DOI:
10.1039/D1SC02965G
(Edge Article)
Chem. Sci., 2021,
12, 13704-13710
Chromophore-radical excited state antiferromagnetic exchange controls the sign of photoinduced ground state spin polarization†
Received
2nd June 2021
, Accepted 1st September 2021
First published on 9th September 2021
Abstract
A change in the sign of the ground-state electron spin polarization (ESP) is reported in complexes where an organic radical (nitronylnitroxide, NN) is covalently attached to a donor–acceptor chromophore via two different meta-phenylene bridges in (bpy)Pt(CAT-m-Ph-NN) (mPh-Pt) and (bpy)Pt(CAT-6-Me-m-Ph-NN) (6-Me-mPh-Pt) (bpy = 5,5′-di-tert-butyl-2,2′-bipyridine, CAT = 3-tert-butylcatecholate, m-Ph = meta-phenylene). These molecules represent a new class of chromophores that can be photoexcited with visible light to produce an initial exchange-coupled, 3-spin (bpy˙−, CAT+˙ = semiquinone (SQ), and NN), charge-separated doublet 2S1 (S = chromophore excited spin singlet configuration) excited state. Following excitation, the 2S1 state rapidly decays to the ground state by magnetic exchange-mediated enhanced internal conversion via the 2T1 (T = chromophore excited spin triplet configuration) state. This process generates emissive ground state ESP in 6-Me-mPh-Pt while for mPh-Pt the ESP is absorptive. It is proposed that the emissive polarization in 6-Me-mPh-Pt results from zero-field splitting induced transitions between the chromophoric 2T1 and 4T1 states, whereas predominant spin–orbit induced transitions between 2T1 and low-energy NN-based states give rise to the absorptive polarization observed for mPh-Pt. The difference in the sign of the ESP for these molecules is consistent with a smaller excited state 2T1 – 4T1 gap for 6-Me-mPh-Pt that derives from steric interactions with the 6-methyl group. These steric interactions reduce the excited state pairwise SQ-NN exchange coupling compared to that in mPh-Pt.
Introduction
Molecular quantum information science (QIS) requires the synthesis and study of new molecules that can enable the generation, manipulation, and readout of specifically prepared quantum states1 in order to advance new nanoscale technologies that include, computing,2–5 sensing,6,7 and communications.8 QIS exploits quantum bits, or qubits, that are unlike two-level classical bits (e.g., binary 0 and 1) since they can be entangled and exist as superposition states described by linear combinations of these 0 and 1 binary representations.1,9 An unpaired electron spin (S = 1/2, ms ±1/2) represents such a two-level quantum system that can be created with relative ease in a molecular environment. However, expanding such a system to include multiple unpaired spins and generating the desired coherences within the system represents a significant challenge.
Building on our earlier work,10–16 we have shown that photoexcitation into the low-energy ligand-to-ligand charge transfer (LL′CT) bands of bipyridine-metal-catecholate ((bpy)M(CAT)) complexes with M = Pt or Pd provide a convenient way to generate charge transfer excited states with considerable biradical spin character.11–13,16,17 When these molecules are elaborated with a persistent radical attached to the CAT donor, the LL′CT excited states can be described as having three localized spin qubits that possess different pairwise exchange interactions.16,17 A particular benefit of this tripartite entanglement9,18–20 is that when the spins in the open-shell excited singlet- (S) and triplet (T) states of the chromophore exchange couple with the radical, two doublet states (2S1 and 2T1) and a quartet state (4T1) result, and magnetic exchange interactions admix the 2S1 and 2T1 states.17 This excited state exchange mixing effectively mediates an enhanced intersystem crossing21–24 that permits access to the localized high-spin triplet configuration of the chromophore. The triplet state of the (bpy)M(CAT) parent chromophore is otherwise inaccessible due to non-competitive intersystem crossing (ISC) rates compared to efficient non-radiative relaxation back to the singlet ground state.13,15
Chromophores with appended radicals have been used to provide high-resolution information regarding the nature of their low-energy excited states,11,12,16,17 illustrate how multiple, pairwise, excited state magnetic exchange interactions influence photophysical processes,11 and highlight how the excited state chromophore triplet – radical magnetic exchange interaction and associated excited state dynamics can affect electron spin polarization (ESP).11,16,17,22,23,25–34 In most systems of this type, ESP is a result of the spin selectivity of the transitions between nearly degenerate 2T1 and 4T1 states and, in the absence of intermolecular collisions, ESP is observed when these states are relatively long lived. These radical-elaborated chromophores possess spin-polarized quartet 4T1 excited states with lifetimes that compete with or exceed the spin-lattice relaxation time (T1) of the appended radical, and this effectively inhibits the transfer of ESP to the electronic ground state. Although the observation of ground state ESP is rare in glassy matrices, a few examples are known and they occur via the reversed quartet mechanism (RQM, Fig. 1).35,36
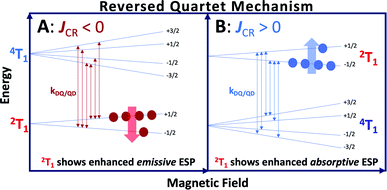 |
| Fig. 1 Left (A): reversed quartet mechanism (RQM), antiferromagnetic JCR. Spin-selective transitions (kQD/DQ) between doublet and quartet result in non-Boltzmann population (red dots) of the doublet ms levels (non-Boltzmann population of quartet not shown) resulting in enhanced emissive ESP. Right (B): RQM, ferromagnetic JCR. Spin-selective transitions (kQD/DQ) between doublet and quartet states result in non-Boltzmann population (blue dots) of the doublet ms levels (non-Boltzmann population of quartet not shown) resulting in enhanced absorptive ESP. | |
Electron spin polarization,23,37,38 including that generated by RQM,35,36 derives from non-Boltzmann ms sublevel populations (Fig. 1) and can be detected using time resolved electron paramagnetic resonance (TREPR) spectroscopy as either an enhanced emissive (Fig. 1A) or absorptive (Fig. 1B) signal. An emissive TREPR signal via the RQM requires the 4T1 state to be higher in energy than 2T1 (and ΔED1Q ∼ kBT where kB is Boltzmann's constant and T is temperature). In this case, spin selective transitions (kQD/DQ, Fig. 1) between the 2T1 and 4T1 states result in an excess population of the higher energy ms = +1/2 sublevel of the doublet and enhanced emissive ESP. Conversely, an absorptive TREPR signal via the RQM (Fig. 1B) requires the 4T1 quartet state to be lower in energy than the 2T1 doublet (and ΔE ∼ kBT). Here, spin selective transitions (kQD/DQ, Fig. 1) between 2T1 and 4T1 result in an excess population of the lower-energy ms = −1/2 level of the doublet and enhanced absorptive ESP. This photogenerated 2T1 ESP can then be transferred to the ground state if 2T1 → 2S0 relaxation is faster than the T1 spin relaxation of the appended radical in the 2S0 state. A key point of the RQM is that the sign of the exchange parameter determines the relative energy ordering of the 2T1 and 4T1 states, and whether the observed ESP will be absorptive or emissive.35,36 This mechanism predicts that antiferromagnetic chromophore-radical exchange (JCR <0), with E(2T1) < E(4T1), results in emissive ESP (Fig. 1A), while ferromagnetic chromophore-radical exchange (JCR >0), with E(4T1) < E(2T1), yields absorptive ESP (Fig. 1B).35,36
Recently, we demonstrated that despite the short 2T1 excited state lifetime and absence of intermolecular collisions, strong ground state ESP can be generated in (bpy)Pt(CAT-m-Ph-NN) (mPh-Pt, NN = nitronylnitroxide radical).16 The intensity of the photoinduced ESP in mPh-Pt was also shown to be dramatically altered by changing the metal ion from Pt to Pd in what are otherwise identical complexes.16 In the present work, we show that the nature of the ground state ESP is also related to the magnitude of the excited state chromophore-radical antiferromagnetic exchange (JSQ-NN (= 2JCR) Fig. 2A), which can be controlled by tailored bond torsions that reduce π-system mediated superexchange coupling. Thus the sign of the photogenerated ESP is not determined by changing the sign of JSQ-NN, as predicted by the RQM.35,36 Here, changing the magnitude of the excited state exchange affects how the coupled chromophore-radical 4T1–2T1 states interact with localized states of the radical (e.g., DNN, QNN, vide infra), suggesting a new mechanism for modulating the sign of ground state ESP.
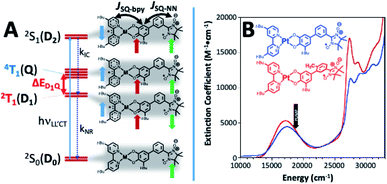 |
| Fig. 2 (A) (Left) ground doublet- (2S0/D0) and LL′CT excited state manifold. Vertical doublet 2S1(D2) undergoes rapid internal conversion (kIC) to 2T1(D1), which has (bpy)Pt(CAT) triplet character. Nonradiative decay of the 2T1 state (kNR) provides the 2S0 ground state with non-Boltzmann ms populations. (Right) depictions of ground- (2S0/D0), and LL′CT excited states; magnetic exchange coupling parameters, JSQ-bpy and JSQ-NN defined; JSQ-NN is related to JCR in Fig. 1. (B) Electronic absorption spectra (300 K; CH2Cl2), for mPh-Pt (blue line) and 6-Me-mPh-Pt (red line), indicating TREPR photoexcitation energy relative to the ∼17 000 cm−1 2S0(D0) → 2S1(D2) LL′CT transition | |
(Bipyridine)PtII(catecholate-bridge-radical) complexes
The (bpy)M(CAT-Ph-NN) chromophoric system (Fig. 2A and B)11,16,17 offers a synthetically flexible platform to explore magnetic exchange contributions to both the sign and magnitude of the photoinduced ground state ESP. Fig. 2B shows the virtually identical solution electronic absorption spectra of mPh-Pt and (bpy)Pt(CAT-6-Me-m-Ph-NN) (6-Me-mPh-Pt), where the characteristic low-energy CAT(HOMO) → bpy(LUMO) ligand-to-ligand charge transfer (LL′CT) transitions are observed at ∼17
000 cm−1.11,15–17 Interestingly, localized aryl-NN π→π* transitions are also observed in this spectral region,39,40 but they are obscured in mPh-Pt and 6-Me-mPh-Pt by the more intense LL′CT band.16 Despite the remarkably similar electronic absorption spectra observed for (bpy)Pt(CAT-bridge-NN) complexes,17 the nature of the magnetic exchange coupling between spins in the excited states of (bpy)Pt(CAT-bridge-NN) systems can dramatically affect excited state processes when compared to the parent (bpy)Pt(CAT) chromophore that lacks an appended radical.11 This was previously exemplified by the observation of both magnetic circular dichroism (MCD) activity,17 and a dramatic modulation of excited state lifetimes in (bpy)Pt(CAT-bridge-NN) molecules.11
Optical excitation into the mPh-Pt and 6-Me-mPh-Pt spin-allowed 2S0 → 2S1 LL′CT transition leads to a high degree of CAT → bpy charge separation, and a bpy1CAT1NN1 (i.e., a bpy˙SQ˙NN˙ tri-radical; SQ = semiquinone; hereafter “˙” is omitted) electronic configuration that has 2S1, 2T1, and 4T1 excited states (Fig. 2A and B).17 The cross-conjugated meta-phenylene bridges in mPh-Pt and 6-Me-mPh-Pt promote an antiferromagnetic chromophore – radical exchange interaction JSQ-NN, Fig. 2A,41 with the primary consequence being that the 2T1 state resides at lower energy than the high-spin 4T1 quartet excited state (Fig. 1A and 2A). Moreover, the spatial separation of the unpaired spins in the LL′CT state leads to large differences in the pairwise magnetic exchange couplings to the NN radical, which results in mixing of the 2S1 and 2T1 states, and ultrafast enhanced intersystem crossing (EISC)21,22,24 from the 2S1 to the 2T1 state. Thus, photoexcitation followed by EISC (kIC, Fig. 2A) results in 2S0 → 2S1 → 2T1 (Fig. 2A) for both mPh-Pt and 6-Me-mPh-Pt. Fast non-radiative decay (kNR, Fig. 2A) of the 2T1 state yields the 2S0 ground state.11,16,17
The effect of bond torsions on excited state exchange
The key structural difference between mPh-Pt and 6-Me-mPh-Pt is a single methyl substituent on the meta-phenylene bridge fragment of 6-Me-mPh-Pt that results in increased CAT-bridge bond torsion. As seen in Fig. 2B, the CAT-bridge bond torsion results in modest changes in the LL′CT band by attenuating CAT-bridge delocalization.10 The increased CAT-bridge torsion reduces the LL′CT excited state SQ-mPh-NN π-overlap relative to that of mPh-Pt.10,14 This reduction in π-overlap decreases the magnitude of JSQ-NN in the LL′CT excited state of 6-Me-mPh-Pt so that ΔED1Q(6-Me-mPh-Pt) < ΔED1Q(mPh-Pt) (Fig. 2A). An experimentally-derived estimate of JSQ-NN for the LL′CT excited state of 6-Me-mPh-Pt is obtained from JSQ-NN in the ground state biradical ligand of LZnSQ-6-Me-mPh-NN (Fig. 3). The SQ-6-Me-mPh-NN linkage in LZnSQ-6-Me-mPh-NN is, by analogy, the two-spin donor half of the charge separated LL′CT excited state for 6-Me-mPhPt.
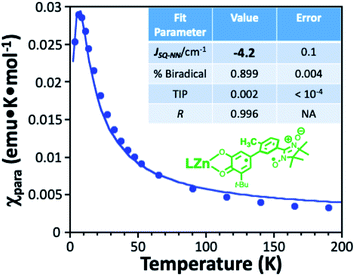 |
| Fig. 3 Variable-temperature paramagnetic susceptibility data for LZn(SQ-6-Me-mPh-NN) (L = hydro-tris(cumenyl, methyl-pyrazolyl)borate), see ESI† for details. The best fit of eqn (2) to the data yields JSQ-NN = −4 cm−1. | |
The magnitude of the antiferromagnetic JSQ-NN mediated by an unsubstituted m-Ph bridge has previously been determined from solid state magnetic susceptibility measurements on LZnSQ-mPh-NN (JSQ-NN = −32 cm−1).41 The same Heisenberg-Dirac-Van Vleck spin Hamiltonian (eqn (1)) is used here to describe the exchange split singlet and triplet components of the LZnSQ-6-Me-mPh-NN ground state. The corresponding expression for the magnetic susceptibility is given by eqn (2), which is used to determine the singlet-triplet energy gap (ΔS–T = −2JSQ-NN) for LZn(SQ-6-Me-mPh-NN). In eqn (2), x is percentage of the biradical that contributes to the susceptibility, (1 − x) is the mole fraction of the monoradical impurity, and TIP is the temperature-independent paramagnetism.42,43
| 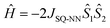 | (1) |
|  | (2) |
The ΔS–T value that we obtain by fitting eqn (2) to the magnetic susceptibility data (Fig. 3) for LZn(SQ-6-Me-mPh-NN) is ΔS-T = 8.0 cm−1 (JSQ-NN = −4.0 cm−1), which corresponds to an 87% decrease in the magnetic exchange coupling relative to LZn(SQ-mPh-NN).10,44 For conjugated systems, the attenuation of π-type superexchange coupling between SQ and NN is approximated by the product of the cosine squared of bond torsion angles for the SQ-bridge and bridge-NN linkages.10,44 Based solely on the differences in SQ-bridge-NN torsion angles, this is expected to reduce the magnitude of JSQ-NN for LZn(SQ-6-Me-mPh-NN) by >99% relative to LZn(SQ-mPh-NN). The larger experimental JSQ-NN value observed for LZn(SQ-6-Me-mPh-NN), compared to that predicted solely by the bond torsion angles, derives from a combination of weak σ- and configuration interaction contributions to the exchange.10,41 Critically, the bridge methyl group attenuates antiferromagnetic JSQ-NN exchange in LZn(SQ-6-Me-mPh-NN) and this translates to a similar attenuation of JSQ-NN in the LL′CT excited state of 6-Me-mPh-Pt that results in ΔED1Q(6-Me-mPh-Pt) < ΔED1Q(mPh-Pt) (Fig. 2A).
Excited state exchange determines ground state electron spin polarization
Low-temperature EPR and time-resolved EPR (TREPR) spectra of mPh-Pt and 6-Me-mPh-Pt are presented in Fig. 4A and B, respectively, and these data highlight the dramatic difference in their spin polarized EPR signals at virtual parity of their molecular (but not conformational) structure, ground-state EPR spectra, and LL′CT energy. Note that the direct-detection method used to measure the TREPR spectra is insensitive to static EPR signals and the difference between EPR absorbance before, and after, the laser flash is taken. Thus, a signal is only observed when time-dependent spin polarization is generated. We note that no ESP is generated in (DMSO)2Pt(CAT-m-Ph-NN), which lacks an LL′CT excited state, indicating that the LL′CT excited state is necessary to observe ground state ESP in these systems.16
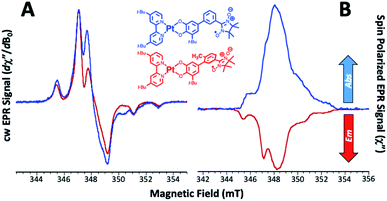 |
| Fig. 4 X-band cw-EPR (A); 20 K; 2-MTHF, and TREPR (B); 20 K; 2-MTHF spectra for mPh-Pt (blue lines) and 6-Me-mPh-Pt (red lines). (A) Field modulation detected cw-EPR spectra of the ground states without light excitation. (B) Light-induced, spin-polarized TREPR spectra, extracted from time/field datasets 4 μs after the laser flash. Light excitation at 532 nm, concentrations ∼0.5 mM. Abs = absorption, Em = emission. | |
Remarkably, and contrary to the predictions of the RQM, we observe emissive ESP in the recovered ground state of 6-Me-mPh-Pt and absorptive ESP in the recovered ground state of mPh-Pt. Thus, the non-Boltzmann population distribution within the 2S0ms = ±1/2 sublevels generated by the laser-induced excitation/relaxation photocycle (Fig. 2A and 5) differs markedly for these two complexes. The differences in the nature of the ESP for mPh-Pt and 6-Me-mPh-Pt correlate with the bridge-dependent energy gaps between 2T1 and the other states (4T1, 2NN, 4NN) in this energy region, which are affected by CAT/SQ-bridge bond torsions. Since the degree of charge separation in the excited state manifolds of mPh-Pt and 6-Me-mPh-Pt is near unity,13 the JSQ-NN value for LZn(SQ-6-Me-mPh-NN) can be used as a proxy for JSQ-NN in the excited state of 6-Me-mPh-Pt. The JSQ-NN exchange interaction that we obtain for LZn(SQ-6-Me-mPh-NN) is related to the 2T1–4T1 energy gap (ΔED1Q) in the three-spin excited states of mPh-Pt and 6-Me-mPh-Pt according to ΔED1Q = 1.5 JSQ-NN when Jbpy˙-SQ ≫ JSQ-NN.17 Thus, the 2T1–4T1 energy gaps (ΔED1Q) are 48 cm−1 for mPh-Pt and 6 cm−1 for 6-Me-mPh-Pt, respectively.
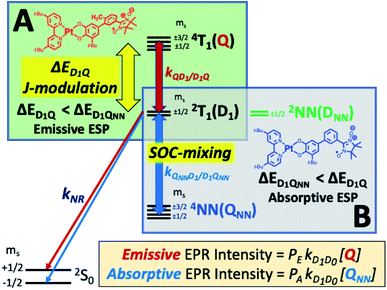 |
| Fig. 5 (A) The reversed quartet mechanism (RQM). Mixing of chromophoric 2T1 and 4T1 states, mediated by conformational JSQ-NN-modulation, gives rise to emissive ESP for 6-Me-mPh-Pt. (B) Spin–orbit induced mixing of chromophoric 2T1 and the NN-based quartet state, 4NN, gives rise to absorptive ESP for mPh-Pt. The 2NN state can be observed spectroscopically (see ESI†). | |
These ΔED1Q values indicate that <6% of the mPh-Pt4T1 state would be populated at thermal equilibrium at 20 K (the temperature at which the EPR spectra were recorded), but this increases dramatically to ∼56% 4T1 population for 6-Me-mPh-Pt at the same temperature. These populations are significant because the RQM (Fig. 1A and 4) requires thermal population of the 4T1(Q) state and spin–orbit coupling (SOC) induced zero-field mixing between the 2T1–4T1 states to observe ground- and excited state ESP in mPh-Pt and 6-Me-mPh-Pt. SOC induced interconversion rates between Zeeman split 2T1 and 4T1 states are given by the rate constants kDQ and kQD (Fig. 5), with kDQ and kQD being related by the equilibrium Boltzmann populations between the two levels (i.e.
).35,36 Given that JSQ-NN is antiferromagnetic in 6-Me-mPh-Pt, the RQM correctly predicts emissive ground state ESP due to E(4T1) > E(2T1), 2T1–4T1 SOC state mixing, and kQD > kDQ.35,36 Since mPh-Pt displays absorptive ground state ESP and a significantly larger 2T1(D1)–4T1(Q) energy gap, a different ESP mechanism must be operational for this complex.
Recently, we described how localized NN radical 2NN(DNN) and 4NN(QNN) states (Fig. 4B) may be nearly isoenergetic with the 2T1 state of mPh-Pt.16 We propose that the dynamic population of the 4NN state effectively competes with the higher energy 4T1 state to determine the sign of the ground state ESP. This competition is readily apparent in mPh-Pt and results from the larger value of JSQ-NN for this complex, which leads to a larger ΔED1Q energy gap, a more stabilized 2T1 state, and ΔED1Q > ΔED1-QNN between chromophoric 2T1 and QNN states (Fig. 5). The inclusion of additional states in the context of the RQM is key, as it correctly predicts the experimentally observed absorptive ESP via2T1 ↔ QNN mixing for mPh-Pt. Thus, the magnitude of the antiferromagnetic excited state exchange interaction, JSQ-NN, controls how the 2T1 state mixes with neighboring excited states to switch the sign of the ground state ESP. This observation highlights the effectiveness of TREPR spectroscopy for revealing details of fast non-radiative processes that are difficult to detect using other spectroscopic methods. In the present case, the sign of the ground state ESP reveals how the small energy gap between charge transfer and localized excited states, coupled with the relative magnitudes of interstate mixing, critically alters the spin selectivity of the electronic relaxation.
Conclusions
In summary, we observe dramatic differences in ground state ESP for mPh-Pt and 6-Me-mPh-Pt that are attributed to the energies of their 2T1 states relative to chromophoric 4T1(Q) states and a localized radical-based QNN state (Fig. 6). For 6-Me-mPh-Pt, an LL′CT excited state SQ-bridge bond torsion effectively attenuates the magnitude of the excited state JSQ-NN antiferromagnetic exchange interaction, which leads to ΔED1Q < ΔED1QNN and emissive ESP as described by the RQM (Fig. 1A) and observed experimentally (Fig. 4B). Conversely, the comparatively planar CAT-mPh-NN π-system of mPh-Pt results in ΔED1QNN < ΔED1Q, and absorptive ESP is both predicted and observed (Fig. 1B and 4B, respectively). Thus, small conformational changes along low-frequency torsional modes of donor–acceptor molecules that have been elaborated with pendant persistent radicals can lead to conformational J-modulation, and this affects the sign and magnitude of the ground state ESP. This indicates that the sign of the excited state chromophore-radical exchange predicted by the RQM is not universal, and our work points to a previously unobserved change in ground state ESP as a function of the magnitude of the excited state exchange interaction, JSQ-NN. The present results highlight the extreme importance of understanding how excited state electronic structure affects excited state properties and dynamics, and demonstrates additional mechanisms to control ESP relevant to QIS. Ongoing research efforts focus on further exploration of synthetic design principles directed toward determining the electronic and geometric structure factors that control optically-generated ESP in these and related radical-elaborated donor–acceptor chromophores, and how these can be exploited for QIS applications.
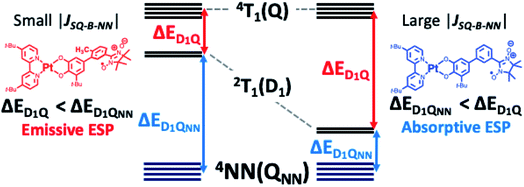 |
| Fig. 6 Origins of emissive and absorptive ESP for NN-elaborated (bpy)Pt(CAT) complexes. Left: RQM with emissive ESP is observed. Right: RQM with absorptive ESP is observed. | |
Experimental
General considerations
Reagents and solvents were used as received and purchased from commercial vendors. See ESI† for compound syntheses and characterization.
Magnetic susceptibility measurements
Magnetic susceptibility measurements were collected on a Quantum Design MPMS-XL7 SQUID magnetometer. Variable temperature magnetometry experiments were performed with a constant field of 0.7 T on a microcrystalline sample (∼15 mg) which was loaded into a gelcap/straw sample holder and mounted to the sample rod with Kapton tape. Collected raw data was initially corrected with a straight line for diamagnetic response of sample and container as a first approximation, where the slope of the line represents the residual diamagnetic correction.
EPR and TREPR spectroscopies
Samples for steady state and transient EPR measurements were prepared by dissolving solid (bpy)Pt(CAT-mPh-NN), (mPh-Pt) or (bpy)Pt(CAT-6-Me-mPh-NN), (6-Me-mPh-Pt) in 2-methylTHF to a concentration of ∼0.5 mM. The samples were placed in 4 mm O.D. quartz EPR tubes and degassed by repeated freeze-pump-thaw cycles. The frozen, degassed samples were then transferred without thawing from liquid nitrogen to the spectrometer cryostat, which was at 20 K. The steady state spectra were collected using field modulation detection with a modulation amplitude of 0.1 mT and a microwave power of 6.3 mW. TREPR time/field dataset were collected with direct detection at the microwave power as for the steady state experiments. The samples were irradiated at 532 nm using 10 ns laser flashes from a frequency doubled NdYAG laser. The modified Bruker EPR 200D-SRC X-band spectrometer used for the EPR experiments has been described in detail elsewhere.45
Data availability
The datasets supporting this article have been uploaded as part of the ESI.†
Author contributions
M. L. K. supervised the research, vetted the data, and wrote the manuscript. D. A. S. supervised the research, vetted the data, and wrote the manuscript. P. H. synthesized and characterized the compounds. D. E. S. synthesized and characterized the compounds. J .C. performed electronic structure computations. A. v. d. E. supervised the research, vetted the data, and wrote the manuscript.
Conflicts of interest
No competing financial interests have been declared.
Acknowledgements
M. L. K. and D. A. S. acknowledge financial support from DOE (DE-SC0020199). A. v. d. E. acknowledges support from NSERC (Discovery grant 2015-04021).
References
- M. R. Wasielewski, M. D. E. Forbes, N. L. Frank, K. Kowalski, G. D. Scholes, J. Yuen-Zhou, M. A. Baldo, D. E. Freedman, R. H. Goldsmith, T. Goodson, M. L. Kirk, J. K. McCusker, J. P. Ogilvie, D. A. Shultz, S. Stoll and K. B. Whaley, Exploiting chemistry and molecular systems for quantum information science, Nat. Rev. Chem., 2020, 4(9), 490–504 CrossRef CAS.
- J. Lehmann, A. Gaita-Arino, E. Coronado and D. Loss, Quantum computing with molecular spin systems, J. Math. Chem., 2009, 19(12), 1672–1677 RSC.
- A. Palii, S. Aldoshin and B. Tsukerblat, Mixed-Valence Triferrocenium Complex with Electric Field Controllable Superexchange as a Molecular Implementation of Triple Quantum Dot, J. Phys. Chem. C, 2017, 121(48), 27218–27224 CrossRef CAS.
- P. C. E. Stamp and A. Gaita-Arino, Spin-based quantum computers made by chemistry: hows and whys, J. Math. Chem., 2009, 19(12), 1718–1730 RSC.
- D. P. DiVincenzo, The physical implementation of quantum computation, Fortschr. Phys., 2000, 48(9–11), 771–783 CrossRef.
- K. S. Akhilesh, B. G. Divyamani, Sudha, A. R. U. Devi and K. S. Mallesh, Spin squeezing in symmetric multiqubit states with two non-orthogonal Majorana spinors, Quantum Inf. Process., 2019, 18(5), 144 CrossRef.
- V. A. Soltamov, C. Kasper, A. V. Poshakinskiy, A. N. Anisimov, E. N. Mokhov, A. Sperlich, S. A. Tarasenko, P. G. Baranov, G. V. Astakhov and V. Dyakonov, Excitation and coherent control of spin qudit modes in silicon carbide at room temperature, Nat. Commun., 2019, 10, 1678 CrossRef CAS PubMed.
- A. de, A. Lang, D. Zhou and R. Joynt, Suppression of decoherence and disentanglement by the exchange interaction, Phys. Rev., 2011, 83(4), 042331 CrossRef.
- M. Orrit, Molecular Entanglements, Science, 2002, 298(5592), 369 CrossRef CAS PubMed.
- D. A. Shultz, M. L. Kirk, J. Y. Zhang, D. E. Stasiw, G. B. Wang, J. Yang, D. Habel-Rodriguez, B. W. Stein and R. D. Sommer, Spectroscopic Signatures of Resonance Inhibition Reveal Differences in Donor-Bridge and Bridge-Acceptor Couplings, J. Am. Chem. Soc., 2020, 142(10), 4916–4924 CrossRef CAS PubMed.
- C. R. Tichnell, D. R. Daley, B. W. Stein, D. A. Shultz, M. L. Kirk and E. O. Danilov, Wave Function Control of Charge-Separated Excited-State Lifetimes, J. Am. Chem. Soc., 2019, 141(9), 3986–3992 CrossRef CAS PubMed.
- B. W. Stein, D. A. Dickie, S. Nedungadi, D. J. R. Brook, D. A. Shultz and M. L. Kirk, Long-range spin dependent delocalization promoted by the pseudo Jahn-Teller effect, J. Chem. Phys., 2019, 151(20), 201103 CrossRef PubMed.
- J. Yang, D. K. Kersi, C. P. Richers, L. J. Giles, R. Dangi, B. W. Stein, C. Feng, C. R. Tichnell, D. A. Shultz and M. L. Kirk, Ground State Nuclear Magnetic Resonance Chemical Shifts Predict Charge-Separated Excited State Lifetimes, Inorg. Chem., 2018, 57(21), 13470–13476 CrossRef CAS PubMed.
- D. E. Stasiw, J. Y. Zhang, G. B. Wang, R. Dangi, B. W. Stein, D. A. Shultz, M. L. Kirk, L. Wojtas and R. D. Sommer, Determining the Conformational Landscape of σ and π Coupling Using para-Phenylene and "Aviram-Ratner" Bridges, J. Am. Chem. Soc., 2015, 137(29), 9222–9225 CrossRef CAS PubMed.
- J. Yang, D. K. Kersi, L. J. Giles, B. W. Stein, C. J. Feng, C. R. Tichnell, D. A. Shultz and M. L. Kirk, Ligand Control of Donor-Acceptor Excited-State Lifetimes, Inorg. Chem., 2014, 53(10), 4791–4793 CrossRef CAS PubMed.
- M. L. Kirk, D. A. Shultz, J. Chen, P. Hewitt, D. Daley, S. Paudel and A. van der Est, Metal Ion Control of Photoinduced Electron Spin Polarization in Electronic Ground States, J. Am. Chem. Soc., 2021, 143(28), 10519–10523 CrossRef CAS PubMed.
- B. W. Stein, C. R. Tichnell, J. Chen, D. A. Shultz and M. L. Kirk, Excited State Magnetic Exchange Interactions Enable Large Spin Polarization Effects, J. Am. Chem. Soc., 2018, 140(6), 2221–2228 CrossRef CAS PubMed.
- M. Milivojevic, Maximal thermal entanglement using three-spin interactions, Quantum Inf. Process., 2019, 18(2), 1–14 Search PubMed.
- Z. B. Zheng Yi-Dan, Tripartite entanglement of {Cu3} single molecular magnet with magnetic field in thermal equilibrium, Acta Phys. Sin., 2016, 65(12), 120301 Search PubMed.
- P. Amit Kumar and B. Indrani, Entanglement in a molecular three-qubit system, J. Phys.: Condens. Matter, 2010, 22(1), 016004 CrossRef PubMed.
- Z. J. Wang, Y. T. Gao, M. Hussain, S. Kundu, V. Rane, M. Hayvali, E. A. Yildiz, J. Z. Zhao, H. G. Yaglioglu, R. Das, L. Luo and J. F. Li, Efficient Radical-Enhanced Intersystem Crossing in an NDI-TEMPO Dyad: Photophysics, Electron Spin Polarization, and Application in Photodynamic Therapy, Chem.– Eur. J., 2018, 24(70), 18663–18675 CrossRef CAS PubMed.
- A. Ito, A. Shimizu, N. Kishida, Y. Kawanaka, D. Kosumi, H. Hashimoto and Y. Teki, Excited-State Dynamics of Pentacene Derivatives with Stable Radical Substituents, Angew. Chem., Int. Ed., 2014, 53(26), 6715–6719 CrossRef CAS PubMed.
- Y. Teki and T. Matsumoto, Theoretical study of dynamic electron-spin-polarization via the doublet-quartet quantum-mixed state and time-resolved ESR spectra of the quartet high-spin state, Phys. Chem. Chem. Phys., 2011, 13(13), 5728–5746 RSC.
- M. T. Colvin, E. M. Giacobbe, B. Cohen, T. Miura, A. M. Scott and M. R. Wasielewski, Competitive Electron Transfer and Enhanced Intersystem Crossing in Photoexcited Covalent TEMPO-Perylene-3,4:9,10-bis(dicarboximide) Dyads: Unusual Spin Polarization Resulting from the Radical-Triplet Interaction, J. Phys. Chem. A, 2010, 114(4), 1741–1748 CrossRef CAS PubMed.
- Y. Teki and T. Matsumoto, Spin dynamics on photoexcited state of functionality π-radical via quantum-mixed state: Theoretical study of the spin polarized state generation using the mechanism via quantum-mixed state, Synth. Met., 2013, 173, 35–39 CrossRef CAS.
- T. Matsumoto and Y. Teki, Theoretical study of dynamic electron-spin-polarization via the doublet-quartet quantum-mixed state (II). Population transfer and magnetic field dependence of the spin polarization, Phys. Chem. Chem. Phys., 2012, 14(29), 10178–10186 RSC.
- M. Zarea, M. A. Ratner and M. R. Wasielewski, Spin polarization transfer by the radical pair mechanism, J. Chem. Phys., 2015, 143(5), 054101 CrossRef PubMed.
- E. T. Chernick, R. Casillas, J. Zirzlmeier, D. M. Gardner, M. Gruber, H. Kropp, K. Meyer, M. R. Wasielewski, D. M. Guldi and R. R. Tykwinski, Pentacene Appended to a TEMPO Stable Free Radical: The Effect of Magnetic Exchange Coupling on Photoexcited Pentacene, J. Am. Chem. Soc., 2015, 137(2), 857–863 CrossRef CAS PubMed.
- M. T. Colvin, R. Carmieli, T. Miura, S. Richert, D. M. Gardner, A. L. Smeigh, S. M. Dyar, S. M. Conron, M. A. Ratner and M. R. Wasielewski, Electron Spin Polarization Transfer from Photogenerated Spin-Correlated Radical Pairs to a Stable Radical Observer Spin, J. Phys. Chem. A, 2013, 117(25), 5314–5325 CrossRef CAS PubMed.
- Q. X. Mi, E. T. Chernick, D. W. McCamant, E. A. Weiss, M. A. Ratner and M. R. Wasielewski, Spin dynamics of photogenerated triradicals in fixed distance electron donor-chromophore-acceptor-TEMPO
molecules, J. Phys. Chem. A, 2006, 110(23), 7323–7333 CrossRef CAS PubMed.
- E. T. Chernick, Q. X. Mi, R. F. Kelley, E. A. Weiss, B. A. Jones, T. J. Marks, M. A. Ratner and M. R. Wasielewski, Electron donor-bridge-acceptor molecules with bridging nitronyl nitroxide radicals: Influence of a third spin on charge- and spin-transfer dynamics, J. Am. Chem. Soc., 2006, 128(13), 4356–4364 CrossRef CAS PubMed.
- Y. E. Kandrashkin and A. van der Est, The triplet mechanism of electron spin polarization in moderately coupled triplet-doublet rigid complexes as a source of the enhanced +1/2 ↔ −1/2 transitions, J. Chem. Phys., 2019, 151, 184301 CrossRef PubMed.
- Y. E. Kandrashkin and A. van der Est, Orientation Information from the Dipolar Interaction Between a Complex in the Excited Quartet State and a Doublet Spin Label, Appl. Magn. Reson., 2014, 45(3), 217–237 CrossRef.
- Y. Kandrashkin and A. van der Est, Electron spin polarization of the excited quartet state of strongly coupled triplet–doublet spin systems, J. Chem. Phys., 2004, 120, 4790–4799 CrossRef CAS PubMed.
- V. Rozenshtein, A. Berg, E. Stavitski, H. Levanon, L. Franco and C. Corvaja, Electron spin polarization of functionalized fullerenes. Reversed quartet mechanism, J. Phys. Chem. A, 2005, 109(49), 11144–11154 CrossRef CAS PubMed.
- A. K. Tripathi, V. Rane, S. Kundu and R. Das, A phenomenological scheme for reversed quartet mechanism of electron spin polarization in covalently linked systems of chromophore and free radical: Determination of magnitude of polarization and application to pyrene–TEMPO linked molecules, J. Chem. Phys., 2019, 151(15), 154305 CrossRef PubMed.
- K. Ishii, Y. Hirose, H. Fujitsuka, O. Ito and N. Kobayashi, Time-resolved EPR, fluorescence, and transient absorption studies on phthalocyaninatosilicon covalently linked to one or two TEMPO radicals, J. Am. Chem. Soc., 2001, 123(4), 702–708 CrossRef CAS PubMed.
- K. Kanemoto, A. Fukunaga, M. Yasui, D. Kosumi, H. Hashimoto, H. Tamekuni, Y. Kawahara, Y. Takemoto, J. Takeuchi, Y. Miura and Y. Teki, Ultrafast photoexcitation dynamics of pi-conjugated bodipy-anthracene-radical triad system, RSC Adv., 2012, 2(12), 5150–5153 RSC.
- M. L. Kirk, D. A. Shultz, E. C. Depperman and C. L. Brannen, Donor-acceptor biradicals as ground state analogues of photoinduced charge separated states, J. Am. Chem. Soc., 2007, 129(7), 1937–1943 CrossRef CAS PubMed.
-
G. Bussiere, R. Beaulac, H. Belisle, C. Lescop, D. Luneau, P. Rey and C. Reber, Excited states and optical spectroscopy of nitronyl nitroxides and their lanthanide and transition metal complexes, in Transition Metal and Rare Earth Compounds Iii: Excited States, Transitions, Interactions, ed. H. Yersin, 2004, vol. 241, pp. 97-+ Search PubMed.
- M. L. Kirk, D. A. Shultz, D. E. Stasiw, D. Habel-Rodriguez, B. Stein and P. D. Boyle, Electronic and Exchange Coupling in a Cross-Conjugated D-B-A Biradical: Mechanistic Implications for Quantum Interference Effects, J. Am. Chem. Soc., 2013, 135(39), 14713–14725 CrossRef CAS PubMed.
-
E. A. Boudreaux and L. N. Mulay, Theory and applications of molecular paramagnetism, Wiley Interscience, New York, 1976, p. 510 Search PubMed.
-
O. Kahn, Molecular Magnetism, VCH, New York, 1993, p. 380 Search PubMed.
- D. E. Stasiw, J. Y. Zhang, G. B. Wang, R. Dangi, B. W. Stein, D. A. Shultz, M. L. Kirk, L. Wojtas and R. D. Sommer, Determining the Conformational Landscape of σ and π Coupling Using para-Phenylene and “Aviram-Ratner” Bridges, J. Am. Chem. Soc., 2015, 137(29), 9222–9225 CrossRef CAS PubMed.
- W. Xu, P. Chitnis, A. Valieva, A. van der Est, Y. N. Pushkar, M. Krzystyniak, C. Teutloff, S. G. Zech, R. Bittl, D. Stehlik, B. Zybailov, G. Z. Shen and J. H. Golbeck, Electron transfer in cyanobacterial photosystem I - I. Physiological and spectroscopic characterization of site-directed mutants in a putative electron transfer pathway from A(0) through A(1) to F-x, J. Biol. Chem., 2003, 278(30), 27864–27875 CrossRef CAS PubMed.
Footnotes |
† Electronic supplementary information (ESI) available: Synthesis and characterization of 6-Me-mPh-Pt and related complexes, X-ray crystallographic analysis for LZn(SQ-6-Me-mPh-NN). CCDC 2087657. For ESI and crystallographic data in CIF or other electronic format see DOI: 10.1039/d1sc02965g |
‡ These authors contributed equally. |
§ Present address: Cree, RTP, Durham, NC. |
|
This journal is © The Royal Society of Chemistry 2021 |