DOI:
10.1039/D1TA03246A
(Paper)
J. Mater. Chem. A, 2021,
9, 17434-17441
A multifunctional covalently linked graphene–MOF hybrid as an effective chemiresistive gas sensor†
Received
18th April 2021
, Accepted 22nd July 2021
First published on 23rd July 2021
Abstract
A hybrid of GA@UiO-66-NH2 was synthesized based on the covalent assembly of graphene acid (GA) and the amine functionalized UiO-66 metal–organic framework through amide bonds. This strategy endows the material with unique properties, such as hierarchical pores, a porous conductive network decorated with functional groups, a high specific surface area, and a good chemical and thermal stability. The resultant hybrid has an electrical resistance of ∼104 Ω, whereas the pristine GA and UiO-66-NH2 possess an electrical resistance of ∼102 Ω and ∼109 Ω, respectively. The hybrid GA@UiO-66-NH2 was demonstrated for CO2 chemiresistive sensing and displayed a very fast response and quick recovery time of ∼18 s for 100% CO2, at 200 °C. While the pristine GA exhibits negligible response under the same conditions, GA@UiO-66-NH2 exhibited a response of 10 ± 0.6%. Further, in situ temperature dependent Raman studies during CO2 exposure confirm the presence of strong hydrogen bonding interaction between CO2 and the amide functionality present on GA@UiO-66-NH2. The resulting gas sensing characteristics of GA@UiO-66-NH2 are majorly attributed to the better interaction of CO2 at the amide/amine functional groups and the readily accessible hierarchical pores. This design strategy opens new horizons in the development of covalently linked hybrids with hierarchical porous conductive networks which can help to improve the gas sensing properties of MOF-based materials.
1. Introduction
The detection of CO2via chemiresistive technology has a huge commercial potential, since it is a very sensitive marker for human activity.1–3 From the CO2 concentration in the atmosphere of closed rooms and buildings, the occupancy by people can be determined.4 This can be used to predict and prevent events related to overcrowding of areas, which ultimately can lead to disasters. Also, CO2 detection can be used by rescuers after earthquakes or similar incidents to detect survivors that are trapped under the rubble of collapsed structures.5 Current technologies detect CO2 based on non-dispersive IR optical sensors.6 Even though these sensors are well established, they still have some drawbacks, including their complexity, cost, scalability, and energy consumption. Chemiresistive sensing is a good alternative to the currently used detection methods.7–15 Upon an outer stimulus, these sensors rely on changes in electrical resistance, that allow continuous monitoring of various gas concentrations. One of the most critical challenges in the development of CO2 gas sensors is the inclusion of active sites for adsorption/desorption, where CO2 gas can cause changes in electrical parameters. Some nanostructured metal oxides, conducting polymers and other carbon-based materials have been considered as active materials in chemiresistive CO2 gas sensors.16–22 Among these, amine functionalized graphene and polymer materials are regarded as attractive materials with the capability of low concentration detection with high sensitivity and selectivity under ambient conditions.19,23–28 However, these amine-based composite sensors display sharply decreasing sensitivity with reduced interactions over time. Hence, the need for the modifications of the active species over graphene-based materials to improve the signal-transducer interaction is essential for CO2 sensing.
Metal–organic frameworks (MOFs), as a class of porous materials have various potential applications in the field of energy storage and conversion and also a lot of efforts have been made in the development of MOF-based sensors.29–33 In particular, conjugated layered two-dimensional (2D) MOFs have recently been demonstrated as attractive CO2 gas sensors by combining the desired properties of conductivity, nano porosity and the presence of functional groups for analyte adsorption.11,12,34 However, their response and recovery times are still limiting their potential application. Although many pristine MOFs have conductivity limitations (mostly near insulators), there are some examples of conductive 2D layered MOF structures.35 Several efforts have been made to improve the conductivity of three dimensional (3D) MOFs, for instance by hybridization with other conducting/semiconducting materials such as metal oxide nanoparticles, quantum dots, polymers, or carbon based materials.36–44 In recent years, our groups demonstrated the noncovalent and covalent hybridization of MOFs with graphene to prepare conductive materials for various energy applications.33,45–48 In particular, the covalent linking of graphene with appropriately functionalized MOFs has shown promising electronic properties for energy applications. Additionally, the strong bonding between each of the pristine components, the hierarchical pore architecture, and the enhanced thermal and chemical stabilities are all features which are sought after for the development of robust chemiresistive sensors.
Herein, we report the use of a covalently assembled hybrid constructed from graphene acid (GA) and amine functionalized UiO-66-NH2 (Zr6O4(OH)4(NH2-bdc)6 with NH2-bdc2− = 2-amino-1,4-benzenedicarboxylate)49–53 for chemiresistive CO2 sensing. In the material, UiO-66-NH2 is covalently bound with GA via amide bonds, creating the hybrid GA@UiO-66-NH2 (Fig. 1). The material shows hierarchical porosity, wherein the micropores are located at the octahedral UiO-66-NH2 nanocrystals and the mesopores are built up through the stacking of GA through the MOF nanocrystals. The hierarchical architecture facilitates gas diffusion through the material, hence providing faster access to the interaction sites for the analytes. On the other hand, the interconnected conductive network ensures quick readout of the signal. In comparison, graphene has a low electrical resistance (∼10−6 Ω) and high carrier electron mobility, but the lack of specific and strong interaction sites for the adsorption of gaseous analytes, renders it unusable for chemiresistive sensing in its pristine form. Here, the resultant hybrid GA@UiO-66-NH2 provides interaction sites at the amide linkages present at the interface of graphene and the MOF for detectable change in resistance upon CO2 interaction. These results are clarified by temperature dependent in situ Raman spectroscopy during exposure of the material to CO2. In this study, a good correlation has been achieved between sensing characteristics and the novel functional properties obtained after integrating the MOF with graphene.
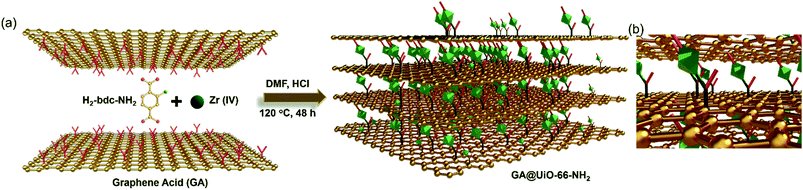 |
| Fig. 1 (a) Schematic representation of the multifunctional (hierarchical porosity, conductive network, amine/amide functional groups) graphene–MOF hybrid (b where carboxyl groups of graphene acid are covalently linked via amide bonds to the amine groups of UiO-66-NH2 MOF. | |
2. Experimental section
2.1. Synthesis of graphene acid
Graphene acid was prepared according to the literature.31 To prepare graphene acid, the commercial fluorinated graphite (120 mg) was sonicated in presence of 15 mL of N,N-dimethylformamide (DMF) for 4 h under nitrogen atmosphere in a 25 mL round-bottom flask. To this mixture, 800 mg sodium cyanide (NaCN) was added and the mixture was heated to 130 °C with a condenser under stirring (500 rpm) for 24 h. During the reaction procedure, the intermediate and final product were cooled to room temperature, after which an equal amount of acetone was added. The materials were then separated by centrifugation and further purified by successive washing steps using DMF, dichloromethane, acetone, ethanol and water (each step was repeated four times). Additionally, hot (80 °C) DMF and water were also used. During the final centrifugation step with water, it was necessary to apply centrifugal force of up to 25
000 rpm to isolate the product. The resultant cyanographene was mixed with HNO3 (65%) under stirring at room temperature. The mixture was then heated at 100 °C under reflux with stirring (350 rpm) for 24 h. Further, the product was thoroughly washed with water to remove soluble impurities to obtain graphene acid as the final material. It should be noted that, the stable aqueous suspensions of GA were prepared by adjusting the pH of the purified suspension to ∼8.
2.2. Synthetic procedure for UiO-66-NH2
Octahedral nano crystals of UiO66-NH2 were prepared according to a slightly modified literature procedure. ZrCl4 (0.233 g) and 2-aminoterepthalic acid (0.181 g)and 4-aminobenzoic acid (0.244 g) were mixed in 10 mL dimethylformamide (DMF) solvent in glass vial and dissolved by addition of 0.16 mL of hydrochloric acid (HCl). Later, the glass vial was tightly closed and placed in an oven at 120 °C for 2 days. After cooling to room temperature, the supernatant was decanted, and the pale-yellow precipitate was washed three times with DMF and three times with methanol. Methanol was decanted and replaced once per day during the course of three days and later it was removed under vacuum. The product was heated under vacuum to 150 °C for 5 h. The sample was cooled to room temperature and stored under ambient conditions.
2.3. Preparation of hybrid GA@UiO-66-NH2
A sample of 100 mg of GA was exfoliated in 10 mL of DMF solution for 30 minutes under sonication. To this GA suspension, ZrCl4 (0.233 g), 2-aminoterepthalic acid (0.181 g), 4-aminobenzoic acid (0.244 g) and 0.16 mL of HCl was added into a 25 mL glass vial. The resultant mixture was sonicated for 30 minutes. The resultant mixture was transferred to a 50 mL glass vial and placed at 120 °C for 2 days. The obtained black gel was washed several times with methanol and dried under nitrogen atmosphere at 100 °C.
2.4. Chemiresistive sensing measurement
All the materials tested for chemiresistive CO2 sensing were weighed and pressed into pellets of uniform thickness. 150 mg of dried powdered sample were weighed and pressed with a pressure of 75 kg cm−2 using a hydraulic pellet press. Pellets with uniform thickness of 1 mm were obtained. Using a toothpick, small silver dots (diameter of 1 mm) were placed on the upper surface of the pellet. This silver conductive adhesive paste has a sheet resistance of <0.025 ohm square−1 @ 0.001 mm thick. A Keithley source meter (Model no. 2450) was used to measure the resistance change in the presence and absence of CO2 gas. The argon (reference/balance gas) and CO2 gas flow into the gas reactor chamber were controlled by using Alicat Mass Flow Controllers (MFCs). A computer-controlled data acquisition system was used to monitor the sensor responses through the resistance-based studies. In order to carry out the sensing studies, the pellet was placed on a hollow graphite heating block containing the halogen lamp to provide the thermal energy. Argon was chosen both as reference and balancing gas. Initially, up to a desired temperature of sensing (controlled by temperature control unit), argon gas was introduced through the inlet in the gas reactor chamber. A calculated amount of CO2 gas was injected on obtaining a stable resistance value under argon flow. The mixing chamber was used to mix the CO2 and argon gas uniformly to obtain the desired quantity of gas flow. The resistance of the sensor/pellet was measured in presence and absence of CO2 gas.
3. Results and discussions
The coupling of the carboxylate functional groups of GA with pristine UiO-66-NH2 under solvothermal conditions was done in analogy to our previously reported study.54 The powder X-ray diffraction of the resulting hybrid (GA@UiO-66-NH2) material confirms the structural integrity of the metal–organic framework (Fig. 2a).
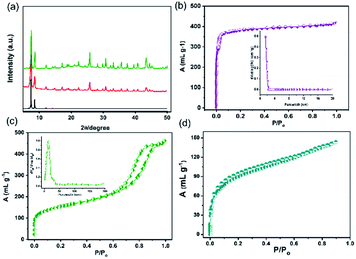 |
| Fig. 2 (a) Simulated (black) and as synthesized powder XRD patterns of pristine UiO-66-NH2 (red) covalent hybrid GA@UiO-66-NH2 (green) nitrogen adsorption desorption isotherm at 77 K of (b) Uio-66-NH2 (c) GA@UiO-66-NH2, inset shows pore size distribution calculated through NLDFT method. (d) CO2 adsorption isotherm measured at 195 K, for GA@UiO-66-NH2. | |
Moreover, the obtained N2 physisorption isotherm of GA@UiO-66-NH2 features a hysteresis loop indicative of mesopores, with a Brunauer–Emmett–Teller (BET) surface area of 598 m2 g−1 and a pore volume of 1.09 cm3 g−1. The pore size distribution of hybrid calculated from nonlocal density functional theory (NLDFT) method gives evidence for pores in the range from 1 to 60 nm (Fig. 2c inset). Further the Barrett, Joyner and Halenda (BJH) pore size distribution confirms meso pores in the 1–60 nm (Fig. S2a†). Contrary, pristine UiO-66-NH2 shows microporous nature and BET surface area of 1185 m2 g−1 (Fig. 2b) and NLDFT method shows micropore distribution (Inset Fig. 2b). These mesopores in GA@UiO-66-NH2 arise from the covalent pillaring of the GA with the UiO-66-NH2 nanocrystals. Additionally, typical features of microporous materials are observed, which originate from the intrinsic pores of the UiO-66-NH2 nanocrystals. Furthermore, CO2 sorption isotherms were measured GA@UiO-66-NH2 at 195 K, and the resultant hybrid shows a significant CO2 uptake of 157 cm3 at p/p0 ∼ 1 (Fig. 2c). Further DFT pore size distribution calculated CO2 adsorption isotherm micro–meso pore size distribution (Fig. S2b†). The deconvolution of the high resolution Zr 3d, C 1s, O 1s and N 1s from X-ray photoelectron spectroscopy (XPS) clearly reveal covalent bonds between GA and UiO-66-NH2 nanocrystals via amide bond (Fig. 3a–d).55 Scanning electron microscopy (SEM) and transmission electron microscopy (TEM) of prestine Uio-66 shows octahedron nanocrystal with size in the range of 150 to 200 nm (Fig. S1† and 4a). Finally, high-resolution transmission electron microscopy (HRTEM) images exhibit the formation of graphene nanosheets with octahedral nanocrystals between 5 to 10 nm (Fig. 4b, c, S2c and d†). Additionally, high-angle annular dark-field (HAADF) and elemental mapping from energy dispersive X-ray analysis (EDAX) shows uniform distribution of Zr, C, N, and O throughout the sample (Fig. 4d–i).
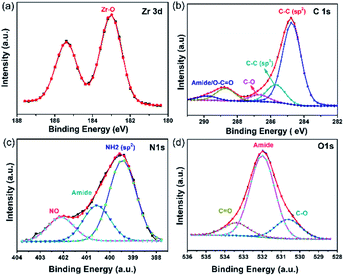 |
| Fig. 3 Magnified XPS spectra of (a) Zr 3d (b) C 1s (c) N 1s and (d) O 1s of GA@UiO-66-NH2. | |
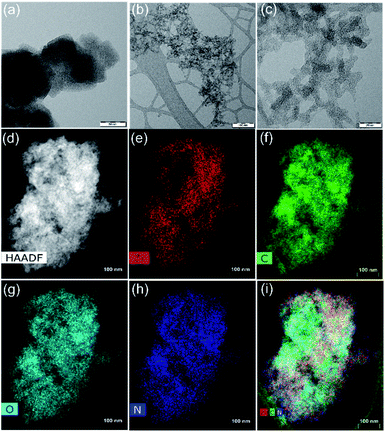 |
| Fig. 4 HRTEM images of (a) Uio-66-NH2 octahedron nanocrystals (b and c) GA@UiO-66-NH2 nanocrystals of anchored graphene acid nanosheets. (d) HAADF image and elemental mapping of (e) Zr, (f) C, (g) O, (h) N, and (i) the combined distribution of Zr, C and N in GA@UiO-66-NH2. | |
Hybridization of GA with UiO-66-NH2 through covalent bonds yielded a hybrid system featuring electrical conductivity, hierarchical pores, amine/amide functional groups and a good CO2 capacity, which is ideally suited for CO2 gas sensing applications. The gas sensing measurements were carried out using a home-built two-probe gas sensing station. The two-probe method is suitable for measuring the electrical resistance of materials that have a much higher resistance than the contacts and wires used during the measurement. The probes are connected to a Keithley source meter. The gas sensing/testing chamber is equipped with a heater and gas-supplying channel that were controlled precisely by a temperature-control device and mass flow controllers (MFCs), respectively (Fig. S3†). The sensing characteristics of the material in the form of pressed pellets tested in this work include response%, response time and recovery time towards different amounts of CO2 (100% to 5%) by recording the change in resistance versus time (Fig. S4†). Using the above-described setup, the resistance of pellets of GA, UiO-66-NH2 and GA@UiO-66-NH2 were determined, obtaining values of around 102 Ω, 109 Ω and 104 Ω, respectively (Fig. 5a). The low resistance of pristine GA results from the delocalized π-electrons. Nevertheless, GA do not possess any favourable interaction sites for CO2. UiO-66-NH2 features suitable interaction sites but has a high resistance due to the very poor orbital overlap between the Zr(IV) d-orbitals and the linker frontier orbitals. Covalent assembly of these two components leads to an intermediate resistance in the range of ∼104 Ω which is in the range of typical metal–oxide semiconductor based sensors like SnO2.56
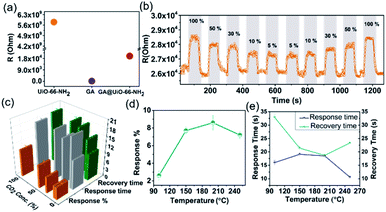 |
| Fig. 5 (a) Comparison between the resistance of UiO-66-NH2, GA and GA@UiO-66-NH2, (b) gas sensing performance of GA@UiO-66-NH2 to 5% to 100% CO2 concentration at an operating temperature of 200 °C, (c) comparison of gas sensing characteristics (response%, response time and recovery time) from 100% to 5% CO2 at an operating temperature of 200 °C. Effect of temperature on the sensing characteristics of GA@UiO-66-NH2 (d) response% and (e) response and recovery times. | |
Based on these properties we explored GA@UiO-66-NH2 as a CO2 chemiresistive sensor. So far, only a handful graphene hybrids haven been reported for CO2 sensing (Table S1†).3,57–64 As expected, due to the lack of strong interaction sites, GA shows negligible response towards CO2 (100% to 5%) at 200 °C (Fig. S5†). Remarkably, GA@UiO-66-NH2 shows significant response of 10 ± 0.6% at the same temperature for 100% CO2. For 50, 30, 10 and 5% CO2, GA@UiO-66-NH2 showed responses of 8 ± 0.8%, 6 ± 0.5%, 4 ± 0.2% and 4 ± 0.3% respectively, at 200 °C (Fig. 5b). The response is found to also decrease almost linearly with decrease in CO2 concentration (Fig. S6†). The recyclability was demonstrated with three cycles of response–recovery curves (Fig. S7 and S8†). The effect of temperature on the sensing characteristics of the material is shown in Fig. 5d and e. The CO2 response increased with increase in temperature from 2 ± 0.5% at 100 °C to 8 ± 0.8% at 200 °C for 50% CO2. Further increase in temperature to 250 °C resulted in a slight drop in the response to 7 ± 0.4%. A very fast response and quick recovery time (∼18 s) are observed at 200 °C. It should be noted that the obtained response/recovery times are either comparable or superior to other reported MOFs as shown in Table S2†.16–19,34,36,56,65–71 The gas sensing performance of GA@UiO-66-NH2 in presence of synthetic air is shown in Fig. S9.† The measured response% for 50% CO2 in presence of synthetic air balance was 7%. This response% value is like the one measured in presence of argon balance gas, that is 8 ± 0.8%. The response and recovery times were in the range of 15–18 s. Hence, the effect of synthetic air was negligible on the gas sensing performance of GA@UiO-66-NH2. For pristine UiO-66 MOF, a stable resistance of 0.5 × 10−11 Ω was measured at 200 °C under argon flow. Upon exposure to CO2, this material did not show any detectable changes in resistance (Fig. S10†).
This could be due to the lack of strong interactive sites in the MOF. Nevertheless, the amide bridge at GA@UiO-66-NH2 connecting the graphene basal plane and –NH2 functionality on the pristine UiO-66-NH2 are known to have affinity towards CO2. The positive sensing curves (Fig. 5b) suggest that donor (amide/amine)–acceptor (CO2) interactions led to a decrease in the conductivity of GA in the hybrid material. We rule out the possibility of sole contribution of UiO-66-NH2 to the sensing characteristics due to its several orders of magnitude higher resistance compared to GA. It is reasonable to predict that the CO2 interactions with the amide functionality at the interface of GA and the MOF are majorly responsible for the observed gas sensing properties (Fig. 6). The MOF has two roles in this hybrid, on one hand it controls the distance between the conductive GA layers constructing the mesoporous diffusion channels and on the other hand it provides the interaction sites at the amide functionalities at the GA–MOF interface.
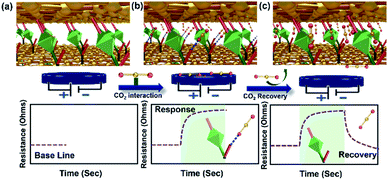 |
| Fig. 6 Schematic illustration of possible sensing mechanism: (a) GA@UiO-66-NH2 shows porous network with amide linkage (b) the CO2 interactions with amide linkage present at the basal plane of the GA could bring about the positive change in resistance of GA@UiO-66-NH2 chemiresistive sensor. (c) Upon removal of CO2, the steady state baseline has been retrieved suggesting that CO2 interaction is reversible. | |
Raman spectroscopy is a very useful technique to understand the interaction of gas molecules with MOFs. In order to verify the interactions proposed in Fig. 6, temperature dependent in situ Raman spectroscopy studies were conducted on GA@UiO-66-NH2 in the presence of CO2. Two new broad features appear around 1230 cm−1 and 1383 cm−1 which are assigned to adsorbed CO2 Fermi resonance modes (Fig. S11 and enlarged figures of figure of S11 shown in Fig. S12–S18†). Dosing of Ar instead of CO2, did not yield any new signals in this region (Fig. S19†). Variable temperature Raman spectroscopy studies were conducted in CO2 environment from room temperature (RT) to 300 °C (Fig. 7). Fig. 7a shows the temperature dependent Raman shift of the vibrational modes corresponding to the C
O stretching, as well as the D and G bands of the GA@UiO-66-NH2. The temperature dependent mode behaviour in Fig. 7a exhibits the expected monotonous softening (decrease in the frequency) with increase in temperature suggesting that the hybrid is stable in the temperature range of RT to 300 °C in the CO2 environment and there is no effect of CO2 adsorption on these modes. Fig. 7b shows the temperature dependent Raman mode behaviour of the amide (CO–NH) linkage connecting the MOF and GA, and the Fermi doublet of the CO2 modes. Interestingly, the observed behaviour can be divided into three distinct regions (i) RT to 125 °C, (ii) 125 °C to 200 °C and (iii) above 200 °C.
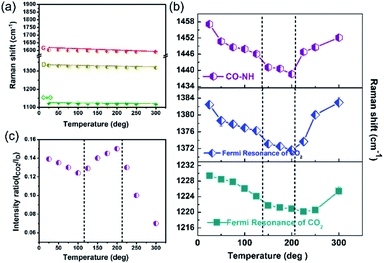 |
| Fig. 7 Temperature dependent Raman study of GA@UiO-66-NH2 under CO2 atmosphere- (a) C O stretching, D and G band, (b) CO–NH and Fermi resonance mode of CO2. (c) Intensity ratio vs. temperature plot showing maximum adsorption of CO2 around 200 °C. | |
The Raman modes corresponding to the amide CO–NH linkage of GA@UiO-66-NH2 and the Fermi resonance mode of CO2 show the expected softening up to 125 °C. This suggests that there is a gradual uptake of CO2 into the pore space without strong interactions with the walls of the pores of the hybrid with rise in temperature. Between 125 °C to 200 °C, we observe that the change in frequency shift remains nearly constant until 200 °C suggesting that further uptake of CO2 is assisted by a strong chemical interaction between CO2 and the CO–NH bond. We observe that above 200 °C there is a sudden increase in the frequency of both the CO–NH linkages and the two Fermi resonance modes of CO2 and they shift back close to the values observed at room temperature. This suggests that CO2 interacts only weakly with CO–NH above 200 °C, possibly due to the high kinetic energy associated with CO2. This is consistent with the chemiresistive gas sensing results (Fig. 5d) where 200 °C is found to be the optimum temperature for achieving the highest CO2 response. Further, the relative intensity ratio of CO2 with respect to the D band (ICO2/D) as shown in Fig. 7c and we observe that the CO2 intensity initially decreases up to 125 °C and then increases to a maximum intensity at 200 °C and then falls steeply beyond.
4. Conclusions
In summary, we have reported a uniquely simple, convenient, and scalable covalent assembly of graphene acid with amine functionalized UiO-66-NH2 under solvothermal conditions. The GA@UiO-66-NH2 hybrid acts as a chemiresistive CO2 sensor with significant efficiency and remarkable stability. The novel CO2 sensing characteristics might be attributed to the synergistic effect between GA and UiO-66-NH2 in terms of good conductivity, hierarchical porous nature facilitating gas diffusion and amide/amine based interaction sites. Temperature-dependent Raman studies during CO2 adsorption confirm interaction of CO2 molecules with the amide bonds of GA@UiO-66-NH2. The present data suggests a proof-of-concept for the use of this hybrid material for gas-sensing applications and there is a lot of room for the development of other conductive graphene–MOF hybrids through covalent assembly for various gas and bio-sensing applications.
Author contributions
K. Jayaramulu: idea, conceptualization, writing – original draft, super vision. M. DMello: Gas sensing measurements, writing. K. Kesavan: Raman measurments, writing – original draft. Schneemann: investigation, supervision. M. Otyepka: graphene synthesis, and investigation. S. Kment: electrochemical measurements, investigation. C. Narayna: Raman measurements and its investigation, S.Kalidindi: sensing measurements and its investigation, R. S. Varma: review & editing. R. Zboril: writing – review & editing. R. A. Fischer: writing – review & editing, super vision.
Conflicts of interest
There are no conflicts to declare.
Acknowledgements
KJR acknowledges support from Indian Institute of Technology Jammu for providing a seed grant (SGT-100038), SERB SRG/2020/000865 and CVD/2021/000086. This research was funded by the Operational Programme Research, Development and Education—European Regional Development Fund, project CZ.02.1.01/0.0/0.0/16_019/0000754 of the Ministry of Education, Youth and Sports of the Czech Republic. RZ and SK acknowledge the support from the Czech Science Foundation, project no. 19-27454X. MED acknowledges Council of Scientific & Industrial Research (CSIR), New Delhi for SRF fellowship 09/1052(0010)/2020-EMR-I) and Manipal Academy of Higher Education (MAHE) for PhD registration. MO acknowledges the ERC grant (683024) from the EU Horizon 2020 Research and Innovation Programme. the German Research Foundation (DFG) within e-conversion (Fundamentals of Energy Conversion Processes, EXC 2089) supported the work. K. K gratefully acknowledges the Department of Science and Technology for the National Post Doctoral Fellowship (PDF/2016/001193). A. S. gratefully acknowledges the Fonds der chemischen Industrie for a Liebig Fellowship.
References
- M. Gruber, A. Trüschel and J.-O. Dalenbäck, Energy and Buildings, 2014, 84, 548–556 CrossRef.
- P. Puligundla, J. Jung and S. Ko, Food Control, 2012, 25, 328–333 CrossRef CAS.
- A. Star, T. R. Han, V. Joshi, J. C. P. Gabriel and G. Grüner, Adv. Mater., 2004, 16, 2049–2052 CrossRef CAS.
- S. Kanaparthi and S. G. Singh, ACS Appl. Nano Mater., 2019, 2, 700–706 CrossRef CAS.
- F. H. Weinlich, Geofluids, 2014, 14, 143–159 CrossRef CAS.
- X. Jia, J. Roels, R. Baets and G. Roelkens, Sensors, 2019, 19, 4260 CrossRef CAS PubMed.
- N. Joshi, T. Hayasaka, Y. Liu, H. Liu, O. N. Oliveira and L. Lin, Microchim. Acta, 2018, 185, 213 CrossRef PubMed.
- S. Pandey, J. Sci.: Adv. Mater. Devices, 2016, 1, 431–453 Search PubMed.
- F. L. Meng, Z. Guo and X. J. Huang, TrAC, Trends Anal. Chem., 2015, 68, 37–47 CrossRef CAS.
- D. X. Ju, H. Y. Xu, Z. W. Qiu, Z. C. Zhang, Q. Xu, J. Zhang, J. Q. Wang and B. Q. Cao, ACS Appl. Mater. Interfaces, 2015, 7, 19163–19171 CrossRef CAS.
- M. G. Campbell, D. Sheberla, S. F. Liu, T. M. Swager and M. Dincă, Angew. Chem., Int. Ed., 2015, 54, 4349–4352 CrossRef CAS PubMed.
- M. G. Campbell, S. F. Liu, T. M. Swager and M. Dincă, J. Am. Chem. Soc., 2015, 137, 13780–13783 CrossRef CAS PubMed.
- D. Ju, H. Xu, Z. Qiu, J. Guo, J. Zhang and B. Cao, Sens. Actuators, B, 2014, 200, 288–296 CrossRef CAS.
- F. Wang, H. Gu and T. M. Swager, J. Am. Chem. Soc., 2008, 130, 5392–5393 CrossRef CAS PubMed.
- F. I. Bohrer, A. Sharoni, C. Colesniuc, J. Park, I. K. Schuller, A. C. Kummel and W. C. Trogler, J. Am. Chem. Soc., 2007, 129, 5640–5646 CrossRef CAS.
- Y. Zhu, Y. Zhao, J. Ma, X. Cheng, J. Xie, P. Xu, H. Liu, H. Liu, H. Zhang, M. Wu, A. A. Elzatahry, A. Alghamdi, Y. Deng and D. Zhao, J. Am. Chem. Soc., 2017, 139, 10365–10373 CrossRef CAS PubMed.
- S. Pandey and K. K. Nanda, ACS Sensors, 2016, 1, 55–62 CrossRef CAS.
- Y. Zhang, J. J. Kim, D. Chen, H. L. Tuller and G. C. Rutledge, Adv. Funct. Mater., 2014, 24, 4005–4014 CrossRef CAS.
- K. Saetia, J. M. Schnorr, M. M. Mannarino, S. Y. Kim, G. C. Rutledge, T. M. Swager and P. T. Hammond, Adv. Funct. Mater., 2014, 24, 492–502 CrossRef CAS.
- N. Ramgir, N. Datta, M. Kaur, S. Kailasaganapathi, A. K. Debnath, D. K. Aswal and S. K. Gupta, Colloids Surf., A, 2013, 439, 101–116 CrossRef CAS.
- F. J. Ibañez and F. P. Zamborini, Small, 2012, 8, 174–202 CrossRef PubMed.
- B. T. Marquis and J. F. Vetelino, Sens. Actuators, B, 2001, 77, 100–110 CrossRef CAS.
- Y. Zhang, B. R. Bunes, N. Wu, A. Ansari, S. Rajabali and L. Zang, Sens. Actuators, B, 2018, 255, 1814–1818 CrossRef CAS.
- S. Wang, J. Liu, H. Zhao, Z. Guo, H. Xing and Y. Gao, Inorg. Chem., 2018, 57, 541–544 CrossRef CAS PubMed.
- F. Wang, Y. T. Wang, H. Yu, J. X. Chen, B. B. Gao and J. P. Lang, Inorg. Chem., 2016, 55, 9417–9423 CrossRef CAS PubMed.
- S. Mirabella, I. P. Oliveri, F. Ruffino, G. MacCarrone and S. Di Bella, Appl. Phys. Lett., 2016, 109, 143108 CrossRef.
- S. F. Liu, L. C. H. Moh and T. M. Swager, Chem. Mater., 2015, 27, 3560–3563 CrossRef CAS.
- J. Hammond, B. Marquis, R. Michaels, B. Oickle, B. Segee, J. Vetelino, A. Bushway, M. E. Camire and K. Davis-Dentici, Sens. Actuators, B, 2002, 84, 113–122 CrossRef CAS.
- M. D. Allendorf, R. Dong, X. Feng, S. Kaskel, D. Matoga and V. Stavila, Chem. Rev., 2020, 120, 8581–8640 CrossRef CAS PubMed.
- M. Wang, H. Shi, P. Zhang, Z. Liao, M. Wang, H. Zhong, F. Schwotzer, A. S. Nia, E. Zschech, S. Zhou, S. Kaskel, R. Dong and X. Feng, Adv. Funct. Mater., 2020, 30, 2002664 CrossRef CAS.
- H. Arora, R. Dong, T. Venanzi, J. Zscharschuch, H. Schneider, M. Helm, X. Feng, E. Cánovas and A. Erbe, Adv. Mater., 2020, 32, 1907063 CrossRef CAS PubMed.
- L. E. Kreno, K. Leong, O. K. Farha, M. Allendorf, R. P. Van Duyne and J. T. Hupp, Chem. Rev., 2012, 112, 1105–1125 CrossRef CAS.
- K. Jayaramulu, D. P. Dubal, A. Schneemann, V. Ranc, C. Perez-Reyes, J. Stráská, Š. Kment, M. Otyepka, R. A. Fischer and R. Zbořil, Adv. Funct. Mater., 2019, 29, 1902539 CrossRef.
- I. Stassen, J.-H. Dou, C. Hendon and M. Dincă, ACS Cent. Sci., 2019, 5, 1425–1431 CrossRef CAS PubMed.
- C. H. Hendon, A. J. Rieth, M. D. Korzyński and M. Dincă, ACS Cent. Sci., 2017, 3, 554–563 CrossRef CAS PubMed.
- C.-W. Kung, P.-C. Han, C.-H. Chuang and K. C. W. Wu, APL Mater., 2019, 7, 110902 CrossRef.
- P. Q. Liao, J. Q. Shen and J. P. Zhang, Coord. Chem. Rev., 2018, 373, 22–48 CrossRef CAS.
- Y. Wang, W. Zhang, X. Wu, C. Luo, Q. Wang, J. Li and L. Hu, Synth. Met., 2017, 228, 18–24 CrossRef CAS.
- C. W. Kung, T. H. Chang, L. Y. Chou, J. T. Hupp, O. K. Farha and K. C. Ho, Electrochem. Commun., 2015, 58, 51–56 CrossRef CAS.
- L. Zhang, Z. Su, F. Jiang, L. Yang, J. Qian, Y. Zhou, W. Li and M. Hong, Nanoscale, 2014, 6, 6590–6602 RSC.
- A. A. Talin, A. Centrone, A. C. Ford, M. E. Foster, V. Stavila, P. Haney, R. A. Kinney, V. Szalai, F. El Gabaly, H. P. Yoon, F. Léonard and M. D. Allendorf, Science, 2014, 343, 66–69 CrossRef CAS.
- S. Horike, D. Umeyama and S. Kitagawa, Acc. Chem. Res., 2013, 46, 2376–2384 CrossRef CAS PubMed.
- M. D. Allendorf, A. Schwartzberg, V. Stavila and A. A. Talin, Chem.–Eur. J., 2011, 17, 11372–11388 CrossRef CAS.
- C. G. Silva, A. Corma and H. García, J. Mater. Chem., 2010, 20, 3141–3156 RSC.
- V. Urbanová, K. Jayaramulu, A. Schneemann, Š. Kment, R. A. Fischer and R. Zbořil, ACS Appl. Mater. Interfaces, 2018, 10, 41089–41097 CrossRef PubMed.
- K. Jayaramulu, J. Masa, O. Tomanec, D. Peeters, V. Ranc, A. Schneemann, R. Zboril, W. Schuhmann and R. A. Fischer, Adv. Funct. Mater., 2017, 27, 1700451 CrossRef.
- K. Jayaramulu, F. Geyer, M. Petr, R. Zboril, D. Vollmer and R. A. Fischer, Adv. Mater., 2017, 29, 1605307–1605312 CrossRef.
- K. Jayaramulu, K. K. Datta, C. Rosler, M. Petr, M. Otyepka, R. Zboril and R. A. Fischer, Angew. Chem., Int. Ed., 2016, 55, 1178–1182 CrossRef CAS PubMed.
- J. D. Xiao, Q. Shang, Y. Xiong, Q. Zhang, Y. Luo, S. H. Yu and H. L. Jiang, Angew. Chem., Int. Ed., 2016, 55, 9389–9393 CrossRef CAS PubMed.
- H. Saleem, U. Rafique and R. P. Davies, Microporous Mesoporous Mater., 2016, 221, 238–244 CrossRef CAS.
- D. Sun, W. Liu, M. Qiu, Y. Zhang and Z. Li, Chem. Commun., 2015, 51, 2056–2059 RSC.
- L. Shen, W. Wu, R. Liang, R. Lin and L. Wu, Nanoscale, 2013, 5, 9374–9382 RSC.
- C. Zlotea, D. Phanon, M. Mazaj, D. Heurtaux, V. Guillerm, C. Serre, P. Horcajada, T. Devic, E. Magnier, F. Cuevas, G. Férey, P. L. Llewellyn and M. Latroche, J. Chem. Soc., Dalton Trans., 2011, 40, 4879–4881 RSC.
- K. Jayaramulu, F. Geyer, A. Schneemann, Š. Kment, M. Otyepka, R. Zboril, D. Vollmer and R. A. Fischer, Adv. Mater., 2019, 1900820 CrossRef.
- K. Jayaramulu, M. Horn, A. Schneemann, H. Saini, A. Bakandritsos, V. Ranc, M. Petr, V. Stavila, C. Narayana, B. Scheibe, Š. Kment, M. Otyepka, N. Motta, D. Dubal, R. Zbořil and R. A. Fischer, Adv. Mater., 2021, 33, 2004560 CrossRef CAS PubMed.
- M. E. Dmello, N. G. Sundaram and S. B. Kalidindi, Chem.–Eur. J., 2018, 24, 9220–9223 CrossRef CAS.
- K. R. Nemade and S. A. Waghuley, Indian J. Phys., 2014, 88, 577–583 CrossRef CAS.
- K. R. Nemade and S. A. Waghuley, Int. J. Mod. Phys.: Conf. Ser., 2013, 22, 380–384 CAS.
- K. R. Nemade and S. A. Waghuley, Opt. Mater., 2014, 36, 712–716 CrossRef CAS.
- B. Andò, S. Baglio, G. Di Pasquale, A. Pollicino, S. D'Agata, C. Gugliuzzo, C. Lombardo and G. Re, Procedia Eng., 2015, 120, 628–631 CrossRef.
- S. Muhammad Hafiz, R. Ritikos, T. J. Whitcher, N. Md. Razib, D. C. S. Bien, N. Chanlek, H. Nakajima, T. Saisopa, P. Songsiriritthigul, N. M. Huang and S. A. Rahman, Sens. Actuators, B, 2014, 193, 692–700 CrossRef.
- H. J. Yoon, D. H. Jun, J. H. Yang, Z. Zhou, S. S. Yang and M. M.-C. Cheng, Sens. Actuators, B, 2011, 157, 310–313 CrossRef CAS.
- A. D. Smith, K. Elgammal, X. Fan, M. C. Lemme, A. Delin, M. Råsander, L. Bergqvist, S. Schröder, A. C. Fischer, F. Niklaus and M. Östling, RSC Adv., 2017, 7, 22329–22339 RSC.
- K. R. Nemade and S. A. Waghuley, J. Electron. Mater., 2013, 42, 2857–2866 CrossRef CAS.
- M. G. Campbell, S. F. Liu, T. M. Swager and M. Dincă, J. Am. Chem. Soc., 2015, 137, 13780–13783 CrossRef CAS.
- M. K. Smith, K. E. Jensen, P. A. Pivak and K. A. Mirica, Chem. Mater., 2016, 28, 5264–5268 CrossRef CAS.
- M. G. Campbell, D. Sheberla, S. F. Liu, T. M. Swager and M. Dincă, Angew. Chem., Int. Ed., 2015, 54, 4349–4352 CrossRef CAS PubMed.
- M.-S. Yao, X.-J. Lv, Z.-H. Fu, W.-H. Li, W.-H. Deng, G.-D. Wu and G. Xu, Angew. Chem., Int. Ed., 2017, 56, 16510–16514 CrossRef CAS.
- M. E. Dmello, N. G. Sundaram, A. Singh, A. K. Singh and S. B. Kalidindi, Chem. Commun., 2019, 55, 349–352 RSC.
- E.-X. Chen, H.-R. Fu, R. Lin, Y.-X. Tan and J. Zhang, ACS Appl. Mater. Interfaces, 2014, 6, 22871–22875 CrossRef CAS.
- E.-X. Chen, H. Yang and J. Zhang, Inorg. Chem., 2014, 53, 5411–5413 CrossRef CAS PubMed.
Footnotes |
† Electronic supplementary information (ESI) available: Tables S1 and S2, general characterization details, gas sensing measurements setup and additional data on Raman spectroscopy. See DOI: 10.1039/d1ta03246a |
‡ K. J. and M. E. D. contributed equally to this work. |
|
This journal is © The Royal Society of Chemistry 2021 |
Click here to see how this site uses Cookies. View our privacy policy here.