Tough thermoplastic hydrogels with re-processability and recyclability for strain sensors†
Received
24th August 2020
, Accepted 13th November 2020
First published on 16th November 2020
Abstract
Tough hydrogels with the ability to be repeatedly processed into various shapes as thermoplastics are highly desired in advanced medical devices and tissue engineering. Here, we have developed a kind of versatile supramolecular hydrogel with a network cross-linked by double hydrogen bonds from poly(N-acryloyl glycinamide) (PNAGA). The resulting PNAGA-30 hydrogels (30 wt% solid content) are tough, re-processable, and recyclable similar to thermoplastics. The hydrogels in the form of fragments can be easily re-processed into various shapes including sheet, filament, cylinder and other complex shapes by using simple stamping and injection methods. The mechanical properties of the re-programed hydrogels are comparable to the properties of the original hydrogels. The re-processability and robust mechanical properties of the PNAGA hydrogels are promising for practical applications in soft materials, tissue engineering and wearable devices. Furthermore, the PNAGA-30&LiCl ionic hydrogels can be fabricated by simply compositing LiCl into thermoplastic hydrogels. The PNAGA-30&LiCl hydrogels can function as multifunctional strain sensors to monitor large human movements and tiny vibrations, thereby showing great application potential in robotics, biomedical prosthetics, personal healthcare monitoring and so on.
1. Introduction
Hydrogels, as soft and wet materials with high water content and tunable properties, are playing an irreplaceable role in “real world” applications.1–7 For a majority of given applications, hydrogels are expected to exhibit an integration of properties embracing toughness, processability, and self-healing.8,9 Recently, hydrogels with arbitrarily designed shapes and high strength have been widely applied in many advanced fields. For instance, flexible wearable sensors require tough hydrogel sheets that can be easily fabricated into sandwich-like or other skin-adapted structures.10,11 Injectable and tough hydrogels can meet the personalized demands of tissue engineering such as cartilage or hard bone substitutes and intervertebral disc repair.12–14 Tubular hydrogels with high mechanical strength are highly desired for vascular repair.15,16 In particular, spring-like tough hydrogels exactly coincide with the needs of hemangioma treatments, because they can readily fill and compact the tumor.17 Therefore, constructing tough hydrogels with easy forming and processing features will be of great significance for their potential applications including flexible sensors, soft machine, tissue engineering and disease treatments. To date, a large number of studies have been proposed to build high-strength hydrogels, which are mainly derived from chemically crosslinked hydrogels such as classic double-network hydrogels,18,19 nanoparticle-crosslinked hydrogels,20,21 sliding-ring hydrogels,22 microcrystal-crosslinked hydrogels,23,24 and physically cross-linked hydrogels driven by hydrophobic association,25 hydrogen bonding,26,27 ionic bonding,28etc. However, due to the natural weakness, the structural integrity of most hydrogels still has the risk of collapsing. In addition, it is also difficult to remodel their shapes once they are cast in the given molds during the gelation process. These issues will largely limit their further applications.
As we all know, thermoplastics have excellent thermo-plasticity and re-processable characteristics, and can be repeatedly processed into any desired shape to meet numerous practical needs. Generally, the manufacturing process of thermoplastic products consists of pelleting and molding processes, which is beneficial to their storage, transport and mass production. However, so far few studies have been focused on tough hydrogels with repeated processing and shaping capacities similar to thermoplastics. In principle, physical networks cross-linked by reversible bonds including hydrogen bonds, ionic bonds and hydrophobic interactions may endow hydrogels with re-processability. Thus, intensive attempts have been made to fabricate re-processable and tough hydrogels based on this strategy. For example, Gong et al. reported a tough polyion complex hydrogel that can be reprocessed into the desired shapes by dissolving it in concentrated sodium chloride solution, followed by regenerating from water.29 Yang et al. fabricated an ultra-strong and tough supramolecular hydrogel that can be tailored arbitrarily using a dissolving–regenerating procedure in good and poor solvents.26 A metal-coordination complex mediated physical hydrogel has been discussed by Zheng's group,30 which features good re-processability and high toughness. Although the utilization of salt, acid, base, or organic solvents can realize the re-processability of hydrogels, this will inevitably cause undesired waste and environmental burden. To overcome this problem, many thermoplastic hydrogels that can be reprocessed by most existing plastic processing methods including extrusion, injection, and compression moldings have been developed. However, the shortcomings, such as poor mechanical properties, non-transparency and the absence of restoration after dehydration, significantly limit their practical applications.31–34 Therefore, the hydrogels with re-processability, toughness, and restoration after dehydration that can be manufactured more similar to thermoplastics are fully in demand.
It has been reported that N-acryloyl glycinamide can directly polymerize in water under UV light or heat, and form strong and thermoreversible hydrogen bonds between the dual amide motifs.27 Herein, thermoplastic and tough poly(N-acryloyl glycinamide) (PNAGA) hydrogels were developed through the strong hydrogen-bonding interactions from dual amide motifs on PNAGA macromolecules. The resulting PNAGA-30 hydrogels (30 wt% solid content) could be processed by a two-step process including a pelleting and molding process similar to thermoplastics, and this process could be repeated many times without damaging the mechanical properties. Thus, it was possible to remodel the PNAGA-30 hydrogels into various desired shapes, such as thin sheets, lumps (heart, pentagram, letter, and cylinder) and filiforms. In addition, the hydrogels also integrated extreme stretchability (nearly 1000%), high strength (1.23 MPa) and the ability to restore in water. Furthermore, the PNAGA-30 hydrogels containing lithium chloride (LiCl) were also investigated. LiCl was introduced to transmit the electrical signal without disturbing the inherent features of the PNAGA-30 hydrogels. As a result, multifunctional PNAGA-30&LiCl hydrogels with excellent recyclability, self-healing ability, toughness, transparency and stable electrical performance were obtained and adopted as flexible strain sensors. The PNAGA-30&LiCl hydrogel-based strain sensors could detect large human movements (fingers, elbow, and knee) and tiny vibrations such as pulse shock, which makes them very attractive for applications in soft robotics, biomedical prosthetics, and personal healthcare monitoring. Naturally, this concept of hybridization can be further extended by compositing thermoplastic-like PNAGA-30 hydrogels with more functional materials to meet diverse requirements in practical applications.
2. Experimental section
2.1 Materials
Acryloyl chloride (96%), glycinamide hydrochloride (98%), anhydrous potassium carbonate (K2CO3, 99%) and photoinitiator 1173 (97%) were purchased from Aladdin Industrial Co., Ltd (Shanghai, China). Anhydrous lithium chloride (LiCl, 99%) was obtained from Macklin Biochemical Technology Co., Ltd (Shanghai, China). Anhydrous diethyl ether, ethanol, and methanol were purchased from Guangshi Agent Technology Co., Ltd (Guangdong, China).
2.2 Preparation of N-acryloyl glycinamide (NAGA)
NAGA was synthesized according to the method reported by Liu's work with a minor modification.27 Briefly, glycinamide hydrochloride (31.5 g), K2CO3 aqueous solution (198 mL, 1.7 M) and cold diethyl ether (180 mL) were added to a 500 mL three-necked flask, and the mixture was placed in an ice bath. Subsequently, acryloyl chloride (25.6 mL) dissolved in 120 mL of cold diethyl ether was added dropwise within 5 h under continuous stirring at 0 °C. The mixed solution was reacted at room temperature for another 4 h and then its pH was adjusted to 2 with HCl aqueous solution (6 M). After separation of the organic phase, the aqueous phase was further washed with diethyl ether (250 mL × 3) and then adjusted to neutral pH with NaOH aqueous solution (2 M). After removal of the solvent, the solid residue was extracted with ethanol/methanol mixture (4/1, v/v, 250 mL × 3). The combined filtrate was concentrated under vacuum and the resultant mixture was recrystallized at 0 °C to obtain the final product NAGA (25.4 g, yield: 63.0%). The chemical structure of NAGA was confirmed by Fourier transform infrared (FTIR) spectroscopy and 1H and 13C nuclear magnetic resonance (NMR) analyses. FTIR (KBr, cm−1): 3387 (m, NH), 3311 (s, NH), 3189 (m, NH), 1661 (vs, C
O), 1626 cm−1 (vs, C
O), 1558 (vs, NH). 1H NMR (D2O): δ (ppm) 3.9 (2H, –NH–CH2−), 5.7 (2H, CH2
CH–), 6.1 (1H, CH2
CH–). 13C NMR (DMSO-d6): δ (ppm) 42.4 (–NH–CH2−), 126.0 (CH2
CH–), 132.3, (CH2
CH–), 165.5 (–CO–NH–), 171.5 (–CO–NH2).
2.3 Preparation of PNAGA and PNAGA-30&LiCl hydrogels
NAGA solution was first prepared by dissolving the NAGA monomer in DI water. Then, the photo-initiator 1173 (2 wt%, relative to the mass of the NAGA monomer) was added into the above solution and fully stirred under a nitrogen atmosphere. The obtained mixture was poured into the molds with a size of 6 cm × 6 cm ×1 mm or 6 cm × 6 cm ×2 mm. The molds were sealed by a glass plate and transferred into a UV curing machine (Intelli-Ray 600, Uvitron, USA) to expose them for 40 min. The resultant PNAGA hydrogels were washed thoroughly with DI water to remove the impurities, and then stored in DI water. Similarly, PNAGA-30&LiCl hydrogels with different LiCl contents (from 0.4 to 2.0 wt%, indicative of the LiCl concentration of the solvent) were prepared using the same procedure, except that the DI water was replaced by LiCl aqueous solutions of different concentrations. Finally, the obtained hydrogels were washed with LiCl solution with a corresponding concentration to remove the impurities, and then stored in such solution at room temperature for further study.
2.4 Characterization
FTIR spectra were recorded on an MAGN-IR760 FTIR spectrometer (Nicolet, USA) by using the potassium bromide pellet method. 1H and 13C NMR spectra were recorded on a Bruker AVANCE III HD 600 MHz spectrometer (Bruker, UK). Chemical shifts were referenced to the solvent values (δH = 4.79 ppm for D2O, δC = 39.52 ppm for DMSO-d6). The UV-vis absorption spectrum of the hydrogel was recorded on a TU-1901 UV-vis spectrophotometer (Beijing General Analysis General Instrument Co., Ltd, China) with a wavelength-scanning range from 400 to 800 nm at a scanning rate of 100 nm min−1. The sol–gel transition of the PNAGA-30 hydrogels was characterized using a AR 200 rheometer (TA Instrument, USA). The PNAGA-30 hydrogel disk (25 mm in diameter and 1 mm in height) was placed under a parallel plate of 25 mm diameter. Silicone oil was covered on the edge of the hydrogel disk to avoid evaporation of water. Temperature sweep upon a heating–cooling cycle (25–100 °C) with a temperature change rate of 5 °C min−1 was performed at a constant frequency of 1 Hz and a strain of 1.0%. The water content (WC) of the hydrogels was measured by the gravimetric method and calculated with the following formula:
where mwet and mdry are the wet and dry weights of the hydrogels, respectively.
2.5 Thermoplastic experiment
The PNAGA-30 hydrogel sheet was cut into fragments, and then filled into the syringe, square, and letter molds, respectively, followed by transfer into a high-pressure reactor. The sealed high-pressure reactor was heated at 110 °C for 1 h, and then withdrawn from the oven to cool slowly. In particular, a filiform hydrogel was obtained by injecting the heated fragments from the syringe, whereas a cylindrical hydrogel was formed by directly cooling the heated fragments in the syringe. The resultant lamella, alphabetic and cylindrical hydrogels were stored in DI water and re-swelled to equilibrium. Furthermore, the thermoplastic behavior of the hydrogels after drying was also evaluated. The PNAGA-30 hydrogel fragments were fully dried in an oven at 60 °C, and then immersed in DI water for 2 days. The swelled hydrogel fragments were separately filled into the pentagram, heart and fish-shaped molds and then kept in a sealed high-pressure reactor at 110 °C for 1 h. After cooling to room temperature, the obtained hydrogels were re-swelled to equilibrium in DI water.
2.6 Reprocessable experiment
The PNAGA-30 hydrogel sheet was cut into fragments and filled into molds. Subsequently, they were placed into a sealed high-pressure reactor and heated at 110 °C for 1 h, and then cooled to room temperature. The breaking and reshaping process of the hydrogels was repeated 4 times. In every breaking–reshaping cycle, the reshaped hydrogels were immersed in DI water to reach swelling equilibrium, and the corresponding WC was evaluated.
2.7 Recycling experiment
The recycling ability of the PNAGA-30 hydrogels after being dried was evaluated. A PNAGA-30 hydrogel sheet was placed in an oven at 60 °C until it completely dried. And then the dry hydrogel was immersed in DI water to fully soak. The weight and size of the hydrogels before and after drying were recorded. Also, the PNAGA-30 hydrogel fragments were fully dried in an oven at 60 °C, and then immersed in DI water for 2 days. The swelled hydrogel fragments were separately filled into the pentagram, heart and fish-shaped molds and then kept in a sealed high-pressure reactor at 110 °C for 1 h. After cooling to room temperature, the obtained hydrogels were re-swelled to equilibrium in DI water.
2.8 Mechanical test
The mechanical properties of the hydrogels were characterized by tensile tests, which were performed on an INSTRON 5967 universal testing machine (Instron Corporation, UK) at 25 °C. The hydrogels were tailored to dumbbell-shaped specimens with an effective gauge size of 10 mm (length) × 4 mm (width) × 2.5 mm (thickness). During uniaxial tensile (to fracture) and cyclic tensile tests, the applied strain rates were set as 50 and 100 mm min−1, respectively.
2.9 Self-healing experiment
To test the self-healing ability, the PNAGA-30&LiCl hydrogels with a size of 6 cm (length) × 1.5 cm (width) × 2 mm (thickness) were cut into two parts and one of them was dyed using methylene blue. Then, the two different colored hydrogel parts were put together in the original mold along the cut surface, and transferred into an oil bath at 90 °C for 3 h. Finally, the self-healed hydrogels were put back into the LiCl solution (1.2 wt%) and soaked for 2 days.
2.10 Fabrication of strain sensors
The PNAGA-30&LiCl hydrogels were cut into strip-shaped strain sensors with a size of 6 cm × 6 cm × 1 mm and then stored in 1.2 wt% LiCl solution. For the conductivity test, the strip-shaped hydrogel sensors were taken out and the surface water was removed using filter paper. Both ends of the hydrogel sensors were connected to the electrodes. To monitor human movements, the strip-like hydrogel sensors were fixed tightly to the fingers or other testing parts with double-sided adhesive tapes (VHB 4914, 3M).
2.11 Electrical measurement
The resistance of the PNAGA-30&LiCl hydrogel-based strain sensors was tested using a DC resistance tester (UC2517, Shenzhen Tongce Electronic Instrument Co., Ltd, China). The relative resistance change was calculated according to the following formula:
where R0 and RS represent the initial and instantaneous resistance, respectively.
3. Results and discussion
3.1 Fabrication of PNAGA hydrogels
To design and fabricate thermoplastic and robust hydrogels, the NAGA monomer was synthesized and subjected to polymerization under UV-light irradiation. Thanks to the strong intermolecular hydrogen bonding among the NAGA moieties, the PNAGA macromolecules could form supramolecular hydrogels in situ from their solutions, as depicted in Fig. 1a, and their chemical structure was further confirmed by the FTIR spectrum (Fig. S1, ESI†). The equilibrated water contents (EWC) of the PNAGA hydrogels, after being stored in DI water, with different monomer concentrations showed a slight increase compared with the initial values (Fig. S2, ESI†). As expected, the mechanical properties of the resultant hydrogels were significantly controlled by varying the solid content (Fig. 1b). The tensile strength of the hydrogels always increased with an increase of solid content, whereas the elongation of the hydrogels first increased upon increasing the initial solid content from 20 to 30%, and then decreased slightly at an initial solid content of 35%, possibly because the excessive cross-linking density impaired the elasticity of the hydrogels. Therefore, the PNAGA-30 hydrogels with the initial and equilibrated solid contents of 30 and 28.3%, respectively, were selected for further discussion. To verify whether the dynamic double hydrogen bonds could endow the PNAGA-30 hydrogels with good thermoplasticity, the hydrogel fragments were heated in a 100 °C-oil bath at atmospheric pressure for 1 h. Unfortunately, the hydrogel fragments were hard to merge into an integral hydrogel, and appeared to shrink because of losing water (Fig. S3, ESI†). This is probably because the enhanced intermolecular hydrogen bonds derived from the double amide motifs result in a higher melting point of PNAGA. To address this problem, a remodeling process of the PNAGA-30 hydrogels was conducted in a high-pressure reactor at 110 °C. It was easy to heat the water to 110 °C without boiling under an increased pressure, which facilitated the destruction of hydrogen bonds. Based on this method, the hydrogel fragments were successfully remolded into various shapes such as lamella, alphabetic, cylindrical and filiform (Fig. 1c). To evaluate the transition process of the PNAGA-30 hydrogels, the storage modulus (G′) of the hydrogels during the heating and cooling cycle was recorded by a rheological test. As shown in Fig. 1d, the G′ of the PNAGA-30 hydrogels decreased from 165.1 to 25.8 kPa with the increasing temperature from 25 to 100 °C, as a result of the disruption of the hydrogen-bonding networks. Moreover, the G′ of the hydrogels recovered to ∼90% of the original value when the temperature decreased to 25 °C, demonstrating the rapid recovery of the hydrogel networks at room temperature. Taken together, these results indicated that the PNAGA-30 hydrogels could be easily destructed and regenerated through the breakage and reformation of dynamic hydrogen bonds under heating and cooling.
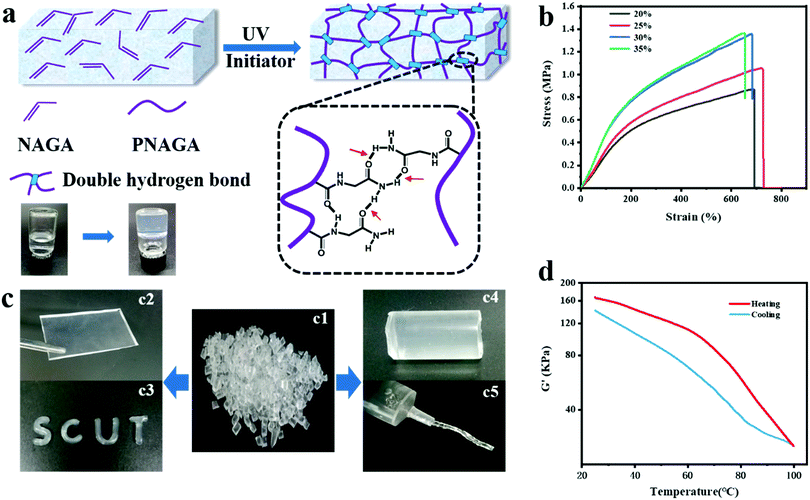 |
| Fig. 1 (a) Schematic illustration for the formation of PNAGA hydrogels via the double hydrogen bonds. (b) Tensile-testing curves of the PNAGA hydrogels with various solid contents. (c) Reprocessing capability of PNAGA hydrogels; (c1) PNAGA-30 hydrogel fragments were shaped into (c2) sheet, (c3) letters “SCUT”, (c4) cylinder, and (c5) strip. (d) G′ variation of PNAGA-30 hydrogels during a heating and cooling cycle. | |
3.2 Re-processability of PNAGA-30 hydrogels
Analogous to thermoplastics, the PNAGA-30 hydrogels may be capable of processing repeatedly, which can largely reduce the economic cost and environmental burden. To evaluate the re-processability of the PNAGA-30 hydrogels, a circularly breaking–reshaping experiment was performed. In every cycle, the PNAGA-30 hydrogel sheet was cut into fragments, filled into a mold with a specific shape, and then heated at 110 °C for 1 h. Even the PNAGA-30 hydrogel sheet underwent four pelleting–reshaping cycles, and it could still be shaped into a beautifully shaped hydrogel without losing its thermoplasticity (Fig. 2a). Thus, the PNAGA-30 hydrogels can be re-processed and reused through a two-step process inclusive of pelleting and thermoforming. Meanwhile, the mechanical properties of the PNAGA-30 hydrogels during the breaking–reshaping cycles were evaluated. As shown in Fig. 2b, the original hydrogels exhibited a tensile strength up to 1.36 MPa, demonstrating the good mechanical properties of the PNAGA-30 hydrogels. With an increase of the breaking–reshaping cycle number, the tensile strength of the reshaped hydrogels showed a slight decrease. After four breaking–reshaping cycles, the tensile strength of the reshaped hydrogels could still reach 85% of the original hydrogels, revealing the good recovery ability of the hydrogels. Additionally, the water contents of the reshaped hydrogels during the breaking–reshaping cycles were also investigated, and there was no obvious change in the water content between the reshaped and original hydrogels (Fig. 2c). Therefore, it can be concluded that the PNAGA-30 hydrogels are a kind of reliable thermoplastic hydrogel, and can be repeatedly processed into different shapes without changing their intrinsic characteristics.
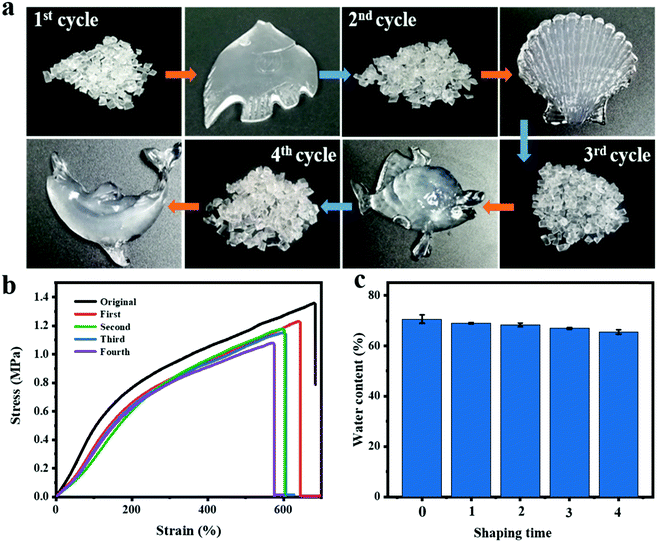 |
| Fig. 2 Re-processability of the hydrogels. (a) Breaking–reshaping cycle of the PNAGA-30 hydrogels could be repeated for four times by simple heating and cooling methods. (b) Tensile-testing curves of the PNAGA-30 hydrogels after shaping for various times. (c) Water contents of the reshaped PNAGA-30 hydrogels after every breaking–reshaping cycle. | |
3.3 Reversible drying–swelling process of PNAGA-30 hydrogels
For conventional hydrogels, the large amount of water within the networks and the lack of thermoplasticity make them inconvenient to transport, store and process them as thermoplastics. Therefore, the ability to recover from the dry state by simple rehydration becomes another necessary property of the hydrogels, which can largely extend their applications in an advanced manufacturing field. Accordingly, the ability of PNAGA-30 hydrogels to restore their original state in water after dehydration was evaluated. As shown in Fig. 3a, after being dried in an oven, the PNAGA-30 hydrogel sheet lost all of the water within it, resulting in a dried-gel sheet with a bent shape and a reduced size. After re-swelling in water, the dried gel sheet could easily recover to its original form. There was no obvious difference in the weight between the re-swelled and original PNAGA-30 hydrogels (Fig. S4, ESI†), demonstrating the good restoration of the PNAGA-30 hydrogels in water after drying. Furthermore, the thermoplasticity of PNAGA-30 hydrogels after restoration was also evaluated. As shown in Fig. 3b, the freshly prepared hydrogel fragments (Fig. 3b1) converted into dried gel pellets (Fig. 3b2) after completely drying at 60 °C, which could be stored and transported conveniently and were easily restored to their initial state by simply soaking in water (Fig. 3b3) for further molding. Subsequently, the re-swelled hydrogel fragments were put into the molds with desired shapes and underwent a melting and cooling process. As a result, integral and perfect hydrogels with pentagram, heart, and fish shapes were generated (Fig. 3c), demonstrating that the PNAGA-30 hydrogels still maintained their thermoplasticity after the drying and re-swelling processes.
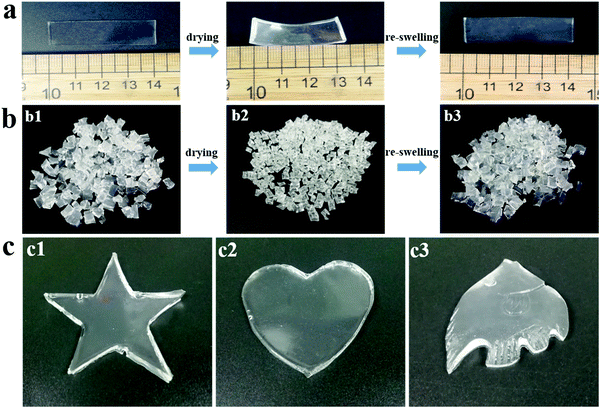 |
| Fig. 3 (a) Recoverability of the dried PNAGA-30 hydrogel sheet in water. (b) Recoverability of the dried PNAGA-30 hydrogel fragments in water; photographs of (b1) the original, (b2) dried, and (b3) re-swelled fragments. (c) Photographs of the (c1) pentagram, (c2) heart, and (c3) fish-shaped PNAGA-30 hydrogels reshaped from the re-swelled hydrogel fragments. | |
3.4 PNAGA-30&LiCl hydrogels as strain sensors
Nowadays, LiCl has been widely adopted to improve the conductivity of hydrogels, because Li+ is the smallest metallic ion with high stability under various conditions.35 Thus, PNAGA-30&LiCl ionic hydrogels were prepared by incorporating different amounts of LiCl into the PNAGA-30 hydrogels. First of all, the mechanical and electrical properties of the PNAGA-30&LiCl hydrogels with different LiCl contents were investigated. As shown in Fig. 4a and b, the conductivity of the hydrogels continually increased with an increase of LiCl content from 0.4 to 2.0 wt%, while the tensile strength and elongation of the hydrogels began to decrease when the LiCl content was higher than 1.2 wt%. Besides, at an LiCl content of 1.2 wt%, the PNAGA-30&LiCl hydrogels showed an EWC similar to the pure PNAGA-30 hydrogels, and exhibited good transmittance (>90%) (Fig. S5, ESI†). Therefore, the PNAGA-30&1.2 wt%LiCl hydrogels with superior comprehensive performance were selected for further study. The recovery of PNAGA-30&LiCl hydrogels was sequentially measured by continuous ten tension and relaxation cycles at strains varying from 100% to 700% (Fig. S6, ESI†). For the results in all test strains, the first stress–strain cycle showed an obvious hysteresis loop, which was probably caused by a distinct and efficient energy dissipation mechanism built on hydrogen bonds.1,6,7 However, the subsequential curves of circulation were nearly superimposable and exhibited no hysteresis loop, indicating the strong and dynamical cross-linking construction. Then, the PNAGA-30&LiCl hydrogel-based strain sensors were stretched using single cyclic uniaxial force at strains varing from 100 to 700%. In all of the seven cycles, the hydrogel sensors showed a smooth relative resistance change consistent with the strain (Fig. 4c). As one of the most crucial parameters during practical operation, the cyclic stability of the hydrogel sensors was further evaluated with a strain of 100% (Fig. 4d). The response to resistance could be maintained after 100 bending cycles, indicating the long working life and good reliability of the PNAGA-30&LiCl hydrogel-based strain sensors. Despite certain resistance deviation after excess bending cycles, the relative resistance change profiles were uniformly similar, suggesting the acceptable durability of the hydrogel sensors in practical application.
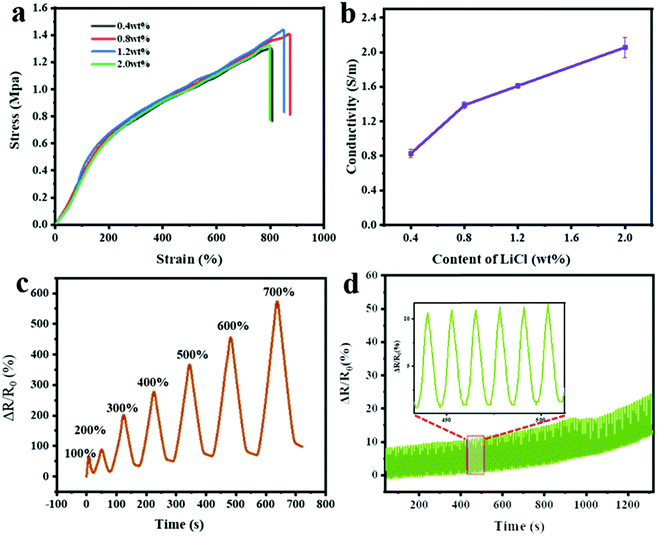 |
| Fig. 4 (a) Tensile-testing curves of the PNAGA-30&LiCl hydrogels with various LiCl contents. (b) Electrical conductivities of the PNAGA-30&LiCl hydrogels with various LiCl contents. (c) Time-dependent resistance change of the sensors during a loading–relaxing cycle at strains varing from 100 to 700%. (d) Time-dependent relative resistance changes with 100 cycles at a strain of 100% (the inset is the amplification of part of the curves). | |
Furthermore, the relationship between the relative resistance change and the strain of the PNAGA-30&LiCl hydrogel-based strain sensors was investigated in detail. As shown in Fig. 5a, the hydrogel sensors were stretched at strains changing from 0% to 100% and then from 100% to 0%. During the step-by-step loading and unloading cycle, the relative resistance change of the PNAGA-30&LiCl hydrogel-based strain sensors showed a clear step-like trend in accordance with strain (Fig. 5a1). It was noted that when a certain elongation was loaded and unloaded, the relative resistance changed immediately, proving the ultra-fast response capability of the hydrogel sensors. From the strain-relative resistance change fitting curve (Fig. 5a2), it could be found that the optimization degree of the fitted curve reached 0.996, indicating the good linear relationship between the relative resistance change and the strain (from 0 to 100%) for the hydrogel sensors. Moreover, the hydrogel sensors were fixed on the index finger and connected to conductive electrodes to monitor the joint motions (Fig. 5b). When bending the index finger at an angle from 0° to 90° step-by-step, the relative resistance change of the hydrogel sensors promptly increased to the corresponding values (Fig. 5b1). Furthermore, the relationship between the bending angle and the relative resistance change was determined by a linear fitting curve. Fig. 5b2 shows that the fitting curve has an optimization degree of 0.999, indicating an excellent linear agreement between the bending angle and the relative resistance change of the hydrogel sensors. It was worth pointing out that the above-mentioned real-time curves of the relative resistance change included background noise, which was possibly attributed to the slight vibration during the testing process, further demonstrating the high sensitivity of the PNAGA-30&LiCl hydrogel-based strain sensors.
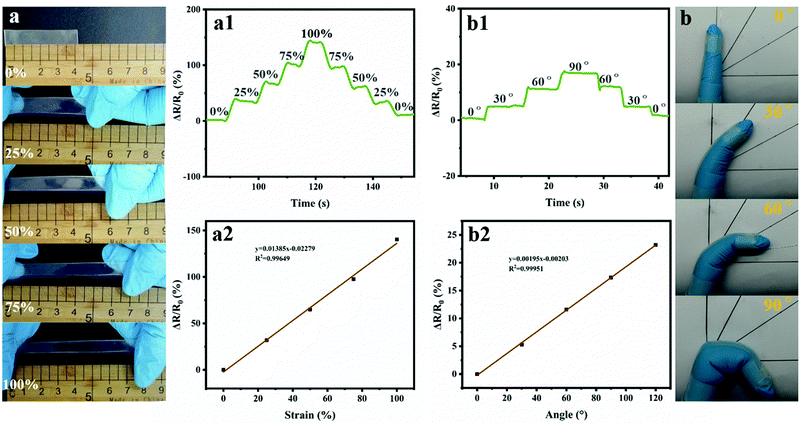 |
| Fig. 5 Relative resistance changes of PNAGA-30&LiCl hydrogel-based strain sensors upon varying the strain and the bending angle. (a) Photographs of the sensors under a continuous strain change from 0% to 100%; (a1) the relative resistance changes of the sensors relative to time during a loading–unloading cycle with different strains; (a2) the variation in relative resistance changes and fixed tensile strain. (b) Photographs of the bending finger at different bending angles (sensors were fixed on index finger joints); (b1) the relative resistance changes of the sensors relative to time during a bending–unbending cycle with different angles; (b2) the variation in relative resistance changes and the fixed bending angle. | |
3.5 Excellent healing ability of the PNAGA-30&LiCl hydrogel-based strain sensors
Owing to the reversible breakage and re-formation of the dynamic hydrogen bonds at high and room temperature, respectively, the PNAGA-30&LiCl hydrogels may present self-healing ability. To demonstrate this, two PNAGA-30&LiCl hydrogel sheets with different colors were cut into two parts. Subsequently, the two different colored halves were contacted along the cut surface within a mold and transferred into a 90 °C water bath for heating for 3 h. After cooling to room temperature and demolding, they were healed into an integral hydrogel sheet with an indistinguishable interface. Similar to the original hydrogel sheet, the healed hydrogel sheet could also withstand 500 g weight without breaking, showing the superior self-healing performance of the hydrogels (Fig. 6a). Moreover, as shown in Fig. 6b, the tensile strength and conductivity of the healed hydrogels separately recovered to 97% and 96% of the original hydrogels, indicating the high self-healing efficiency upon reversible breakage and the re-formation mechanism of hydrogen bonds. When serving as a strain sensor, it is very important to ensure the reliability of response signals before and after healing, which drives high requirements for the stability of hydrogel electrical performance. Thus, the dynamic stretchable sensibility of the PNAGA-30&LiCl hydrogel-based strain sensors before and after healing was investigated. Fig. 6c and Fig. S7 (ESI†) show the continuous resistance outputs of the original and healed PNAGA-30&LiCl hydrogel-based strain sensors for five cycles in small (100%) and large strain (400%). For the self-healed hydrogel sensors, the cyclic resistance output was almost the same as that of the original sensors at all test strains, proving that the healed hydrogel sensors still maintained the excellent electrical performance. Furthermore, both of the original and self-healed PNAGA-30&LiCl hydrogel-based strain sensors were adopted to test the bending–unbending movements of fingers. As shown in Fig. 6d and e, the curves of the relative resistance change for the hydrogel sensors before and after self-healing showed consistent trends and good stability. Therefore, the healed hydrogels were still suitable for serving as a strain sensor, which was functionally similar to the human skin capable of recovery of its responsive ability even after wound healing. Moreover, the PNAGA-30&LiCl hydrogels still maintained good electrical performance after re-processing (Fig. 6f). The PNAGA-30&LiCl hydrogels with good re-processability will have the following three advantages to be used as strain sensors. First, the shape of the strain sensors can be arbitrarily adapted to different human movements according to practical needs. Second, the serving life of the sensors can be largely extended. Third, as a recyclable and environmentally friendly electronic device, the sensors can ease the burden of environmental pollution.
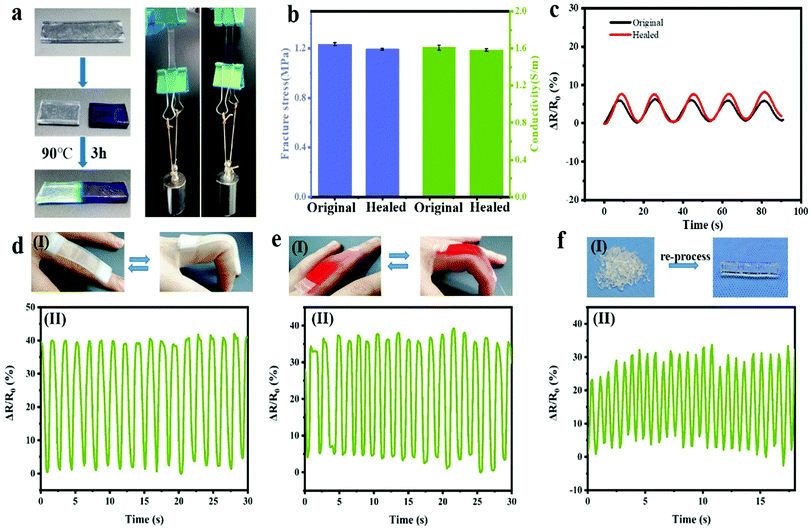 |
| Fig. 6 (a) Self-healing process of the PNAGA-30&LiCl hydrogel sheet and the healed hydrogel sheet could completely support 500 g weight. (b) Comparison of fracture stress and conductivity of the PNAGA-30&LiCl hydrogel before and after self-healing. (c) Time-dependent relative resistance changes of the original and self-healed hydrogel sensors at a small strain of 100% for five cycles. Relative resistance changes of the (d) original and (e) the self-healed hydrogel sensors with the motions of the index finger; (I) photographs of the continuous finger bending and unbending cycles; (II) the corresponding relative resistance changes. (f) Relative resistance changes of the reshaped hydrogel sensor with the bending and unbending of the index finger; (I) the hydrogel sensor regenerated from the hydrogel fragments; (II) the relative resistance changes of the reshaped hydrogel sensor with the motion of the index finger. | |
3.6 PNAGA-30&LiCl hydrogel strain sensors as wearable devices
Given the features of superior mechanical strength, electrical performance, re-processability and recoverability, the PNAGA-30&LiCl hydrogels were fabricated into a series of wearable strain sensors to monitor various human movements and tiny vibrations of pulse. As shown in Fig. 7a, the PNAGA-30&LiCl hydrogel-based strain sensors were applied to detect neck motions. When the neck was bent and straightened repeatedly, the real-time relative resistance change signals of the sensors could be stably and repeatedly recorded. Moreover, the electrical signals of the PNAGA-30&LiCl hydrogel-based strain sensors could be precisely detected with the bending and stretching movements of the index finger (Fig. 7b). In addition, the movement information of the elbow and the knee could also be obtained in a similar way, as shown in Fig. 7c and d. The responding signals of the hydrogel sensors towards the bending–stretching movements of the neck, index finger, elbow and knee showed a significant difference, demonstrating the high sensitivity of the hydrogel sensors to every complicated movement. Most importantly, all the resistance signals were very obvious and had a smooth baseline.
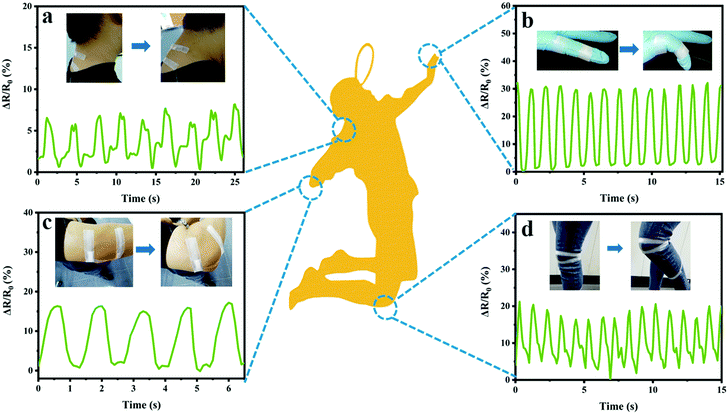 |
| Fig. 7 Relative resistance changes of the sensors with the movement of the (a) neck, (b) index finger, (c) elbow, and (d) knee. | |
Furthermore, a sandwich-like PNAGA-30&LiCl hydrogel-based strain sensor was fabricated (Fig. 8a), and fixed on the wrist of a volunteer to detect the blood pulse (Fig. 8b). Obviously, the relative resistance change signal varied with the pulsation, showing the good followability of the hydrogel sensors to fine vibration (Fig. 8c). As shown by the output signals of the relative resistance change, the average pulse was 78 times per minute, which was consistent with the pulse of a normal healthy adult. After exercise for 5 min, the amplitude and frequency of the output signal were strengthened significantly. For example, the average pulse after exercise increased to 102 times per minute. These results fully mirrored the pulse beat without and with exercise, and also demonstrated the high sensitivity and ultrafast responsiveness of the PNAGA-30&LiCl hydrogel-based strain sensors, thereby making them a superior candidate for human health detection.
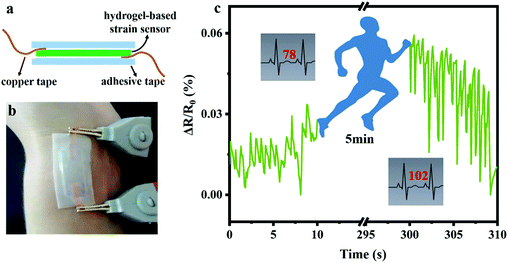 |
| Fig. 8 (a) Schematic illustration for the preparation of PNAGA-30&LiCl hydrogel-strain sensor construct. (b) Photograph of the PNAGA-30&LiCl hydrogel-based strain sensor as a wearable pulse monitor. (c) Relative resistance changes relative to time when monitoring the pulse before and after exercise for 5 min. | |
4. Conclusion
In summary, a hydrogen-bonding based physically cross-linked, thermoplastic and tough supramolecular hydrogel system was engineered by one-pot step-growth polymerization of N-acryloyl glycinamide (NAGA). Due to the strong and thermo-reversible hydrogen bonds among dual amide motifs, the PNAGA-30 hydrogels at a solid content of 30wt% showed attractive toughness, re-processability, and recyclability. Through a simple heating and cooling method, the PNAGA-30 hydrogels could be repeatedly processed into desirable shapes without degrading their mechanical properties. Furthermore, the PNAGA-30&LiCl hydrogels were prepared easily by incorporating LiCl into the hydrogels, which were capable of serving as multifunctional strain sensors to monitor large human movements and tiny vibrations, thereby showing great potential in applications including robotics, biomedical prosthetics and personal healthcare monitoring. Naturally, the strategy for the development of PNAGA-30&LiCl hydrogels can be further extended by mixing thermoplastic PNAGA-30 hydrogels with other functional additives or materials to obtain more advanced composite materials.
Conflicts of interest
There are no conflicts to declare.
Acknowledgements
The synthesis of NAGA was enthusiastically guided by Prof. Liu Wenguang and Mr Wu Yuanhao at School of Materials Science and Engineering, Tianjin University. This work was financially supported by the Key-Area Research and Development Program of Guangdong Province (2020B090924004), the National Natural Science Foundation of China (Grant No. 51873071 and 51873069), the National Key R&D Program of China (2018YFC1106300), and the Guangzhou Science and Technology Research Project (Grant No. 201707010150).
Notes and references
- T. Bai, P. Zhang, Y. Han, Y. Liu, W. Liu, X. Zhao and W. Lu, Soft Matter, 2011, 7, 2825–2831 RSC.
- M. A. Haque, T. Kurokawa, G. Kamita and J. P. Gong, Macromolecules, 2011, 44, 8916–8924 CrossRef CAS.
- J.-Y. Sun, X. Zhao, W. R. K. Illeperuma, O. Chaudhuri, K. H. Oh, D. J. Mooney, J. J. Vlassak and Z. Suo, Nature, 2012, 489, 133–136 CrossRef CAS.
- T. L. Sun, T. Kurokawa, S. Kuroda, A. B. Ihsan, T. Akasaki, K. Sato, M. A. Haque, T. Nakajima and J. P. Gong, Nat. Mater., 2013, 12, 932–937 CrossRef CAS.
- Y. Tamai, H. Tanaka and K. Nakanishi, Macromolecules, 1996, 29, 6761–6769 CrossRef CAS.
- K. Xue, S. S. Liow, A. A. Karim, Z. Li and X. J. Loh, Chem. Rec., 2018, 18, 1517–1529 CrossRef CAS.
- X. Zhao, Soft Matter, 2014, 10, 672–687 RSC.
- L. Wang, G. Gao, Y. Zhou, T. Xu, J. Chen, R. Wang, R. Zhang and J. Fu, ACS Appl. Mater. Interfaces, 2019, 11, 3506–3515 CrossRef CAS.
- Z. Wang, Y. Cong and J. Fu, J. Mater. Chem. B, 2020, 8, 3437–3459 RSC.
- S. Lin, X. Zhao, X. Jiang, A. Wu, H. Ding, Y. Zhong, J. Li, J. Pan, B. Liu and H. Zhu, Small, 2019, 15, 1900848 CrossRef.
- Y.-J. Liu, W.-T. Cao, M.-G. Ma and P. Wan, ACS Appl. Mater. Interfaces, 2017, 9, 25559–25570 CrossRef.
- D. Macaya and M. Spector, Biomed. Mater., 2012, 7, 012001 CrossRef CAS.
- C. Fan, L. Liao, C. Zhang and L. Liu, J. Mater. Chem. B, 2013, 1, 4251–4258 RSC.
- B. Xu, P. Zheng, F. Gao, W. Wang, H. Zhang, X. Zhang, X. Feng and W. Liu, Adv. Funct. Mater., 2017, 27, 1604327 CrossRef.
- G. Guan, C. Yu, M. Xing, Y. Wu, X. Hu, H. Wang and L. Wang, Polymers, 2019, 11, 810 CrossRef CAS.
- S. Wu, H. Dong, Q. Li, G. Wang and X. Cao, Carbohydr. Polym., 2017, 168, 147–152 CrossRef CAS.
- Y. Zhang, H. Gao, H. Wang, Z. Xu, X. Chen, B. Liu, Y. Shi, Y. Lu, L. Wen, Y. Li, Z. Li, Y. Men, X. Feng and W. Liu, Adv. Funct. Mater., 2018, 28, 1705962 CrossRef.
- L. Wang, G. Shan and P. Pan, Soft Matter, 2014, 10, 3850–3856 RSC.
- J. P. Gong, Y. Katsuyama, T. Kurokawa and Y. Osada, Adv. Mater., 2003, 15, 1155–1158 CrossRef CAS.
- Q. Wang, R. Hou, Y. Cheng and J. Fu, Soft Matter, 2012, 8, 6048–6056 RSC.
- J. Yang, J.-J. Zhao, F. Xu and R.-C. Sun, ACS Appl. Mater. Interfaces, 2013, 5, 12960–12967 CrossRef CAS.
- Y. Okumura and K. Ito, Adv. Mater., 2001, 13, 485–487 CrossRef CAS.
- D. Xu, J. Huang, D. Zhao, B. Ding, L. Zhang and J. Cai, Adv. Mater., 2016, 28, 5844–5849 CrossRef CAS.
- D. Zhao, J. Huang, Y. Zhong, K. Li, L. Zhang and J. Cai, Adv. Funct. Mater., 2016, 26, 6279–6287 CrossRef CAS.
- G. Jiang, C. Liu, X. Liu, G. Zhang, M. Yang and F. Liu, Macromol. Mater. Eng., 2009, 294, 815–820 CrossRef CAS.
- N. Yang, H. Yang, Z. Shao and M. Guo, Macromol. Rapid Commun., 2017, 38, 1700275 CrossRef.
- X. Dai, Y. Zhang, L. Gao, T. Bai, W. Wang, Y. Cui and W. Liu, Adv. Mater., 2015, 27, 3566–3571 CrossRef CAS.
- C. K. Roy, H. L. Guo, T. L. Sun, A. B. Ihsan, T. Kurokawa, M. Takahata, T. Nonoyama, T. Nakajima and J. P. Gong, Adv. Mater., 2015, 27, 7344–7348 CrossRef CAS.
- F. Luo, T. L. Sun, T. Nakajima, T. Kurokawa, A. B. Ihsan, X. Li, H. Guo and J. P. Gong, ACS Macro Lett., 2015, 4, 961–964 CrossRef CAS.
- S. Y. Zheng, H. Ding, J. Qian, J. Yin, Z. L. Wu, Y. Song and Q. Zheng, Macromolecules, 2016, 49, 9637–9646 CrossRef CAS.
- Y. Ko, J. Kim, H. Y. Jeong, G. Kwon, D. Kim, M. Ku, J. Yang, Y. Yamauchi, H.-Y. Kim, C. Lee and J. You, Carbohydr. Polym., 2019, 203, 26–34 CrossRef CAS.
- Q. Wu, J. Wei, B. Xu, X. Liu, H. Wang, W. Wang, Q. Wang and W. Liu, Sci. Rep., 2017, 7, 41566 CrossRef.
- A. Dutta, S. Maity and R. K. Das, Macromol. Mater. Eng., 2018, 303, 1800322 CrossRef.
- Z. He, H. Niu, N. Zheng, S. Liu and Y. Li, Polym. Chem., 2019, 10, 4789–4800 RSC.
- C. Wang, K. Hu, C. Zhao, Y. Zou, Y. Liu, X. Qu, D. Jiang, Z. Li, M.-R. Zhang and Z. Li, Small, 2020, 16, 1904758 CrossRef CAS.
Footnotes |
† Electronic supplementary information (ESI) available. See DOI: 10.1039/d0tb02049d |
‡ Xiaohua Zhang and Xuefeng Yang contributed equally to this work. |
|
This journal is © The Royal Society of Chemistry 2021 |
Click here to see how this site uses Cookies. View our privacy policy here.