DOI:
10.1039/D1TA01019K
(Paper)
J. Mater. Chem. A, 2021,
9, 19788-19795
Enhancing hydrogen production by photobiocatalysis through Rhodopseudomonas palustris coupled with conjugated polymers†
Received
3rd February 2021
, Accepted 31st March 2021
First published on 1st April 2021
Abstract
Utilizing solar energy for hydrogen production by combining light-activated materials and biocatalysts has become a promising alternative to fossil fuels. Herein, a feasible and simple bio-hybrid complex based on water-soluble oligofluorene (OF), polythiophene (PTP) and Rhodopseudomonas palustris (R. palustris), one kind of photosynthetic bacteria, was constructed for enhancing photocatalytic hydrogen production. Under the irradiation of visible light, there was fluorescence resonance energy transfer (FRET) between OF and PTP, which amplified photoelectron signals and transferred electrons via PTP to methyl viologen (MV2+), an electron mediator that established electron transfer pathways between organic materials and R. palustris. Hydrogen production involving R. palustris was catalyzed by nitrogenase. It is noteworthy that MV2+ was reduced to MV+˙ and transferred electrons to nitrogenase in R. palustris, converting protons and electrons to molecular hydrogen. Besides, triethanolamine (TEOA) was added as an electron donor to provide electrons to PTP. The proposed OF/PTP/MV2+/TEOA/R. palustris system provided a feasible strategy for photocatalytic hydrogen production with low-cost and easily implemented techniques.
Introduction
Photocatalytic hydrogen production is cost-effective for the storage of solar energy in chemical fuels, and it is a sustainable strategy to overcome energy shortages and environmental issues.1–3 Biohydrogen production methods are efficient hydrogen formation ways, and researchers have focused on the semi-artificial photosynthetic field coupling specific enzymes for biocatalysts and light-activated materials, such as inorganic semiconductors and molecular photosensitizers.4,5 These hybrid systems integrate effective solar energy conversion of materials and selective catalysis of enzymes, which has been widely applied for light-driven hydrogen production.6–8 For example, purified [FeFe]-hydrogenase or nitrogenase was modified on the surface of inorganic semiconductors (e.g., cadmium sulfide (CdS) and titanium dioxide (TiO2)) to realize photocatalytic hydrogen production.9 However, time-consumption and cumbersome operations to obtain purified enzymes hinder their practical application.10,11
The application of whole-cells is an emerging method that utilizes the photocatalytic activity of semiconductor materials and the stability of enzymes in microorganisms for photocatalytic hydrogen production.12 These biological hybrids avoid complicated genetic techniques and purification steps and are easily scaled up and widely adopted.13,14 For photocatalytic hydrogen production, such a cell-based strategy was constructed by the combination of TiO2, methyl viologen (MV2+) as an electron mediator, and recombinant Escherichia coli.15 MV2+ was required to collect photoelectrons and establish an electron transfer pathway in a cell-based photocatalytic hydrogen production hybrid composed of a water-soluble dye and Shewanella oneidensis.16 In addition, the hydrogen production efficiency can be increased by 90 times based on enhancing the reduction of MV2+ with an inorganic semiconductor in a bio-hybrid.17 Recently, a cell-based strategy has been achieved through the CdS nanoparticles coated on the surface of the photoheterotrophic bacterium, Rhodopseudomonas palustris (R. palustris), and this bio-hybrid produces hydrogen under visible light while producing other chemicals with nitrogenase.18 The classical methods of photocatalytic hydrogen production using bio-hybrids include direct or indirect photolysis, in which electron–hole separation depends on photocatalytic materials and the fermentation process using facultative anaerobic and obligate anaerobic bacteria.19 Photocatalytic materials are crucial factors in photocatalytic hydrogen production which determine the light absorption and the production of photoelectrons.20–22
Conjugated polymers (CPs) are polymers with a large delocalized π-conjugated backbone in the main chain, and show semiconductor properties and can be used as photosensitizers for light-induced hydrogen formation.23–25 Compared with photosensitive dyes and inorganic semiconductors, CPs have many excellent characteristics, such as good photoelectric properties in electrochemical devices, light harvesting, light stability for CO2 reduction, tunable energy levels in photovoltaic cells, and biocompatibility with organisms in biological applications.26–34 Owing to the outstanding properties of CPs, our group reported a CP-based bio-optical composite to augment photosynthesis of R. palustris.35 The proposed method opens up a feasible way to use CPs for photocatalytic hydrogen production.
In this work, we constructed an efficient bio-hybrid photocatalytic hydrogen production complex, in which water-soluble oligofluorene (OF) and polythiophene (PTP) serve as superior photosensitizers combined with R. palustris as a biocatalyst. Under the irradiation of visible light, there is fluorescence resonance energy transfer (FRET) between OF and PTP, which can amplify photoelectron signals and transfer electrons to microorganisms for hydrogen production. Moreover, MV2+ was added as an electron mediator in the complex to permeate cells and facilitate electron transfer between the OF/PTP pair and nitrogenase in R. palustris, and triethanolamine (TEOA) was used as a sacrificial electron donor. In the OF/PTP/MV2+/TEOA/R. palustris bioorganic complex, separated electrons of the OF/PTP pair continuously provided by TEOA were delivered to MV2+, and then MV2+ was reduced to blue MV+˙ under illumination. After that, MV+˙ transferred the electrons via the nitrogenase in intact R. palustris, making it accept more electrons to enhance hydrogen synthesis. This bio-hybrid system provided a fossil-fuel-free, efficient and clean way for hydrogen production through natural enzymes and organic photosensitizers (Scheme 1).
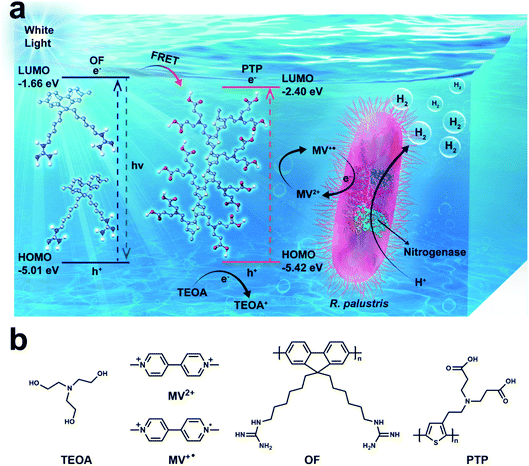 |
| Scheme 1 (a) Bio-hybrid photocatalytic hydrogen production complex. There are four parts in this system: OF and PTP as photosensitizers, MV2+ as an electron mediator, TEOA as an electron donor and R. palustris as a biocatalyst. (b) Chemical structures of TEOA, MV2+, MV+˙, OF and PTP. | |
Results and discussion
The preparation methods of PTP and OF have been reported in previous literature.36,37 Their excellent optical properties were attributed to thiophene units on PTP and fluorene units on OF, and their water solubility arose from carboxyl and guanidine groups in the side chains respectively. The absorption peak of OF in the visible light region was observed at 370 nm and that of PTP was observed at 410 nm. The emission peaks of OF were observed at 440 and 470 nm, and that of PTP was observed at 550 nm (Fig. 1a). There was a good overlap between the fluorescence emission spectrum of OF and the absorption spectrum of PTP, which satisfied the energy transfer conditions through the FRET mechanism.38,39 As shown in Fig. 1b, the fluorescence intensity of OF decreased gradually as the concentration of PTP increased, which indicated the energy transfer from OF to PTP. The ratio of the emission intensity at 440 nm and 550 nm (I440 nm/I550 nm) gradually increased and the fluorescence intensity of OF decreased by 12.8 times which was also verified by the trend of FRET from OF to PTP (ESI Fig. S1†). MV2+ as an electron mediator that was often used in artificial hydrogen producing systems was added in the complex to transfer electrons.40–42Fig. 1c shows that the fluorescence intensity of PTP was gradually quenched with the addition of MV2+ , indicating electron transfer from PTP to MV2+. The Stern–Volmer constant (Ksv) was calculated by measuring the fluorescence of PTP via the Stern–Volmer equation (eqn (1)).
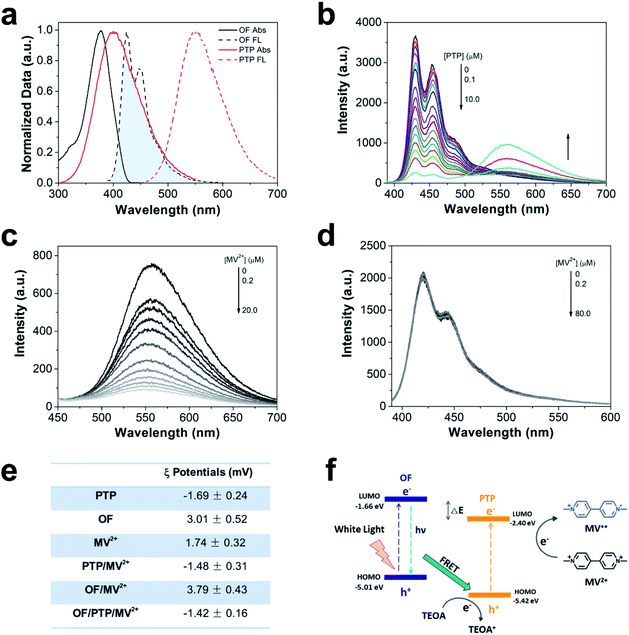 |
| Fig. 1 The characteristics of the OF/PTP/MV2+/TEOA complex. (a) Normalized UV-vis absorption spectra and fluorescence spectra of OF and PTP. (b) Fluorescence intensity of OF with increasing concentration of PTP. [OF] = 1 μM, [PTP] = 0–10.0 μM. (c) Fluorescence intensity of PTP with successive addition of MV2+. [PTP] = 10 μM, [MV2+] = 0–20 μM. (d) Fluorescence intensity of OF with successive addition of MV2+. [OF] = 1 μM, [MV2+] = 0–80 μM. (e) ζ potentials of PTP, OF, MV2+, PTP/MV2+, OF/MV2+ and OF/PTP/MV2+. [PTP] = 20 μM, [OF] = 20 μM, [MV2+] = 500 μM. (f) Schematic diagram of the electron transfer pathway of the OF/PTP/MV2+/TEOA complex under illumination. | |
Under low concentrations of MV2+ (0–1.0 μM), the Ksv value was 1.78 × 106 M−1 from the linear Stern–Volmer plot, indicating the quenching of the fluorescence intensity of PTP by MV2+ (ESI, Fig. S2†). However, there was no explicit interaction between MV2+ and OF with the increase of MV2+ concentration (Fig. 1d). Both OF and MV2+ have positive charge properties, leading to electrostatic repulsive interactions, which made it difficult for MV2+ to obtain electrons from OF. In contrast, negative charge properties of PTP made the electrons transfer from PTP to MV2+ more easily than from OF (Fig. 1e). As shown in ESI Fig. S3,† in the case of electron donor TEOA and CPs, the fluorescence of OF remained at the same level, while the fluorescence of PTP increased with the concentration of TEOA. In addition, the OF/PTP/MV2+/TEOA complex formed aggregates with a hydrodynamic diameter of about 700 nm, which was consistent with the OF/PTP/TEOA complex through dynamic light scattering (DLS) measured, indicating that MV2+ had no effect on its assembly in solution (ESI, Fig. S4†). The schematic diagram of energy transfer among the OF/PTP pair, TEOA and MV2+ is shown in Fig. 1f. Electron–hole separation occurred when OF and PTP acted as photosensitizers under light illumination. The HOMO and LOMO energy level offset between OF and PTP both exceeded 0.3 eV (ESI, Fig. S5†), which provided a sufficient driving force for the hole/electron dissociation of the OF/PTP pair.43 Due to the energy which is able to transfer from OF to PTP through FRET and the electrostatic interaction between PTP and MV2+, MV2+ was reduced to MV+˙ (blue) by separated electrons from the OF/PTP pair, and the electrons missing from the holes were supplied by TEOA. The OF/PTP/MV2+/TEOA complex established an efficient electron transfer pathway through FRET between OF and PTP to MV2+.
To deeply investigate the generation of electrons in the OF/PTP/MV2+/TEOA complex, the absorption of MV+˙ at 605 nm was determined under illumination for one hour. Compared with other complexes, the OF/PTP/MV2+/TEOA complex produced the most MV+˙ as shown in Fig. 2a. However, there were few MV+˙ ions produced by MV2+/TEOA and OF/PTP/MV2+/TEOA complexes. Owing to the transfer of electrons to MV2+ through the FRET between OF and PTP in the OF/PTP/MV2+/TEOA complex, the production of MV+˙ was greatly increased. To study the process of MV+˙ production in the OF/PTP/MV2+/TEOA complex, the UV-visible spectrum of the complex was measured under illumination for 20 min (Fig. 2b). Upon extension of the illumination time, the absorption of reduced MV+˙ at both 395 and 603 nm gradually increased and the solution changed from yellow to blue as a result of gradual accumulation of MV+˙. These results indicated that the OF/PTP pair satisfied the requirements of photosensitizers and could effectively reduce MV2+ to MV+˙.
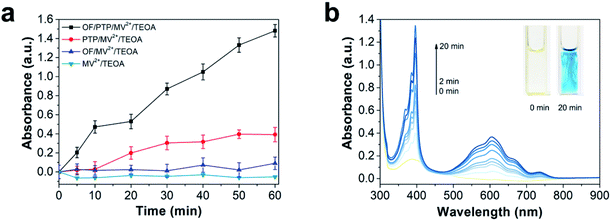 |
| Fig. 2 The production of MV+˙ in the OF/PTP/MV2+/TEOA complex under illumination conditions. (a) The absorption of MV+˙ at 605 nm in OF/PTP/MV2+/TEOA, PTP/MV2+/TEOA, OF/MV2+/TEOA and TEOA/MV2+ complexes under irradiation at 10 mW cm−2 for 1 h. (b) UV-visible spectra of the OF/PTP/MV2+/TEOA complex under irradiation at 10 mW cm−2 for 20 min; the light yellow line at 0 min, the dark blue line at 20 min; inset: photographs of the solution before and after irradiation for 20 minutes. [OF] = 20 μM, [PTP] = 20 μM, [MV2+] = 5 mM, 1% TEOA (v/v). The error bars show the standard deviation of three independent experiments. | |
It was documented that MV2+ acted as an electron mediator that permeated bacterial cells and established a pathway to transfer electrons from chemical reductants to microbial enzymes.44 Sodium dithionite, a chemical reductant that can easily reduce MV2+ to MV+˙, was used to evaluate whether MV+˙ could reduce enzymes of R. palustris to produce hydrogen. Sodium dithionite was added to a sealed 3 mL sample containing MV2+ and R. palustris filled with argon for 30 min to determine its hydrogen production. As the largest amount of hydrogen production strategy, the complex of sodium dithionite, MV2+ and R. palustris produced 624.80 nmol hydrogen in 30 min as shown in Fig. 3a. The proposed complex produced 247.01 nmol hydrogen in the absence of MV2+, while it could not generate hydrogen without R. palustris. These results implied that MV2+ was an effective promoter that transferred the acquired electrons to R. palustris and effectively increased the production of hydrogen. Here, R. palustris as a natural catalyst combined with OF and PTP as photosensitizers was used in the OF/PTP/MV2+/TEOA/R. palustris complex for photocatalytic hydrogen production. The proposed OF/PTP/MV2+/TEOA/R. palustris complex was able to produce the largest amount of hydrogen with irradiation for 2 h, while no hydrogen was generated in the absence of R. palustris as shown in Fig. 3b. In addition, illumination played a crucial role in the photocatalytic hydrogen production process using the OF/PTP/MV2+/TEOA/R. palustris complex, in which hydrogen production fell sharply in the absence of irradiation (ESI, Fig. S6†). Compared with other systems for hydrogen production with and without photosynthetic organisms (ESI, Table S1†), the OF/PTP/MV2+/TEOA/R. palustris complex exhibited some excellent characteristics, such as efficient hydrogen production, low-cost and simple operation. The results suggested the feasibility of the enzyme catalyzed for hydrogen production using the OF/PTP/MV2+/TEOA/R. palustris complex.
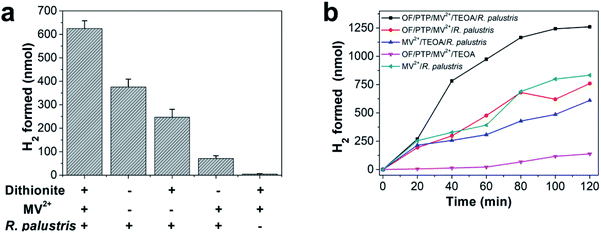 |
| Fig. 3 Hydrogen production of the OF/PTP/MV2+/TEOA/R. palustris complex. (a) Hydrogen production of dithionite, MV2+ and R. palustris in different complexes under anaerobic conditions for 30 min (“−” indicates that the complex had not been treated accordingly, and “+” indicates that the system had been treated under these conditions). (b) H2 production of different complexes under irradiation at 10 mW cm−2 for 120 min. Light intensity was 10 mW cm−2, [OF] = 20 μM, [PTP] = 20 μM, [MV2+] = 500 μM, 1% TEOA (v/v). The optical density at 660 nm in 10 mM phosphate-buffered saline (PBS) solution was 1. | |
Hydrogen production using the OF/PTP/MV2+/TEOA/R. palustris complex at different pHs and light intensities was deeply investigated. The influence of pH on hydrogen production using the OF/PTP/MV2+/TEOA/R. palustris complex is shown in Fig. 4a. It produced the largest amount of hydrogen at a pH of 7 under biocatalyst-friendly conditions. The hydrogen production decreased gradually as the pH value changed from 6 to 5 with the acidification of the solution. The changes in pH affected the transfer of electrons from MV+˙ to the enzyme and inhibited the biological enzyme activity of R. palustris. Under the conditions of pH 8 and pH 9, the decrease in hydrogen production was mainly due to the influence of the proton gradient on the R. palustris cell, which affects ATP synthesis of photophosphorylation. With the increase of light intensity, the hydrogen production of the bio-hybrid system gradually increased (Fig. 4b). When it reached 8 mW cm−2, the hydrogen production was the most. When the light intensity was further enhanced, the hydrogen production decreased gradually. The catalytic activity of R. palustris was destroyed by high light intensity. The hydrogen production process of electron transfer to R. palustris in the OF/PTP/MV2+/TEOA/R. palustris complex was subject to the regulation of enzyme activity by stimulus conditions.
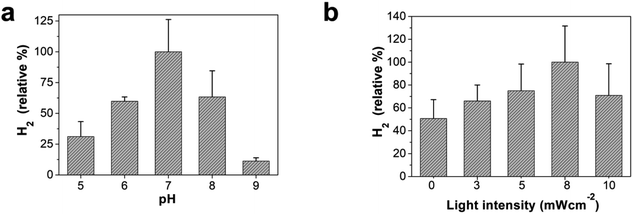 |
| Fig. 4 The effect of pH and light intensity on H2 production using the OF/PTP/MV2+/TEOA/R. palustris complex. (a) H2 production using the OF/PTP/MV2+/TEOA/R. palustris complex at different pH. (b) H2 production using the OF/PTP/MV2+/TEOA/R. palustris complex at different light intensities. [OF] = 20 μM, [PTP] = 20 μM, [MV2+] = 500 μM, 1% TEOA (v/v). The optical density at 660 nm in 10 mM PBS solution was 1. | |
The capability of the OF/PTP/MV2+/TEOA/R. palustris complex for photocatalytic hydrogen production is well established. In the process of hydrogen production involving R. palustris, nitrogenase reduced protons into molecular hydrogen and fixed molecular nitrogen.45,46Fig. 5a shows the diagram of hydrogen production by using the OF/PTP/MV2+/TEOA/R. palustris complex. MV+˙ transferred electrons to nitrogenase, and was oxidized to MV2+. Then the electrons and protons generated hydrogen under the catalysis of nitrogenase. In order to verify the mechanism of photocatalytic hydrogen production using the OF/PTP/MV2+/TEOA/R. palustris complex, the protein containing nitrogenase was extracted for subsequent experiments. The whole protein electrophoresis diagram of R. palustris is shown in ESI Fig. S7,† which illustrated the existence of nitrogenase in R. palustris. The different conditions of hydrogen production with nitrogenase, the OF/PTP/MV2+/TEOA complex and illumination were deeply investigated in Fig. 5b. Maximum hydrogen production was obtained by using the OF/PTP/MV2+/TEOA complex, reaching 48.49 nmol. In the absence of the OF/PTP/MV2+/TEOA complex or nitrogenase, hydrogen production was about 40% of that obtained by using the OF/PTP/MV2+/TEOA complex. Without illumination and in the absence of nitrogenase, the proposed OF/PTP/MV2+/TEOA complex could not produce any hydrogen. The results showed the feasibility of the light induction complex of OF/PTP/MV2+/TEOA to catalyze hydrogen production by using biological enzymes.
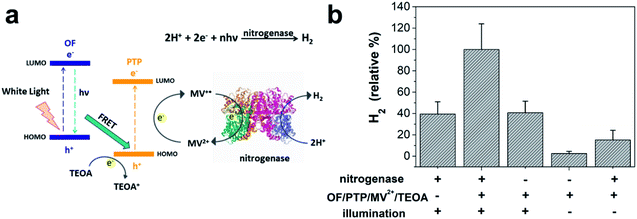 |
| Fig. 5 Hydrogen production with nitrogenase extracted from R. palustris. (a) Schematic diagram of H2 production using the OF/PTP/MV2+/TEOA/R. palustris complex. (b) Hydrogen production with nitrogenase, the OF/PTP/MV2+/TEOA complex and illumination under anaerobic conditions for 30 min (“−” indicates that the complex has not been treated accordingly, and “+” indicates that the complex has been treated under these conditions). The concentration of the crude protein of nitrogenase used in the experiment was 100 μg, light intensity was 10 mW cm−2, [OF] = 20 μM, [PTP] = 20 μM, [MV2+] = 500 μM, 1% TEOA (v/v). | |
Experimental methods
Materials
Two typical conjugated polymers PTP and OF were synthesized according to previous literature.47,48R. palustris (AS1.2352) was purchased from Beijing BeNa Culture Co., Ltd. (China). All other chemicals were purchased from Aladdin, Sigma and used as received.
Instruments
UV-vis absorption spectra were measured on a SPECORD 250 PLUS (Germany). Fluorescence spectra were measured on a Hitachi F-4600 fluorescence spectrophotometer (Japan). Dynamic light scattering (DLS) and ζ potentials were measured using a Malvern Nano-ZS90 (UK). The irradiation source of visible light was a MEJIRO GENOSSEN MVL-210 (Japan). Electrochemical measurements were performed on a CH Instruments CHI600E (China). The analysis of hydrogen production was performed on a CEAULIGHT GC-7900 gas chromatograph with argon as the carrier gas. The pH was measured using a Sartorius PB-10 (Germany).
Concentration of CPs
[CPs] = mcp/(Mcp × Vsolution) |
[CPs] is the concentration of CPs, mcp is the mass of CPs, Mcp is the molar mass of the repeating unit in CPs, and Vsolution is the volume of the solution.
Detection of ζ potential
20 μM PTP, 20 μM OF and 500 μM MV2+ aqueous solution was prepared for ζ potential measurements. Then aqueous solutions of PTP/MV2+, OF/MV2+ and OF/PTP/MV2+ complexes with the above concentrations were prepared as well. The potential was measured at 25 °C for 120 s, and the average was obtained three times to obtain the zeta potential value.
DLS measurements
The solutions of OF/PTP/TEOA and OF/PTP/MV2+/TEOA complexes were prepared for size measurements. The concentrations involved in the above systems design were 20 μM PTP, 20 μM OF, 500 μM MV2+ and 1% (v/v) TEOA. All experiments were performed at 25 °C for 120 s, and the average was obtained three times to obtain the size value.
Fluorescence quenching experiments
The fluorescence spectrum of PTP (10 μM) solution with the increase of the concentration of MV2+ (0–20 μM) was measured. Then the fluorescence spectrum of OF (1 μM) solution with the increase of the concentration of MV2+ (0–80 μM) was measured. The excitation maxima of PTP and OF were at 390 nm and 420 nm respectively.
Detection of MV+˙ accumulation
A mixed solution containing PTP (20 μM), OF (20 μM), TEOA (v/v = 1%) and MV2+ (5 mM) was placed in a special quartz cell and covered with a sealing rubber stopper. The solution was bubbled with argon gas for 30 min to remove oxygen in the system under 10 mW cm−1 irradiation. The UV-vis absorption spectrum of the solution was measured every 10 min until the accumulated light time reached the end of 2 hours. All the experiments were repeated three times.
R. palustris culture
This strain was grown on 112 van Niel's yeast agar49 under the conditions of 1.0 g of K2HPO4, 0.5 g of MgSO4, 10.0 g of yeast extract, 1000 mL of distilled water, and pH 7.0–7.4. Argon gas was pumped into the medium for exhausting the air, and then the inoculated culture solution (15% (v/v)) was added to a 20 mL serum bottle. The culture solution was placed in an incubator under anaerobic illumination for 5 days at a temperature of 30 °C until the culture solution turned dark red. The optical density at 660 nm with PBS solution (10 mM) was used as the biological index in the following experiments.50
Hydrogen generation using dithionite
Hydrogen production using dithionite (300 mg L−1), MV2+ (500 μM) and R. palustris in different complexes was measured under anaerobic conditions for 30 min. Then the hydrogen production was determined by gas chromatography through 200 μL of the upper gas. The amount of hydrogen produced was calculated using H2 normalized curves. All the experiments were repeated three times.
Hydrogen generation measurement using different complexes
The solutions of MV2+/R. palustris, MV2+/TEOA/R. palustris and OF/PTP/TEOA, OF/PTP/MV2+/TEOA/R. palustris complexes were sealed in Pyrex bottles, bubbled with argon gas for 30 minutes and irradiated with 10 mW cm−1 for 2 h. Every 20 min 200 μL of the upper gas of the reactor was injected into a gas chromatograph to measure hydrogen production for 2 h. Moreover, the hydrogen production of the OF/PTP/MV2+/TEOA/R. palustris solution without illumination was also studied. The amount of hydrogen produced was calculated using H2 normalized curves. All the experiments were repeated three times.
Hydrogen generation using a bio-hybrid complex at different pHs
The pH of the bio-hybrid complex was adjusted with NaOH and HCl. Mixed solutions consisting of 20 μM PTP, 20 μM OF, 500 μM MV2+, 1% (v/v) TEOA and a suspension of R. palustris (OD660 = 1.0) were sealed in Pyrex bottles and bubbled with argon gas for 30 min. The mixed solutions of different pH (5, 6, 7, 8 and 9) were irradiated with 10 mW cm−1 for 1 h. 200 μL of the upper gas of the reactor was injected into a gas chromatograph to measure hydrogen production. The amount of hydrogen produced was calculated by using H2 normalized curves. All the experiments were repeated three times.
Hydrogen generation using a bio-hybrid complex at different light intensities
Mixed solutions consisting of 20 μM PTP, 20 μM OF, 500 μM MV2+, 1% (v/v) TEOA and a suspension of R. palustris (OD660 = 1.0) were sealed in Pyrex bottles and bubbled with argon gas for 30 min. The mixed solutions were irradiated for 1 h with different light intensities (0, 3, 5, 8, 10 mW cm−2) . 200 μL of the upper gas of the reactor was injected into the gas chromatograph to measure hydrogen production. The amount of hydrogen produced was calculated by using H2 normalized curves. All the experiments were repeated three times.
H2 normalized curve
Standard H2, 4.9% (v/v) H2 in argon, was chosen as a balance gas. Two needles of different lengths were inserted into a 200 mL glass bottle with a stopper. The long needle was connected to the gas cylinder and acted as an air inlet, and the small needle was inserted into the rubber stopper to provide an air outlet. Gas exchange was performed in the glass bottle for 30 min. 20, 40, 60, 80, 100 and 120 μL of gas were injected into the GC respectively, and each one for three times. The relationship between the H2 peak area and the number of moles of hydrogen follows the equation y = 3.23 × 106x2 + 97
796x by fitting.
Cyclic voltammetry measurements
Cyclic voltammetry (CV) measurements were carried out using a three-electrode system with a glassy carbon electrode as the working electrode, platinum wire as the counter electrode, and a Ag/AgCl electrode as the reference electrode. 2 mg PTP or OF was added into 2 mL 0.1 M tetrabutylammonium hexafluorophosphate in anaerobic acetonitrile solution to obtain electrochemical properties and the energy levels of PTP and OF were calculated at a scan rate of 0.05 V s−1. Eg = (1240/λonset) eV, EHOMO = −(Eox + 4.40) eV, ELOMO = EHOMO + Eg.
Protein extraction
To prepare the protein of R. palustris, the culture was harvested under anaerobic conditions by centrifugation at 8000 rpm for 5 min, and then it was washed with 1× PBS 3 times. The suspension was treated by the ultrasonic disruption method to obtain the protein of R. palustris. The precipitate was discarded after centrifugation at 3000 rpm for 30 min, and the supernatant contained nitrogenase protein. Protein concentrations were analyzed by the Biuret assay using BSA as the standard. The nitrogenase protein was assessed by SDS-PAGE analysis with Coomassie G-250.
Conclusions
We have established a feasible bio-hybrid complex for hydrogen production, which was based on four solid foundations: PTP and OF as water-soluble photosensitizers, MV2+ as an electron mediator, TEOA as an electron donor and R. palustris as a biocatalyst. Under the illumination of visible light, FRET between OF and PTP amplified the photoelectron signals and transferred electrons to MV2+. MV2+ was added as an electron mediator in the complex to permeate cells and facilitate electron transfer between the OF/PTP pair and R. palustris. The hydrogen production of the bio-hybrid complex reached 0.63 μmol h−1, which was 8.1 times higher than that of the OF/PTP/MV2+/TEOA complex. The biocatalytic activity of R. palustris was the main factor in hydrogen production using the bio-hybrid complex and it was influenced by the change of pH and light intensity. Moreover, it has been confirmed that nitrogenase in R. palustris played a key role in hydrogen production using R. palustris as a biocatalyst. This approach provided a simple and efficient strategy for visible light-driven hydrogen production using bio-hybrid complexes of organic semiconductors and microorganisms.
Conflicts of interest
There are no conflicts to declare.
Acknowledgements
The authors are grateful to Prof. Shu Wang from Key Laboratory of Organic Solids, Institute of Chemistry, Chinese Academy of Sciences and Dr Huan Lu from Peking University (Beijing, China). The authors are also grateful for the financial support from the National Natural Science Foundation of China (no. 21773054, 21905072, 22077025 and 51803046) and the Natural Science Foundation of Hebei Province (no. B2020202062 and B2020202086).
Notes and references
- S. F. Sousa, B. L. Souza, C. L. Barros and A. O. T. Patrocinio, Int. J. Photoenergy, 2019, 2019, 23 Search PubMed
.
- A. M. Abdalla, S. Hossain, O. B. Nisfindy, A. T. Azad, M. Dawood and A. K. Azad, Energy Convers. Manage., 2018, 165, 602–627 Search PubMed
.
- G. Glenk and S. Reichelstein, Nat. Energy, 2019, 4, 216–222 Search PubMed
.
- Y. Honda, Y. Shinohara and H. Fujii, Catal. Sci. Technol., 2020, 10, 6006–6012 Search PubMed
.
- S. H. Lee, D. S. Choi, S. K. Kuk and C. B. Park, Angew. Chem., Int. Ed., 2018, 130, 8086–8116 Search PubMed
.
- H. Tong, S. Ouyang, Y. Bi, N. Umezawa, M. Oshikiri and J. Ye, Adv. Mater., 2012, 24, 229–251 Search PubMed
.
- A. A. Ismail and D. W. Bahnemann, Sol. Energy Mater. Sol. Cells, 2014, 128, 85–101 Search PubMed
.
- Y. Ding, J. R. Bertram, C. Eckert, R. R. Bommareddy, R. Patel, A. Conradie, S. Bryan and P. Nagpal, J. Am. Chem. Soc., 2019, 141, 10272–10282 Search PubMed
.
- K. A. Brown, M. B. Wilker, M. Boehm, G. Dukovic and P. W. King, J. Am. Chem. Soc., 2012, 134, 5627–5636 Search PubMed
.
- E. A. Vasconcelos, R. C. Leitão and S. T. Santaella, BioEnergy Res., 2016, 9, 1260–1271 Search PubMed
.
-
S. W. M. Kengen, H. P. Goorissen, M. Verhaart, E. W. J. van Niel, P. A. M. Claassen and A. J. M. Stams, Biological hydrogen production by anaerobic microorganisms, Biofuels, ed. W. Soetaert, and E. J. Vandamme, John Wiley and Sons, 2009, pp. 197–221 Search PubMed
.
- B. Ramprakash and A. Incharoensakdi, Bioresour. Technol., 2020, 318, 124057 Search PubMed
.
- S. Cestellos-Blanco, H. Zhang, J. M. Kim, Y. X. Shen and P. D. Yang, Nat. Catal., 2020, 3, 245–255 Search PubMed
.
- Y. C. Ding, J. R. Bertram, C. Eckert, R. R. Bommareddy, R. Patel, A. Conradie, S. Bryan and P. Nagpal, J. Am. Chem. Soc., 2019, 141, 10272–10282 Search PubMed
.
- Y. Honda, H. Hagiwara, S. Ida and T. Ishihara, Angew. Chem., Int. Ed., 2016, 55, 8045–8048 Search PubMed
.
- S. F. Rowe, G. N. L. Le Gall, E. V. Ainsworth, J. A. Davies, C. W. Lockwood, L. Shi, A. Elliston, I. N. Roberts, K. W. Waldron and D. Richardson, ACS Catal., 2017, 7, 7558–7566 Search PubMed
.
- Y. Honda, M. Watanabe, H. Hagiwara, S. Ida and T. Ishihara, Appl. Catal., B, 2017, 210, 400–406 Search PubMed
.
- B. Wang, K. M. Xiao, Z. F. Jiang, J. F. Wang, J. C. Yu and P. K. Wong, Energy Environ. Sci., 2019, 12, 2185–2191 Search PubMed
.
- W. Wei, P. Q. Sun, Z. Li, K. S. Song, W. Y. Su, B. Wang, Y. Z. Liu and J. Zhao, Sci. Adv., 2018, 4, eaap9253 Search PubMed
.
- J. L. Guo, M. Suastegui, K. K. Sakimoto, V. M. Moody, G. Xiao, D. G. Nocera and N. S. Joshi, Science, 2018, 362, 813–816 Search PubMed
.
- L. Su and C. M. Ajo-Franklin, Curr. Opin. Biotechnol., 2019, 57, 66–72 Search PubMed
.
- W. Lubitz, H. Ogata, O. RüDiger and E. Reijerse, Chem. Rev., 2014, 114, 4081–4148 Search PubMed
.
- S. Yanagida, A. Kabumoto, K. Mizumoto, C. Pac and K. Yoshino, J. Chem. Soc., Chem. Commun., 1985, 474–475 Search PubMed
.
- W. W. Yong, H. Lu, H. Li, S. Wang and M. T. Zhang, ACS Appl. Mater. Interfaces, 2018, 10, 10828–10834 Search PubMed
.
- T. M. Swager, Acc. Chem. Res., 1998, 31, 201–207 Search PubMed
.
- H. Lu, R. Hu, H. Bai, H. Chen, F. Lv, L. Liu, S. Wang and H. Tian, ACS Appl. Mater. Interfaces, 2017, 9, 10355–10359 Search PubMed
.
- Y. Xiang, X. Wang, L. Rao, P. Wang, D. Huang, X. Ding, X. Zhang, S. Wang, H. Chen and Y. Zhu, ACS Energy Lett., 2018, 3, 2544–2549 Search PubMed
.
- L. Feng, L. Hui, Y. Qu and C. Lv, Chem. Commun., 2012, 43, 4633–4635 Search PubMed
.
- H. B. Yuan, Y. Zhan, A. E. Rowan, C. F. Xing and P. H. J. Kouwer, Angew. Chem., Int. Ed., 2020, 59, 2720–2724 Search PubMed
.
- P. Gai, W. Yu, H. Zhao, R. Qi, F. Li, L. Liu, F. Lv and S. Wang, Angew. Chem., Int. Ed., 2020, 59, 7224–7229 Search PubMed
.
- X. Zhou, Y. Zeng, Y. Y. Tang, Y. M. Huang, F. T. Lv, L. B. Liu and S. Wang, Sci. Adv., 2020, 6, eabc5237 Search PubMed
.
- R. Chai, C. F. Xing, J. J. Qi, Y. B. Fan, H. B. Yuan, R. M. Niu, Y. Zhan and J. L. Xu, Adv. Funct. Mater., 2016, 26, 9026–9031 Search PubMed
.
- Y. X. Wang, S. L. Li, L. B. Liu, F. T. Lv and S. Wang, Angew. Chem., Int. Ed., 2017, 56, 5308–5311 Search PubMed
.
- J. Y. Lin, B. Liu, M. N. Yu, C. J. Ou, Z. F. Lei, F. Liu, X. H. Wang, L. H. Xie, W. S. Zhu, H. F. Ling, X. W. Zhang, P. N. Stavrinou, J. P. Wang, D. D. C. Bradley and W. Huang, J. Mater. Chem. C, 2017, 5, 6762–6770 Search PubMed
.
- Z. Wang, D. Gao, Y. Zhan and C. Xing, ACS Appl. Bio Mater., 2020, 3, 3423–3429 Search PubMed
.
- H. Chen, B. Wang, J. Zhang, C. Nie, F. Lv, L. Liu and S. Wang, Chem. Commun., 2015, 51, 4036–4039 Search PubMed
.
- C. Xing, Q. Xu, H. Tang, L. Liu and S. Wang, J. Am. Chem. Soc., 2009, 131, 13117–13124 Search PubMed
.
- B. T. Bajar, E. S. Wang, S. Zhang, M. Z. Lin and J. Chu, Sensors, 2016, 16, 1488 Search PubMed
.
- H. Swaminathan and K. Balasubramanian, Sens. Actuators, B, 2018, 264, 337–343 Search PubMed
.
- B. Chica, C. H. Wu, Y. Liu, M. W. W. Adams, T. Q. Lian and R. B. Dyer, Energy Environ. Sci., 2017, 10, 2245–2255 Search PubMed
.
- H. Kotani, T. Ono, K. Ohkubo and S. Fukuzumi, Phys. Chem. Chem. Phys., 2007, 9, 1487–1492 Search PubMed
.
- H. Zhu, N. Song, H. Lv, C. L. Hill and T. Lian, J. Am. Chem. Soc., 2012, 134, 11701–11708 Search PubMed
.
- M. C. Scharber, D. Mühlbacher, M. Koppe, P. Denk, C. Waldauf, A. J. Heeger and C. J. Brabec, Adv. Mater., 2006, 18, 789–794 Search PubMed
.
- P. Maruthamuthu, S. Muthu, K. Gurunathan, M. Ashokkumar and M. V. C. Sastri, Int. J. Hydrogen Energy, 1992, 17, 863–866 Search PubMed
.
- Y. Zheng and C. S. Harwood, Appl. Environ. Microbiol., 2019, 85, e02671 Search PubMed
.
- E. K. Heiniger, Y. Oda, S. K. Samanta and C. S. Harwood, Appl. Environ. Microbiol., 2012, 78, 1023–1032 Search PubMed
.
- G. X. Feng, C. Y. Tay, Q. X. Chui, R. R. Liu, N. Tomczak, J. Liu, B. Z. Tang, D. Leong and B. Liu, Biomaterials, 2014, 35, 8669–8677 Search PubMed
.
- L. H. Feng, L. B. Liu, F. T. Lv, G. C. Bazan and S. Wang, Adv. Mater., 2014, 26, 3926–3930 Search PubMed
.
- N. Kamal, V. Sabaratnam, N. Abdullah, A. S. H. Ho, S. H. Teo, H. B. Lee and A. V. Leeuw, J. Microbiol., 2009, 95, 179–188 Search PubMed
.
- D. Muzziotti, A. Adessi, C. Faraloni, G. Torzillo and R. D. Philippis, Microbiol. Res., 2017, 197, 49–55 Search PubMed
.
Footnote |
† Electronic supplementary information (ESI) available. See DOI: 10.1039/d1ta01019k |
|
This journal is © The Royal Society of Chemistry 2021 |
Click here to see how this site uses Cookies. View our privacy policy here.