DOI:
10.1039/D1SC02937A
(Edge Article)
Chem. Sci., 2021,
12, 11447-11454
Pyrimidine-directed metal-free C–H borylation of 2-pyrimidylanilines: a useful process for tetra-coordinated triarylborane synthesis†
Received
31st May 2021
, Accepted 22nd July 2021
First published on 23rd July 2021
Abstract
Convenient, easily handled, laboratory friendly, robust approaches to afford synthetically important organoboron compounds are currently of great interest to researchers. Among the various available strategies, a metal-free approach would be overwhelmingly accepted, since the target boron compounds can be prepared in a metal-free state. We herein present a detailed study of the metal-free directed ortho-C–H borylation of 2-pyrimidylaniline derivatives. The approach allowed us to synthesize various boronates, which are synthetically important compounds and various four-coordinated triarylborane derivatives, which could be useful in materials science as well as Lewis-acid catalysts. This metal-free directed C–H borylation reaction proceeds smoothly without any interference by external impurities, such as inorganic salts, reactive functionalities, heterocycles and even transition metal precursors, which further enhance its importance.
Introduction
Directed C–H bond activation has emerged as one of the most powerful and reliable tools in synthetic organic chemistry.1 A variety of directing groups are being designed for achieving a regioselective C–H bond functionalization reaction. Among them, the metal-catalyzed C–H functionalization of 2-pyrimidylanilines was investigated on a number of occasions.2,3 In this regard, ortho-C–H alkylation,2 alkyne annulation,3a alkynylation,3b,c chalcogenation,3d amidation3f–h and the alkenylation3i of aniline derivatives have been achieved using various metal catalysts (Fig. 1A). Nevertheless, given the importance of organoboron compounds in organic synthesis,4 selective C–H borylation has not yet been achieved in the case of 2-pyrimidylanilines, which are key structural motifs that are found in numerous bioactive compounds that are used in crop protection and medicinal chemistry.5 Considering the recent progress in metal-free directed C–H borylation, it would be predicted that this reaction could be used in conjunction with a broad spectrum of substrates to prepare a wide variety of synthetically important organoboron compounds without the use of any metal precursors. In this regard, notable contributions include the directed C–H borylation of various nitrogen-containing heterocycles,6 such as benzimidazoles,6a pyridines,6c–e,h,k benzothiadiazoles,6f,i,j quinolines,6g benzoselenadiazoles,6l benzotriazoles,6l and indoles,6m a thio-directed intramolecular C–H borylation of 2-mercaptobiphenyl,6b and pre-installing a directing group7 assisted C–H borylation of phenol,7a,b aniline,7c–e indole,7c,d and pyrrole derivatives.7f We recently reported the transient imine-directed C–H borylation of benzaldehydes.8 Having a continuous interest in this metal-free C–H borylation protocol, we were prompted to explore the selective C–H borylation of 2-pyrimidylanilines.
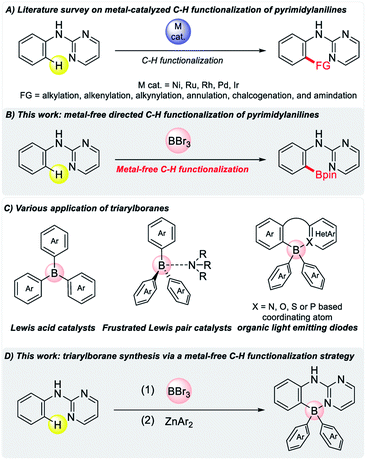 |
| Fig. 1 (A) Literature survey of the directed ortho-C–H functionalization of 2-pyrimidylanilines. (B) Our work on the metal-free C–H borylation of 2-pyrimidylanilines. (C) The importance of triarylboranes in various fields and (D) our approach to accessing tetra-coordinated triarylboranes in a straightforward manner. | |
Triarylborane-based compounds have wide application as Lewis acid catalysts or frustrated Lewis pair catalysts in various catalyses such as hydrosilylation, hydroboration, Diels–Alder reactions and for small molecule activation.9 In addition, there are widespread applications for tetra-coordinated triarylboranes as organic materials, such as anion sensors, organic light emitting devices, imaging and electron transport materials (Fig. 1C).10 Four-coordinated organoboron compounds can function as electron-transport materials via the boron-stabilized p* orbital of the donating ligands. Considering these wide applications, various strategies have emerged for their synthesis. One of the more recent strategies is cascade B–Cl/C–B cross-metathesis and C–H bond borylation.11 While this strategy is interesting, it has some drawbacks, including the need for harsh conditions, the production of various waste by-products, and relatively poor yields of triarylboranes. Therefore, the most attractive strategy would involve the use of boron trihalide to construct tetra-coordinated fluorophores via activating a C–H bond using a directing atom of a heterocycle. Given our focus on metal-free C–H borylation reactions,8 we developed a keen interest in accessing tetracoordinated triarylboranes containing a 2-pyrimidylaniline group. In 2019, Shi/Houk and Ingleson independently reported the directed C–H borylation of anilides using an oxygen atom as a directing moiety with BBr3 for preparing boronic esters of anilines.7c,d We hypothesized that, compared to an oxygen directing atom, a nitrogen moiety would provide access to more stable tetra-coordinated triarylboranes. Hence, nitrogen-based heterocycles were mainly used for organic materials due to their high stability. We herein report on the metal-free C–H borylation of 2-pyrimidylaniline scaffolds to afford synthetically important boronic esters (Fig. 1B) and the synthesis of tetra-coordinated triarylboranes (Fig. 1D).
Results and discussion
We started the project by investigating the reaction conditions for the directed metal-free C–H borylation of 2-pyrimidylanilines. The reaction of N-(pyrimidine-2-yl)aniline (1a) and 1 equiv. of BBr3 in dichloroethane (DCE) gave 21% NMR yield of the expected borylated product 1b (entry 1, Table 1). Remarkably, the reaction proceeded even in the case of 1a, which contains a free NH moiety. An increase in the amount of BBr3 to 2 equiv. improved the product yield to 50% (entry 2). After a thorough screening of various bases, it was found that 2,3,5,6-tetramethylpyrazine (B5) was the optimal base, giving 1b in 84% yield (entry 10 vs. entries 3–9). Adjusting the amounts of base and BBr3 used provided the optimal conditions in which the use of 3 equiv. of BBr3 along with 1.2 equiv. of B5 gave 1b in 77% isolated yield (entry 12). The reactivity was retained when the solvent was switched from DCE to CHCl3 (entry 13).
Table 1 Optimization of the metal-free borylation of 2-pyrimidylaniline 1aa

|
Entry |
Base (YY equiv.) |
BBr3 (XX equiv.) |
Solvent |
Yield of 1b (%) |
Reaction conditions: 2-pyrimidylaniline (1a, 0.1 mmol, 1 equiv.), BBr3 and base in DCE (0.5 mL) at r.t. for 4 h, then pinacol (0.2 mmol) and NEt3 (1.0 mmol) in DCE (0.5 mL) for 2 h at r.t. Yields of the product were determined by 1H NMR of the crude mixture using 1,1,2,2-tetrachloroethane as an internal standard. Isolated yields are given in parentheses.
1 equiv. of pinacol was used in the final step.
3 equiv. of pinacol was used in the final step.
|
1b |
— |
1 equiv. |
DCE |
21 |
2 |
— |
2 equiv. |
DCE |
50 |
3 |
B1 (2 equiv.) |
2 equiv. |
DCE |
71 |
4 |
B2 (2 equiv.) |
2 equiv. |
DCE |
66 |
5 |
Pyridine (2 equiv.) |
2 equiv. |
DCE |
5 |
6 |
NEtiPr2 (2 equiv.) |
2 equiv. |
DCE |
65 |
7 |
B3 (2 equiv.) |
2 equiv. |
DCE |
3 |
8 |
B4 (2 equiv.) |
2 equiv. |
DCE |
17 |
9 |
NEt3 (2 equiv.) |
2 equiv. |
DCE |
61 |
10 |
B5 (2 equiv.) |
2 equiv. |
DCE |
84 |
11c |
B5 (3 equiv.) |
3 equiv. |
DCE |
93 |
12c |
B5 (1.2 equiv.) |
3 equiv. |
DCE |
92 (77) |
13c |
B5 (1.2 equiv.) |
3 equiv. |
CHCl3 |
90 (76) |
|
With the optimized reaction conditions in hand, we explored the versatility of this ortho-directed metal-free borylation reaction using various substituted 2-pyrimidylaniline derivatives (Table 2). This protocol provided a broad scope of substrates and a high tolerance for various functional groups. Notably, a variety of substituted aniline derivatives containing an electron-donating group, such as methyl (2b-4b, 8b, and 9b), ethyl (5b), benzyl (6b), phenyl (7b), methylthio (10b), phenylthio (11b), and phenoxy (12b), reacted smoothly to afford the corresponding boronic esters in yields in the range of 41–80%. 1-Naphthylamine (13a) and 1-aminopyrene (14a) selectively produced the desired products (13b and 14b) in high yields without the formation of any side products. In the case of the meta-Me-substituted aniline, the reaction occurred exclusively at the sterically less hindered site (3b). A variety of electron-withdrawing functional groups, such as F (15b–17b), Cl (18b–21b), Br (22b–25b), I (26b), CF3 (27b), and even NO2 (28b) were also tolerated in this metal-free C–H borylation reaction. The reaction proceeded readily in the case of substituents on the pyrimidine ring, as in Me (29a and 30a), Cl (31a), and Br (32a) groups. Notably, this wide substrate applicability increases the significance of this easily handled and convenient directed C–H borylation protocol to afford borylated synthetic intermediates.
Table 2 Substrate scope for the metal-free borylation of 2-pyrimidylanilines
Reaction conditions: 2-pyrimidylaniline derivative (0.3 mmol, 1 equiv.), BBr3 (0.9 mmol, 3 equiv.) and tetramethylpyrazine (0.36 mmol, 1.2 equiv.) in DCE (1.0 mL) at r.t. for 4 h, then pinacol (0.9 mmol, 3 equiv.) and NEt3 (3.0 mmol, 10 equiv.) in DCE (1.0 mL) at r.t. for 2 h. Isolated yields of the product are given.
Reaction was performed on a 5 mmol scale.
|
|
Four-coordinated organoboron compounds have been extensively investigated in the past decade as emissive materials for use in organic light-emitting diodes, due to their high thermal and chemical stability.10 We attempted to prepare a series of tetra-coordinated triarylborane derivatives using this metal-free ortho-C–H borylation of 2-pyrimidylaniline derivatives. A pyrimidine-directed borylation with BBr3 gave a dibromoborane intermediate and the subsequent reaction with diarylzinc provided the desired four-coordinated triarylboranes. Various electron-donating or neutral group-substituted aniline derivatives (33–37), electron-withdrawing group substituted aniline derivatives (38–42), and even a substrate with a substitution on the pyrimidine ring (43) reacted efficiently to afford the desired products in good to high yields (Table 3). These entities would be of value in terms of preparing many other pyrimidinyl-coordinated triarylborane compounds. A di-pentafluorophenyl substituted triarylborane (44) was also synthesized when bis(pentafluorophenyl)zinc was used. This protocol could lead to the production of various substituted boron-based Lewis acid catalysts. When trimethylaluminum was reacted with the dibromoborane intermediate, a dimethyl substituted aryl borane compound 45 was obtained. This convenient synthetic protocol for obtaining various substituted four-coordinated boranes clearly provides opportunities to produce a library of compounds containing the 2-pyrimidylaniline moiety, which would be useful as emissive materials.
Table 3 Synthesis of tetra-coordinated triarylborane via a metal-free C–H borylationa
Reaction conditions: 2-pyrimidylaniline derivative (0.15 mmol, 1 equiv.), BBr3 (0.45 mmol, 3 equiv.), and tetramethylpyrazine (0.18 mmol, 1.2 equiv.) in CHCl3 (1.0 mL) at r.t. for 4 h, evaporated under reduced pressure, after which, toluene (1.5 mL) and (0.45 mmol, 3 equiv.) were added followed by heating at 70 °C for 12 h.
2-Pyrimidylaniline (0.15 mmol, 1 equiv.), BBr3 (0.45 mmol, 3 equiv.) and 2,6-lutidine (0.45 mmol, 3 equiv.) in DCE (1.0 mL) at r.t. for 4 h, then AlMe3 (0.9 mmol, 6 equiv.) for 12 h at r.t. Isolated yields of the product are given.
|
|
The wide substrate applicability of this metal-free borylation prompted us to investigate the sustainability of this protocol in the presence of impurities. Although detail studies of robustness and sensitivity have been previously reported for metal-catalyzed organic synthetic procedures,12 metal-free protocols have not gained much attention.8 To examine this issue, we performed a series of borylation reactions using 1a in the presence of 1 equiv. of various external impurities, such as inorganic salts, reactive functionalities, heterocycles, and transition metals (Fig. 2). To our delight, in most cases, the reaction proceeded smoothly to afford the desired product 1b with negligible interference by external impurities. These observations further strongly support the sustainability of this protocol from a synthetic point of view. We anticipated that the robustness of the procedure would make this protocol useful in terms of large-scale organic synthesis.
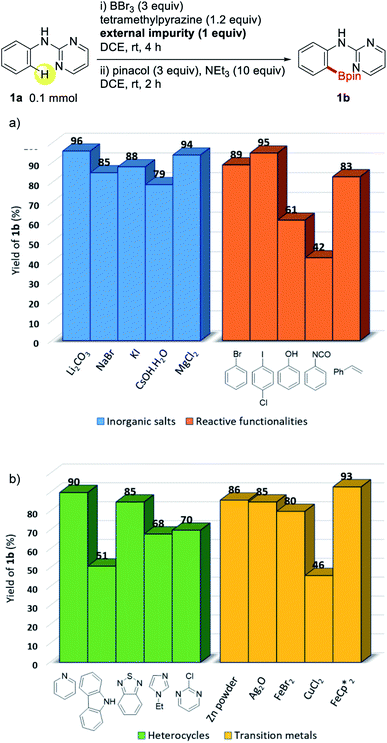 |
| Fig. 2 Metal-free directed borylation of 2-pyrimidylaniline in the presence of external impurities such as (a) inorganic salts and reactive functionalities, (b) heterocycles and transition metals. | |
Since various impurities were tolerated in this protocol, we performed a series of sequential reactions, in which the first step is the synthesis of the starting 2-pyrimidylanilines followed by directed C–H borylation without isolating the starting materials. The sequential reactions proceeded without any difficulties to afford the desired products 1b, 11b, and 30b, despite the presence of various impurities such as aniline derivatives, 2-chloropyrimidine, traces of solvent and acetic acid (Scheme 1). This experiment further confirms the efficacy of this protocol for use in a multi-step organic synthetic process.
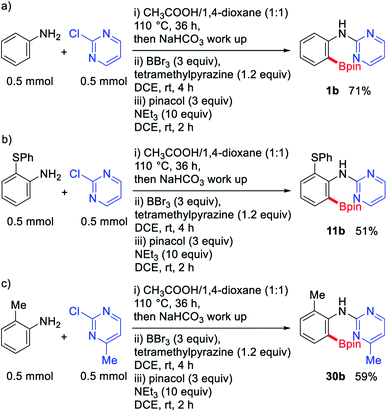 |
| Scheme 1 (a)–(c) Sequential one-pot reaction of starting material synthesis followed by metal-free directed borylation. | |
To evaluate the synthetic utilities of ortho-borylated 2-pyrimidylaniline derivatives, some important synthetic transformations were performed and the results for reactions including arylation and alkenylation are presented in Scheme 2, which indicate the smooth formation of the desired products (46-48).
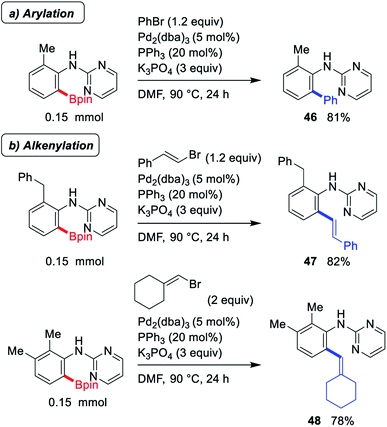 |
| Scheme 2 Representative synthetic application of C–H borylated products in terms of (a) phenylation and (b) alkenylation. | |
We predicted that one of the reasons for the wide substrate scope and high impurity tolerance of this metal-free directed borylation is that the reaction proceeds rapidly. To confirm this, we plotted the reaction profile for this reaction with yield vs. time (Fig. 3a), and were surprised to observe that the reaction gave the desired borylated product 1b in 70% yield, even after a reaction time of 1 minute at room temperature. This finding can be attributed to the high reactivity of BBr3 and the electron-rich arene system. Similar to the previously reported metal-free directed borylation using BBr3,7,8 the present reaction also appears to proceed through an electrophilic aromatic substitution pathway. To confirm this, two control experiments were performed, in which an electron-donating group substituted aniline derivative (10a or 2a) and an electron-withdrawing group substituted aniline derivative (18a or 22a) were reacted in a one-pot reaction. As expected, anilines with electron-donating groups gave higher product yields in both cases (Fig. 3b). However, the ratio of the product yields for electronically different sets of substrates were not as high as we expected. An additional substituent on the aniline substrate had only a minor influence on the reactivity because the substrate itself is an electron-rich system. To determine whether tetramethylpyrazine has any other role in this metal-free borylation except for abstracting a proton, we conducted 11B NMR studies. Unlike the previous observation in the reaction of 2,6-lutidine and BBr3, in which the generation of a mixture of an adduct complex and a borenium ion was observed,8,13 only one signal appeared in the negative region in the reaction of tetramethylpyrazine and BBr3, which corresponds to the formation of the adduct complex (49) (Fig. 3c).14 A similar signal was also observed in the reaction of 2-pyrimidylaniline 1a with BBr3. We were not able to confirm the exact species that is responsible for the reaction, due to the speed of the reaction.
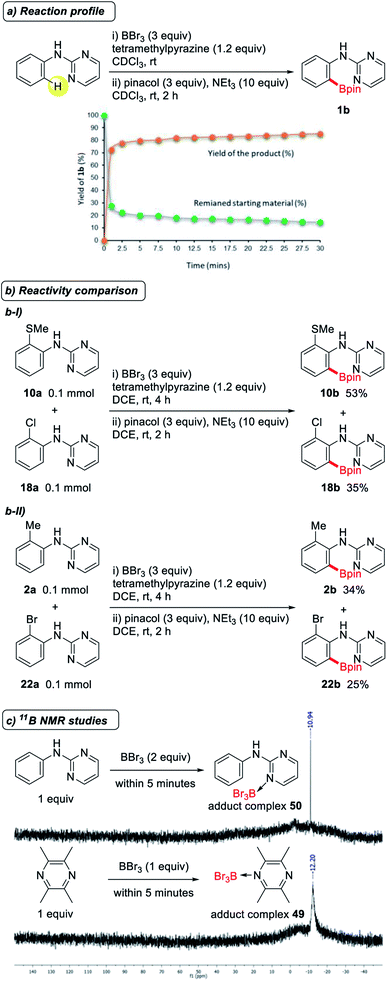 |
| Fig. 3 (a) Reaction profile of the metal-free directed borylation. (b) Reactivity comparison of electron rich and electron deficient functional group substituted anilines. (c) In situ11B NMR studies. | |
A proposed mechanism for this ortho-C–H borylation of 2-pyrimidylanilines is shown in Scheme 3. A Lewis acid–base adduct is initially formed between BBr3 and the N atom of a pyrimidyl group in 1a. A bromine transfer from 50 to another BBr3 molecule then leads to the formation of the borenium species 51.15 Due to the strong electrophilic nature of the aniline ring in 51, an electrophilic aromatic substitution proceeds readily to give a Wheland intermediate 52.7d Proton abstraction by a base then gives the dibromoboron complex 1c, which, upon quenching with pinacol and triethyl amine, provides the desired borylated product 1b. The results of 11B NMR experiments suggest that electrophilic borylation proceeds soon after a borenium species 51 is generated from the adduct 50, because of the electron-rich nature of 2-pyrimidylaniline. However, the possibility that a base accelerates disproportionation between 50 and BBr3 cannot be excluded. It is noteworthy that due to the speed of this reaction, it is not possible to trap or detect any of the other reactive intermediates.
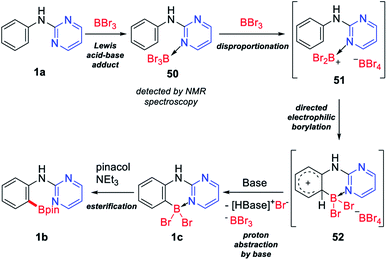 |
| Scheme 3 Plausible mechanism for metal-free directed C–H borylation of 2-pyrimidylaniline. | |
Conclusions
In summary, we report that a biologically important key structural motif of a pyrimidyl moiety functions efficiently as a directing group for the ortho-C–H borylation of aniline derivatives. Despite being a highly significant structural component, the C–H borylation of 2-pyrimidylanilines has not yet been achieved, even by a metal-catalysed process. Moreover, the high impurity tolerance of this process makes it the first choice for the synthesis of organoboron compounds. This approach can also be a useful protocol for producing a series of four-coordinated triarylborane derivatives containing a pyrimidyl moiety, which are of importance in material chemistry for use in OLED devices or as a Lewis acid catalyst. The protocol involves the use of readily available starting materials, can be carried out at room temperature without the need for external additives, and reaction times are minimal, thus being potentially useful as an industrial process. Finally, we conclude that the detailed study presented here represents a notable contribution in the field of synthetic organic chemistry as well as in organic materials chemistry, since it permits libraries of compounds containing key structural scaffolds to be produced.
Data availability
See ESI† for all experimental details including synthetic procedure and characterization.
Author contributions
S. R. conceptualized the work. Experimental work and data analysis were performed by S. R. and A. D. N. C. supervised the project. The manuscript was written with discussion and approval of all authors.
Conflicts of interest
There are no conflicts to declare.
Acknowledgements
This work was supported by a Grant in Aid for Specially Promoted Research by MEXT (17H06091). S. R. wishes to expresses his special thanks for a JSPS Postdoctoral Research Fellowship. We also wish to thank the Instrumental Analysis Centre, Faculty of Engineering, Osaka University, for assistance with the HRMS.
References
- For selected recent reviews on transition metal-catalysed directed C–H bond functionalization, see:
(a) C. Sambiagio, D. Schönbauer, R. Blieck, T. Dao-Huy, G. Pototschnig, P. Schaaf, T. Wiesinger, M. F. Zia, J. Wencel-Delord, T. Besset, B. U. W. Maes and M. Schnürch, Chem. Soc. Rev., 2018, 47, 6603–6743 RSC;
(b) A. Dey, S. K. Sinha, T. K. Achar and D. Maiti, Angew. Chem., Int. Ed., 2019, 58, 10820–10843 CrossRef CAS PubMed;
(c) P. Gandeepan, T. Müller, D. Zell, G. Cera, S. Warratz and L. Ackermann, Chem. Rev., 2019, 119, 2192–2452 CrossRef CAS PubMed;
(d) S. Rej, Y. Ano and N. Chatani, Chem. Rev., 2020, 120, 1788–1887 CrossRef CAS PubMed;
(e) J. I. Highamand and J. A. Bull, Org. Biomol. Chem., 2020, 18, 7291–7315 RSC;
(f) S. Rej, A. Das and N. Chatani, Coord. Chem. Rev., 2021, 431, 213683 CrossRef CAS.
- For papers on transition metal-catalyzed directed C–H bond alkylation of 2-pyrimidylanilines, see:
(a) N. K. Mishra, M. Choi, H. Jo, Y. Oh, S. Sharma, S. H. Han, T. Jeong, S. Han, S.-Y. Lee and I. S. Kim, Chem. Commun., 2015, 51, 17229–17232 RSC;
(b) G.-D. Tang, C.-L. Pan and X. Li, Org. Chem. Front., 2016, 3, 87–90 RSC;
(c) Z. Ruan, S. Lackner and L. Ackermann, Angew. Chem., Int. Ed., 2016, 55, 3153–3157 CrossRef CAS PubMed;
(d) S. Allu, M. Ravi and K. C. K. Swamy, Eur. J. Org. Chem., 2016, 5697–5705 CrossRef CAS;
(e) Z. Ruan, D. Ghorai, G. Zanoni and L. Ackermann, Chem. Commun., 2017, 53, 9113–9116 RSC;
(f) D. Ghorai, L. H. Finger, G. Zanoni and L. Ackermann, ACS Catal., 2018, 8, 11657–11662 CrossRef CAS.
- For selected papers on transition metal-catalyzed directed C–H bond functionalization of 2-pyrimidylanilines, see:
(a) W. Song and L. Ackermann, Chem. Commun., 2013, 49, 6638–6640 RSC;
(b) Z. Ruan, S. Lackner and L. Ackermann, ACS Catal., 2016, 6, 4690–4693 CrossRef CAS;
(c) G.-D. Tang, C.-L. Pan and F. Xie, Org. Biomol. Chem., 2016, 14, 2898–2904 RSC;
(d) T. Müller and L. Ackermann, Chem.–Eur. J., 2016, 22, 14151–14154 CrossRef PubMed;
(e) H. Jiang, S. Gao, J. Xu, X. Wu, A. Lin and H. Yao, Adv. Synth. Catal., 2016, 358, 188–194 CrossRef CAS;
(f) Z. Ruan, S. Lackner and L. Ackermann, Angew. Chem., Int. Ed., 2016, 55, 3153–3157 CrossRef CAS PubMed;
(g) L. Wang, Z. Yang, M. Yang, R. Zhang, C. Kuai and X. Cui, Org. Biomol. Chem., 2017, 15, 8302–8307 RSC;
(h) M. K. Manna, G. Bairy and R. Jana, J. Org. Chem., 2018, 83, 8390–8400 CrossRef CAS PubMed;
(i) S. M. Khake and N. Chatani, Org. Lett., 2020, 22, 3655–3660 CrossRef CAS PubMed;
(j) H. Shen, T. Liu, D. Cheng, X. Yi, Z. Wang, L. Liu, D. Song, F. Ling and W. Zhong, J. Org. Chem., 2020, 85, 13735–13746 CrossRef CAS PubMed;
(k) S. M. Khake, K. Yamazaki, Y. Ano and N. Chatani, ACS Catal., 2021, 11, 5463–5471 CrossRef CAS.
-
(a) A. Suzuki, Angew. Chem., Int. Ed., 2011, 50, 6723–6737 Search PubMed;
(b) I. P. Beletskaya, F. Alonso and V. Tyurin, Coord. Chem. Rev., 2019, 385, 137–173 CrossRef CAS;
(c) H. B. Chandrashekar, A. Maji, G. Halder, S. Banerjee, S. Bhattacharyya and D. Maiti, Chem. Commun., 2019, 55, 6201–6204 RSC;
(d) H. B. Chandrashekar, P. Dolui, B. Li, A. Mandal, H. Liu, S. Guin, H. Ge and D. Maiti, Angew. Chem., Int. Ed., 2021 DOI:10.1002/anie.202105204.
- For importance of 2-pyrimidylaniline moiety, see:
(a) E. Weisberg, P. Manley, J. Mestan, S. Cowan-Jacob, A. Ray and J. D. Griffin, Br. J. Cancer, 2006, 94, 1765–1769 CrossRef CAS PubMed;
(b) T. Panneer Selvam, C. Richa James, P. V. Dniandev and S. Karyn Valzita, Res. Pharm., 2012, 2, 1–9 Search PubMed;
(c) R. S. Dongre, A. R. Bhat and J. S. Meshram, Am. J. PharmTech Res., 2014, 4, 138–155 Search PubMed;
(d) R. Kaur, P. Kaur, S. Sharma, G. Singh, S. Mehndiratta, P. M. S. Bedi and K. Nepali, Recent Pat. Anti-Cancer Drug Discovery, 2015, 10, 23–71 CrossRef CAS PubMed;
(e) S. Kumar, A. Deep and B. Narasimhan, Cent. Nerv. Syst. Agents Med. Chem., 2015, 15, 5–10 CrossRef CAS PubMed.
- For reports on metal-free C–H borylation of in-built heterocyclic compounds, see:
(a) R. L. Letsinger and D. B. MacLean, J. Am. Chem. Soc., 1963, 85, 2230–2236 CrossRef CAS;
(b) F. A. Davis and M. J. S. Dewar, J. Am. Chem. Soc., 1968, 90, 3511–3515 CrossRef CAS;
(c) N. Ishida, T. Moriya, T. Goya and M. Murakami, J. Org. Chem., 2010, 75, 8709–8712 CrossRef CAS PubMed;
(d) Z. Zhao, Z. Chang, B. He, B. Chen, C. Deng, P. Lu, H. Qiu and B. Z. Tang, Chem.–Eur. J., 2013, 19, 11512–11517 CrossRef CAS PubMed;
(e) J. Chen, R. A. Lalancette and F. Ja, Organometallics, 2013, 32, 5843–5851 CrossRef CAS;
(f) D. L. Crossley, I. A. Cade, E. R. Clark, A. Escande, M. J. Humphries, S. M. King, I. Vitorica-Yrezabal, M. J. Ingleson and M. L. Turner, Chem. Sci., 2015, 6, 5144–5151 RSC;
(g) A. C. Shaikh, D. S. Ranade, S. Thorat, A. Maity, P. P. Kulkarni, R. G. Gonnade, P. Munshi and N. T. Patil, Chem. Commun., 2015, 51, 16115–16118 RSC;
(h) M. Yusuf, K. Liu, F. Guo, R. A. Lalancette and F. Jäkle, Dalt. Trans., 2016, 45, 4580–4587 RSC;
(i) D. L. Crossley, I. Vitorica-Yrezabal, M. J. Humphries, M. L. Turner and M. J. Ingleson, Chem.–Eur. J., 2016, 22, 12439–12448 CrossRef CAS PubMed;
(j) D. L. Crossley, L. Urbano, R. Neumann, S. Bourke, J. Jones, L. A. Dailey, M. Green, M. J. Humphries, S. M. King, M. L. Turner and M. J. Ingleson, ACS Appl. Mater. Interfaces, 2017, 9, 28243–28249 CrossRef CAS PubMed;
(k) K. Liu, R. A. Lalancette and F. Jäkle, J. Am. Chem. Soc., 2017, 139, 18170–18173 CrossRef CAS PubMed;
(l) B. P. Dash, I. Hamilton, D. J. Tate, D. L. Crossley, J. S. Kim, M. J. Ingleson and M. L. Turner, J. Mater. Chem. C, 2019, 7, 718–724 RSC;
(m) S. A. Iqbal, K. Yuan, J. Cid, J. Pahl and M. J. Ingleson, Org. Biomol. Chem., 2021, 19, 2949–2958 RSC.
- For reports on metal-free C–H borylation directed by removable auxiliary, see:
(a) L. Niu, H. Yang, R. Wang and H. Fu, Org. Lett., 2012, 14, 2618–2621 CrossRef CAS PubMed;
(b) C. Cazorla, T. S. De Vries and E. Vedejs, Org. Lett., 2013, 15, 984–987 CrossRef CAS PubMed;
(c) S. A. Iqbal, J. Cid, R. J. Procter, M. Uzelac, K. Yuan and M. J. Ingleson, Angew. Chem., Int. Ed., 2019, 58, 15381–15385 CrossRef CAS PubMed;
(d) J. Lv, X. Chen, X.-S. Xue, B. Zhao, Y. Liang, M. Wang, L. Jin, Y. Yuan, Y. Han, Y. Zhao, Y. Lu, J. Zhao, W. Y. Sun, K. N. Houk and Z. Shi, Nature, 2019, 575, 336–340 CrossRef CAS PubMed;
(e) G. Wu, X. Fu, Y. Wang, K. Deng, L. Zhang, T. Ma and Y. Ji, Org. Lett., 2020, 22, 7003–7007 CrossRef CAS PubMed;
(f) Z. J. Wang, X. Chen, L. Wu, J. J. Wong, Y. Liang, Y. Zhao, K. N. Houk and Z. Shi, Angew. Chem., Int. Ed., 2021, 60, 8500–8504 CrossRef CAS PubMed.
- For a report on transient directing group assisted metal-free C–H borylation, see: S. Rej and N. Chatani, J. Am. Chem. Soc., 2021, 143, 2920–2929 CrossRef CAS PubMed.
- For selected reports on the use of triarylborane as a catalyst, see:
(a) D. W. Stephan, Org. Biomol. Chem., 2008, 6, 1535–1539 RSC;
(b) V. Sumerin, F. Schulz, M. Atsumi, C. Wang, M. Nieger, M. Leskelä, T. Repo, P. Pyykkö and B. Rieger, J. Am. Chem. Soc., 2008, 130, 14117–14119 CrossRef CAS PubMed;
(c) V. Sumerin, K. Chernichenko, M. Nieger, M. Leskelä, B. Rieger and T. Repo, Adv. Synth. Catal., 2011, 353, 2093–2110 CrossRef CAS;
(d) K. Chernichenko, Á. Madarász, I. Pápai, M. Nieger, M. Leskelä and T. Repo, Nat. Chem., 2013, 5, 718–723 CrossRef CAS PubMed;
(e) M. Lindqvist, K. Borre, K. Axenov, B. Kótai, M. Nieger, M. Leskelä, I. Pápai and T. Repo, J. Am. Chem. Soc., 2015, 137, 4038–4041 CrossRef CAS PubMed;
(f) J. R. Lawson, L. C. Wilkins and R. L. Melen, Chem.–Eur. J., 2017, 23, 10997–11000 CrossRef CAS PubMed;
(g) K. A. Andrea and F. M. Kerton, ACS Catal., 2019, 9, 1799–1809 CrossRef CAS;
(h) A. Dasgupta, K. Stefkova, R. Babaahmadi, L. Gierlichs, A. Ariafard and R. L. Melen, Angew. Chem., Int. Ed., 2020, 59, 15492–15496 CrossRef CAS PubMed;
(i) J. L. Carden, A. Dasgupta and R. L. Melen, Chem. Soc. Rev., 2020, 49, 1706–1725 RSC.
- For selected reports on the importance of tetra-coordinated triarylborane compounds as organic materials, see:
(a) A. Wakamiya, T. Taniguchi and S. Yamaguchi, Angew. Chem., Int. Ed., 2006, 45, 3170–3173 CrossRef CAS PubMed;
(b) Y. Shirota and H. Kageyama, Chem. Rev., 2007, 107, 953–1010 CrossRef CAS PubMed;
(c) T. W. Hudnall, C.-W. Chiu and F. P. Gabbaï, Acc. Chem. Res., 2009, 42, 388–397 CrossRef CAS PubMed;
(d) Z. M. Hudson and S. Wang, Acc. Chem. Res., 2009, 42, 1584–1596 CrossRef CAS PubMed;
(e) F. Jäkle, Chem. Rev., 2010, 110, 3985–4022 CrossRef PubMed;
(f) C. R. Wade, A. E. J. Broomsgrove, S. Aldridge and F. P. Gabbaï, Chem. Rev., 2010, 110, 3958–3984 CrossRef CAS PubMed;
(g) D. Li, H. Zhang and Y. Wang, Chem. Soc. Rev., 2013, 42, 8416–8433 RSC;
(h) X. Li, X. Guo, L. Cao, Z. Xun, S. Wang, S. Li, Y. Li and G. Yang, Angew. Chem., Int. Ed., 2014, 53, 7809–7813 CrossRef CAS PubMed;
(i) V. F. Pais, M. M. Alcaide, R. Lõpez-Rodríguez, D. Collado, F. Nájera, E. Pérez-Inestrosa, E. Álvarez, J. M. Lassaletta, R. Fernández, A. Ros and U. Pischel, Chem.–Eur. J., 2015, 21, 15369–15376 CrossRef CAS PubMed;
(j) T. Kushida, A. Shuto, M. Yoshio, T. Kato and S. Yamaguchi, Angew. Chem., Int. Ed., 2015, 54, 6922–6925 CrossRef CAS PubMed;
(k) S. Griesbeck, Z. Zhang, M. Gutmann, T. Lühmann, R. M. Edkins, G. Clermont, A. N. Lazar, M. Haehnel, K. Edkins, A. Eichhorn, M. Blanchard-Desce, L. Meinel and T. B. Marder, Chem.–Eur. J., 2016, 22, 14701–14706 CrossRef CAS PubMed;
(l) G. Turkoglu, M. E. Cinar and T. Ozturk, Molecules, 2017, 22, 1522 CrossRef PubMed;
(m) R. Hecht, J. Kade, D. Schmidt and A. Nowak-Król, Chem.–Eur. J., 2017, 23, 11620–11628 CrossRef CAS PubMed.
- K. Yang, G. Zhang and Q. Song, Chem. Sci., 2018, 9, 7666–7672 RSC.
- For reports on robustness and sensitivity screening of metal catalyzed synthetic process, see:
(a) K. D. Collins and F. Glorius, Nat. Chem., 2013, 5, 597–601 CrossRef CAS PubMed;
(b) K. D. Collins and F. Glorius, Acc. Chem. Res., 2015, 48, 619–627 CrossRef CAS PubMed;
(c) T. Gensch and F. Glorius, Science, 2016, 352, 294–295 CrossRef CAS PubMed;
(d) L. Pitzer, F. Schäfers and F. Glorius, Angew. Chem., Int. Ed., 2019, 58, 8572–8576 CrossRef CAS PubMed;
(e) S. D. Friis, M. J. Johansson and L. Ackermann, Nat. Chem., 2020, 12, 511–519 CrossRef CAS PubMed.
- S. Tanaka, Y. Saito, T. Yamamoto and T. Hattori, Org. Lett., 2018, 20, 1828–1831 CrossRef CAS PubMed.
- For 11B NMR signal of a pyridine–BBr3 adduct complex, see: M. J. Farquharson and J. S. Hartman, Can. J. Chem., 1996, 74, 1309–1320 CrossRef CAS.
- For a review on borenium chemistry, see: T. S. De Vries, A. Prokofjevs and E. Vedejs, Chem. Rev., 2011, 112, 4246–4282 CrossRef PubMed.
Footnote |
† Electronic supplementary information (ESI) available: Experimental details and spectroscopic data. See DOI: 10.1039/d1sc02937a |
|
This journal is © The Royal Society of Chemistry 2021 |
Click here to see how this site uses Cookies. View our privacy policy here.