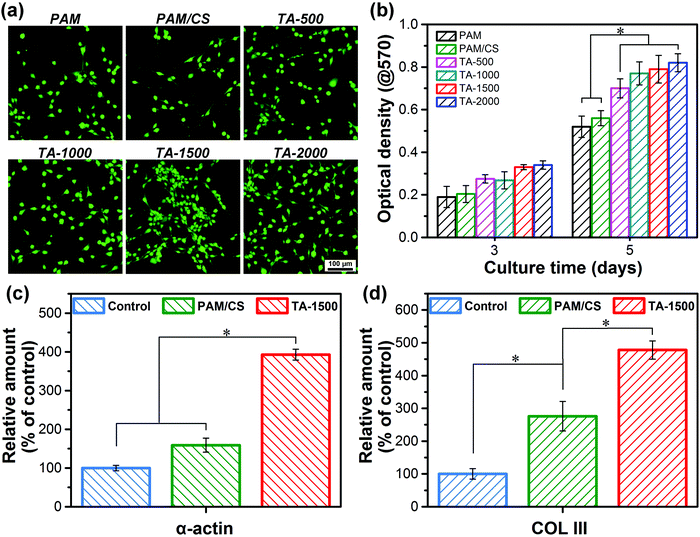 |
| Fig. 5 (a) Representative CLSM micrographs of 3T3 cells after 3 days of culture on the hydrogels. (b) Proliferation of 3T3 cells after 3 and 5 days of culture on the hydrogels. Relative gene expression of (c) smooth muscle α-actin and (d) COL III of the 3T3 cells as evaluated after being cultured with hydrogels for 3 days. * indicates the significant difference (p < 0.05). | |
In short, as a green, safe and non-toxic NIR photosensitizer, the introduction of TA@Fe3+ can improve the cell affinity of the hydrogels to enhance the attachment and proliferation of fibroblasts on the surface of the hydrogels, for which we have the following explanation. First of all, the flexibility and the microporous structures of the hydrogels are beneficial for attachment, elongation, and growth of the fibroblasts. Previous research has demonstrated that soft porous 3D matrices are more favorable for cells than stiff matrices.49–51 Secondly, the rich number of catechol groups in the TA@Fe3+ incorporated hydrogels can explain the hydrogel's excellent adhesive performance in recruiting and capturing suspended cells onto the surface of the hydrogel. Many studies demonstrate that the catechol groups act as adhesive sites and can promote cell adhesion to the matrix.52,53 Thirdly, the rich number of catechol groups introduced by the presence of TA@Fe3+ can be used as multiple bioactive sites to capture the growth factor from cells and serum proteins from the culture medium to promote cell behavior.
In order to further analyze the effect of TA@Fe3+ on cell behavior, qRT-PCR tests were performed to estimate the relative gene expression, such as smooth muscle α-actin and COL III. As shown in Fig. 5c and d, the gene expression levels of smooth muscle α-actin and COL III in the TA-1500 hydrogel groups were significantly higher than those of the control and the PAM/CS hydrogel treated group. As is well known, the expression level of smooth muscle α-actin is critical to skin wound healing, as it can cause fibroblast proliferation and differentiation into myofibroblasts.37 In addition, the expression of COL III can directly affect granulation tissue reorganization and basement membrane regeneration.37 Therefore, the TA@Fe3+ complex has the potential to promote tissue regeneration and accelerate wound healing. Based on the antibacterial test and cell culture results, we can conclude that the TA-1500 hydrogel possesses both NIR photothermal antimicrobial activity and positive cell affinity, which are key for application as wound dressings for skin repair.
3.7. NIR photothermal assisted antibacterial properties in vivo
To evaluate the antibacterial activity of the prepared hydrogels in vivo, the models of SD rats with subcutaneous infection were applied as shown in Fig. 6a. The photothermal images (Fig. S7a, ESI†) show that the temperature around the TA-1500 hydrogel increased by about 40 °C after NIR laser irradiation, whereas less temperature increase is observed for the PAM/CS treated group under the same conditions. The above results indicate that NIR can effectively penetrate the skin tissue and stimulate the TA-1500 hydrogels to produce local high temperatures for bacterial disinfection. The photographs of the surgical sites are shown in Fig. 6b. After 7 days, the wounds treated with the TA-1500 hydrogel under NIR irradiation nearly closed. However, a large scar area can be found for the other groups due to serious bacterial infection.
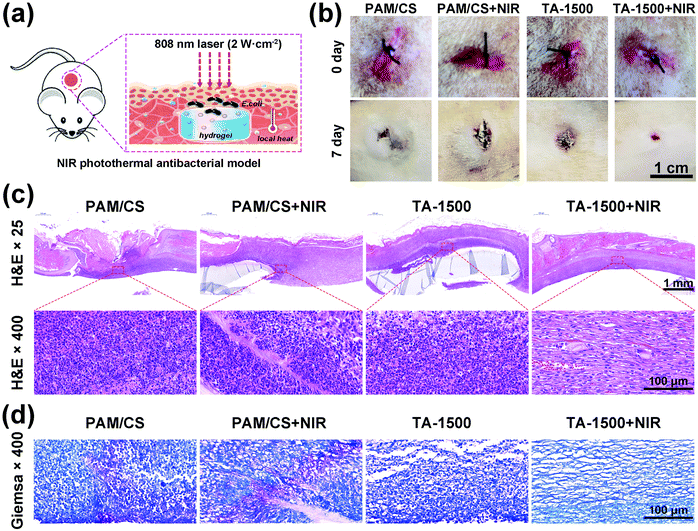 |
| Fig. 6 Antibacterial properties of the PAM/CS hydrogel and the TA-1500 hydrogel in vivo. (a) NIR photothermal antibacterial model in vivo. (b) Representative photos of the implant site after 0 and 7 days. (c) H&E staining of the skin tissues surrounding the implant hydrogels. (d) Giemsa staining of the skin tissues surrounding the implant hydrogels. | |
Following this, H&E staining was applied to investigate the tissue growth and inflammatory reactions in the defects. As shown in Fig. 6c, the epidermis and dermis were severely damaged in the PAM/CS hydrogel with/without NIR irradiation and the TA-1500 hydrogel group without NIR irradiation. Moreover, a large number of inflammatory cells can be observed due to the invasion of E. coli. In contrast, the number of inflammatory cells in the TA-1500 hydrogel with NIR irradiation is found to be significantly less compared to the other groups due to the photothermally antibacterial activity. Also, Giemsa staining was conducted to observe the remaining bacteria. As displayed in Fig. 6d, lots of bacteria (blue purple area) were found in the PAM/CS hydrogel group with and without NIR laser irradiation. Only a small number of bacteria were found in the TA-1500 hydrogel group. In contrast, there were no detectable signs of bacteria in the TA-1500 hydrogel with NIR laser irradiation group.
3.8. Skin wound healing in vivo
The representative photos of wound defects are shown in Fig. 7a. After implantation for 7 days, the TA-1500 hydrogel group had smaller wound defects than the blank and PAM/CS hydrogel groups. On the 15th day, the wound defect was almost closed in the TA-1500 hydrogel group, whereas visible scars were observed in the blank group and the PAM/CS hydrogel group. The wound closure rate was evaluated quantitatively by calculating the skin wound closure areas (Fig. 7b). After implantation for 7 days, the healing ratio for the TA-1500 hydrogel group was 67%, which was higher than those of the blank group (52%) and the PAM/CS hydrogel group (54%). The quantitative assessment of the wound closure ratio showed that there is no significant difference at day 15. However, H&E staining demonstrated that the quality of the regenerated skin tissue at the wound sites was obviously different. As shown in Fig. 7c, all wound defect sites had been covered by an intact and complete layer of epidermis after implantation for 15 days. Yet, the dimension of granulation tissue of the regenerated area in the TA-1500 hydrogel group (2150 μm) was significantly lower than those in the blank group (4650 μm) and the PAM/CS hydrogel group (4000 μm). In addition, many collagen fibers appeared in the regenerated area of the TA-1500 hydrogel group. Finally, as an important endogenous cell growth factor, EGF is good for the proliferation of epithelial cells and fibroblasts and collagen synthesis.54,55 By loading EGF in hydrogels, faster healing rates of the wounds could be obtained than the other groups. Additionally, the regeneration area of the EGF-loaded hydrogel group was basically completely covered by collagen fibers and hair follicles. These results indicate that the combination of EGF in the hydrogels can further promote wound healing and skin tissue regeneration and maturation.
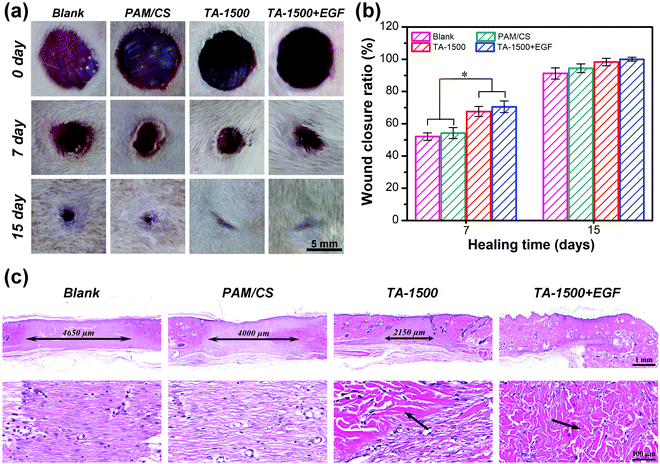 |
| Fig. 7 (a) Representative photos of gross appearance of defects treated with blank, PAM/CS hydrogel, TA-1500 hydrogel, and EGF loaded TA-1500 hydrogel at days 0, 7 and 15. (b) Percent wound closure area at 0, 7, and 15 days post wounding. (c) H&E staining of wound sections after 15 days of treatment (double arrow: granulation tissue; single arrow: collagen fibers). * indicates the significant difference (p < 0.05). | |
In short, the full-thickness skin defect repair test demonstrates that the TA-1500 hydrogels have excellent tissue affinity to promote skin tissue regeneration and accelerate tissue maturation, which might be ascribed to the following two aspects. First, the biocompatible TA-1500 hydrogel exhibits good cell affinity to promote attachment, spreading and proliferation of the fibroblast, which has been proven in Fig. 5, and can provide a suitable platform for cell migration and tissue regeneration. Many studies have indicated that fibroblast behavior, such as adhesion, proliferation and differentiation, is conducive to skin tissue regeneration and maturation.56,57 Second, the incorporation of biocompatible TA@Fe3+ can promote the differentiation of fibroblasts, granulation tissue reorganization and basement membrane regeneration, which has been demonstrated by qRT-PCR tests, to accelerate wound healing and tissue maturation. In addition, loading of the hydrogels with EGF can further increase the rate of skin tissue regeneration and wound closure. The release curve of EGF (Fig. S8, ESI†) demonstrates that the TA-1500 hydrogel can enable effective immobilization and sustain the release of growth factors. As a growth factor associated with skin regeneration, the release of EGF was conducive to the working of its biological function, including promoting skin tissue growth and accelerating wound closure.58,59
4. Conclusions
In summary, NIR photothermally responsive and adhesive hydrogels for accelerated skin wound healing have been fabricated by a one step in situ self-catalyzed reaction. TA@Fe3+ promote the self-catalyzed polymerization to form tough hydrogels, but also endow the hydrogels with repeated tissue adhesiveness. In addition, the high NIR photothermal conversion of TA@Fe3+ endows the hydrogels with good antibacterial properties without the need of utilizing antibiotics, which is demonstrated by antibacterial experiments both in vitro and in vivo. Moreover, TA@Fe3+ shows good cell affinity, which can promote cell attachment, proliferation and differentiation of fibroblasts to accelerate skin wound closure and tissue maturation. Consequently, the non-antibiotic and NIR photothermally responsive hydrogel with efficient tissue adhesion and cell affinity is a promising wound dressing for both skin wound disinfection and repair.
Conflicts of interest
The authors declare no conflict of interest.
Acknowledgements
The National Natural Science Foundation of China (No. 51703145 and 51673130) and the China Postdoctoral Science Foundation (2017M620426 and 2019T120844) are gratefully acknowledged for financial support. The authors would also like to thank the Center of Testing and Analysis of Sichuan University for SEM measurement, and Lingzhu Yu and Guolong Meng (Sichuan University) for EDS mapping.
References
- Y. Yu, P. Li, C. Zhu, N. Ning, S. Zhang and G. J. Vancso, Adv. Funct. Mater., 2019, 29, 1904402 CrossRef.
- X. Zhao, H. Wu, B. Guo, R. Dong, Y. Qiu and P. X. Ma, Biomaterials, 2017, 122, 34–47 CrossRef CAS.
- T. Mandana, M. L. Jose Gil, V. Amin, I. Md. Shahidul, K. Joseph, T. Nathalie and G. M. v. d. V. Theo, ACS Appl. Mater. Interfaces, 2020, 12, 39991–40001 CrossRef.
- J. L. Drury and D. J. Mooney, Biomaterials, 2003, 24, 4337–4351 CrossRef CAS.
- J. Kopeček, Biomaterials, 2007, 28, 5185–5192 CrossRef.
- E. Mir Morteza Sadat and S. Holger, Langmuir, 2014, 30, 7842–7850 CrossRef.
- N. T. Thet, D. R. Alves, J. E. Bean, S. Booth, J. Nzakizwanayo, A. E. R. Young, B. V. Jones and A. T. A. Jenkins, ACS Appl. Mater. Interfaces, 2016, 8, 14909–14919 CrossRef CAS.
- S. O. Blacklow, J. Li, B. R. Freedman, M. Zeidi, C. Chen and D. J. Mooney, Sci. Adv., 2019, 5, eaaw3963 CrossRef CAS.
- C. Tao, C. Yujie, R. Hafeez Ur, C. Zhen, Y. Zhi, W. Man, L. Hua and L. Hezhou, ACS Appl. Mater. Interfaces, 2018, 10, 33523–33531 CrossRef.
- S. Gopendra, N. Aradhana, M. Sahil and K. Veena, Carbohydr. Polym., 2020, 247, 116757 CrossRef.
- R. Wang, J. Li, W. Chen, T. Xu, S. Yun, Z. Xu, Z. Xu, T. Sato, B. Chi and H. Xu, Adv. Funct. Mater., 2017, 27, 1604894 CrossRef.
- M. Changez, V. Koul and A. K. Dinda, Biomaterials, 2005, 26, 2095–2104 CrossRef CAS.
- L. Shuqiang, D. Shujun, X. Weiguo, T. Shicheng, Y. Lesan, Z. Changwen, D. Jianxun and C. Xuesi, Adv. Sci., 2018, 5, 1700527 CrossRef.
- E. Mir-Morteza Sadat, V. Yvonne and S. Holger, ACS Appl. Mater. Interfaces, 2015, 7, 20190–20199 CrossRef.
- J. M. Blair, M. A. Webber, A. J. Baylay, D. O. Ogbolu and L. J. Piddock, Nat. Rev. Microbiol., 2015, 13, 42–51 CrossRef CAS.
- L. R. Hoffman, D. A. D'Argenio, M. J. MacCoss, Z. Zhang, R. A. Jones and S. I. Miller, Nature, 2005, 436, 1171–1175 CrossRef CAS.
- H. Dengfeng, Y. Yunlong, L. Fangqin, Y. Yongchao, L. Pengfei, C. Jiali, N. Ning and Z. Shiyong, Chem. Eng. J., 2019, 382, 122976 Search PubMed.
- Y. Yang, L. Ma, C. Cheng, Y. Deng, J. Huang, X. Fan, C. Nie, W. Zhao and C. Zhao, Adv. Funct. Mater., 2018, 28, 1705708 CrossRef.
- X. Li, H. Bai, Y. Yang, J. Yoon, S. Wang and X. Zhang, Adv. Mater., 2019, 31, 1805092 Search PubMed.
- Y. Zhao, Q. Guo, X. Dai, X. Wei, Y. Yu, X. Chen, C. Li, Z. Cao and X. Zhang, Adv. Mater., 2019, 31, 1806024 CrossRef.
- Z. Zhijun and S. Ming, Nanomaterials, 2017, 7, 160 CrossRef.
- Q. Gao, X. Zhang, W. Yin, D. Ma, C. Xie, L. Zheng, X. Dong, L. Mei, J. Yu and C. Wang, Small, 2018, 14, 1802290 CrossRef.
- H. Ma, C. Jiang, D. Zhai, Y. Luo, Y. Chen, F. Lv, Z. Yi, Y. Deng, J. Wang and J. Chang, Adv. Funct. Mater., 2016, 26, 1197–1208 CrossRef CAS.
- Q. Zou, M. Abbas, L. Zhao, S. Li, G. Shen and X. Yan, J. Am. Chem. Soc., 2017, 139, 1921–1927 CrossRef CAS.
- Z. Xin, L. Yongping, G. Baolin, Y. Zhanhai, Z. Dun and H. Yong, Chem. Eng. J., 2021, 403, 126329 CrossRef.
- T. Liu, M. Zhang, W. Liu, X. Zeng, X. Song, X. Yang, X. Zhang and J. Feng, ACS Nano, 2018, 12, 3917–3927 CrossRef CAS.
- J. Zeng, M. Cheng, Y. Wang, L. Wen, L. Chen, Z. Li, Y. Wu, M. Gao and Z. Chai, Adv. Healthcare Mater., 2016, 5, 772–780 CrossRef CAS.
- V. Natarajan, N. Krithica, B. Madhan and P. K. Sehgal, J. Biomed. Mater. Res., Part B, 2013, 101, 560–567 CrossRef.
- N. Ninan, A. Forget, V. P. Shastri, N. H. Voelcker and A. Blencowe, ACS Appl. Mater. Interfaces, 2016, 8, 28511–28521 CrossRef CAS.
- Y. Liu, K. Ai and L. Lu, Chem. Rev., 2014, 114, 5057–5115 CrossRef CAS.
- M. V. Rapp, G. P. Maier, H. A. Dobbs, N. J. Higdon, J. H. Waite, A. Butler and J. N. Israelachvili, J. Am. Chem. Soc., 2016, 138, 9013–9016 CrossRef CAS.
- S. Zhao, S. Xie, Z. Zhao, J. Zhang, L. Li and Z. Xin, ACS Sustainable Chem. Eng., 2018, 6, 7652–7661 CrossRef CAS.
- P. Lin, S. Ma, X. Wang and F. Zhou, Adv. Mater., 2015, 27, 2054–2059 CrossRef CAS.
- J. Yang, M. Li, Y. Wang, H. Wu, T. Zhen, L. Xiong and Q. Sun, Biomacromolecules, 2019, 20, 801–812 CrossRef CAS.
- Z. Jia, Y. Zeng, P. Tang, D. Gan, W. Xing, Y. Hou, K. Wang, C. Xie and X. Lu, Chem. Mater., 2019, 31, 5625–5632 CrossRef CAS.
- L. Wei, L. Guoguang, L. Ya, H. Yafeng, P. Huizhuo, W. Hanjie, N. Baoan, X. Hang and H. Xian, Adv. Mater., 2018, 30, 1800917 CrossRef.
- C. Mao, Y. Xiang, X. Liu, Z. Cui, X. Yang, Z. Li, S. Zhu, Y. Zheng, K. W. K. Yeung and S. Wu, ACS Nano, 2018, 12, 1747–1759 CrossRef CAS.
- Q. Li, F. Lu, G. Zhou, K. Yu, B. Lu, Y. Xiao, F. Dai, D. Wu and G. Lan, Biomacromolecules, 2017, 18, 3766–3775 CrossRef CAS.
- H. Aydin, A. El Haj, E. Pişkin and Y. Yang, Tissue Eng. Regener. Med., 2009, 3, 470–476 CrossRef CAS.
- P. Li, Z. Jia, Q. Wang, P. Tang, M. Wang, K. Wang, J. Fang, C. Zhao, F. Ren and X. Ge, J. Mater. Chem. B, 2018, 6, 7427–7438 RSC.
- P. Matricardi, C. Di Meo, T. Coviello, W. E. Hennink and F. Alhaique, Adv. Drug Delivery Rev., 2013, 65, 1172–1187 CrossRef CAS.
- P. L. Bruce, P. B. Messersmith, J. N. Israelachvili and J. H. Waite, Annu. Rev. Mater. Res., 2011, 41, 99–132 CrossRef.
- H. Lu, Y. Liwei, W. Menghao, W. Kefeng, F. Liming, Z. Jie, F. Ju, R. Fuzeng and L. Xiong, Chem. Mater., 2018, 30, 5561–5572 CrossRef.
- S. Changyou, W. Meng, M. Lei, C. Huanliang, W. Bo, X. Feng, Y. Jun and W. Pengbo, Chem. Mater., 2018, 30, 3110–3121 CrossRef.
- M. Zhong, Y. T. Liu and X. M. Xie, J. Mater. Chem. B, 2015, 3, 4001–4008 RSC.
- A. Anitha, S. Sowmya, P. S. Kumar, S. Deepthi, K. Chennazhi, H. Ehrlich, M. Tsurkan and R. Jayakumar, Prog. Polym. Sci., 2014, 39, 1644–1667 CrossRef CAS.
- J. Luo, J. Lai, N. Zhang, Y. Liu, R. Liu and X. Liu, ACS Sustainable Chem. Eng., 2016, 4, 1404–1413 CrossRef CAS.
- Y. Yang, P. He, Y. Wang, H. Bai, S. Wang, J. F. Xu and X. Zhang, Angew. Chem., Int. Ed., 2017, 56, 16239–16242 CrossRef CAS.
- M. Lutolf and J. Hubbell, Nat. Biotechnol., 2005, 23, 47 CrossRef CAS.
- F. M. Watt and W. T. Huck, Nat. Rev. Mol. Cell Biol., 2013, 14, 467–473 CrossRef CAS.
- L. Jiun, H. Jiyoung, K. WonJin and K. Geun Hyung, Carbohydr. Polym., 2020, 250, 116914 CrossRef.
- H. Ceylan, A. B. Tekinay and M. O. Guler, Biomaterials, 2011, 32, 8797–8805 CrossRef CAS.
- S. Zhang, K. Xu, M. A. Darabi, Q. Yuan and M. Xing, Mater. Sci. Eng., C, 2016, 69, 496–504 CrossRef CAS.
- M. Goh, Y. Hwang and G. Tae, Carbohydr. Polym., 2016, 147, 251–260 CrossRef CAS.
- G. Carpenter and S. Cohen, Annu. Rev. Biochem., 1979, 48, 193–216 CrossRef CAS.
- H. Yu, J. Peng, Y. Xu, J. Chang and H. Li, ACS Appl. Mater. Interfaces, 2015, 8, 703–715 CrossRef.
- R. R. Driskell and F. M. Watt, Trends Cell Biol., 2015, 25, 92–99 CrossRef CAS.
- Z. Su, H. Ma, Z. Wu, H. Zeng, Z. Li, Y. Wang, G. Liu, B. Xu, Y. Lin and P. Zhang, Mater. Sci. Eng., C, 2014, 44, 440–448 CrossRef CAS.
- H. J. Lai, C. H. Kuan, H. C. Wu, J. C. Tsai, T. M. Chen, D. J. Hsieh and T. W. Wang, Acta Biomater., 2014, 10, 4156–4166 CrossRef CAS.
Footnote |
† Electronic supplementary information (ESI) available. See DOI: 10.1039/d0tb02160a |
|
This journal is © The Royal Society of Chemistry 2021 |
Click here to see how this site uses Cookies. View our privacy policy here.