DOI:
10.1039/D1SC02905C
(Edge Article)
Chem. Sci., 2021,
12, 12971-12976
Electrooxidative o-carborane chalcogenations without directing groups: cage activation by copper catalysis at room temperature†
Received
30th May 2021
, Accepted 28th August 2021
First published on 1st September 2021
Abstract
Copper-catalyzed electrochemical direct chalcogenations of o-carboranes was established at room temperature. Thereby, a series of cage C-sulfenylated and C-selenylated o-carboranes anchored with valuable functional groups was accessed with high levels of position- and chemo-selectivity control. The cupraelectrocatalysis provided efficient means to activate otherwise inert cage C–H bonds for the late-stage diversification of o-carboranes.
Carboranes are polyhedral molecular boron–carbon clusters, which display unique properties, such as a boron enriched content, icosahedron geometry and three-dimensional electronic delocalization.1 These features render carboranes as valuable building blocks for applications to optoelectronics,2 as nanomaterials, in supramolecular design,3 organometallic coordination chemistry,4 and boron neutron capture therapy (BNCT) agents.5 As a consequence, considerable progress has been witnessed in transition metal-catalyzed regioselective cage B–H functionalization of o-carboranes6 and different functional motifs have been incorporated into the cage boron vertices.7–10 However, progress in this research arena continues to be considerably limited by the shortage of robust and efficient methods to access carborane-functionalized molecules. While C–S bonds are important structural motifs in various biologically active molecules and functional materials,11 strategies for the assembly of chalcogen-substituted carboranes continue to be scarce. A major challenge is hence represented by the strong coordination abilities of thiols to most transition metals, which often lead to catalyst deactivation.12 While copper-catalyzed B(4,5)–H disulfenylation of o-carboranes was achieved,7e elevated reaction temperature was required, and 8-aminoquinoline was necessary as bidentate directing group. The bidentate directing group13 needs to be installed and removed, which jeopardizes the overall efficacy. Likewise, an organometallic strategy was recently devised for cysteine borylation with a stoichiometric platinum(II)-based carboranes.14 Meanwhile, oxidative cage B/C–H functionalizations largely call for noble transition metal catalysts15 and stoichiometric amounts of chemical oxidants, such as expensive silver(I) salts.16
In recent years, electricity has been identified as an increasingly viable, sustainable redox equivalent for environmentally-benign molecular synthesis.17,18 While significant advances have been realized by the merger of electrocatalysis with organometallic bond activation,19 electrochemical carborane functionalizations continue unfortunately to be underdevelopment. In sharp contrast, we have now devised a strategy for unprecedented copper-catalyzed electrochemical cage C–H chalcogenations of o-carboranes in a dehydrogenative manner, assembling a variety of C-sulfenylated and C-selenylated o-carboranes (Fig. 1a). It is noteworthy that our electrochemical cage C–S/Se modification approach is devoid of chemical oxidants, and does not need any directing groups, operative at room temperature.
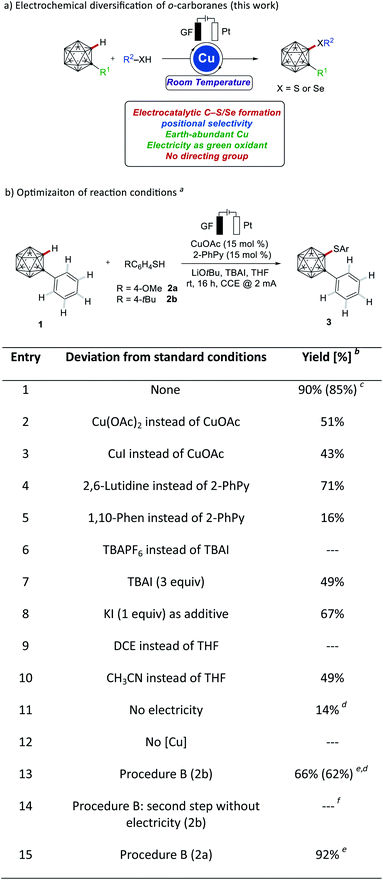 |
| Fig. 1 Electrochemical diversification of o-carboranes and optimization of reaction conditions. aReaction conditions: procedure A: 1a (0.10 mmol), 2a (0.3 mmol), CuOAc (15 mol%), 2-PhPy (15 mol%), LiOtBu (0.2 mmol), TBAI (2.0 equiv.), solvent (3 mL), platinum cathode (10 mm × 15 mm × 0.25 mm), graphite felt (GF) anode (10 mm × 15 mm × 6 mm), 2 mA, under air, r.t., 16 h. bYield was determined by 1H NMR with CH2Br2 as the internal standard. cIsolated yields in parenthesis. dKI (1.0 equiv.) as additive. eProcedure B: 2 (0.3 mmol), LiOtBu (0.2 mmol), TBAI (2.0 equiv.), solvent (3.0 mL), 2 mA, r.t., 3 h, then adding 1a (0.10 mmol), 2-PhPy (15 mol%), CuOAc (15 mol%), 2 mA, rt, 16 h. f2b (0.3 mmol), LiOtBu (0.2 mmol), KI (1.0 equiv.), TBAI (2.0 equiv.), solvent (3.0 mL), 2 mA, r.t., 3 h, then adding 1a (0.10 mmol), 2-PhPy (15 mol%), CuOAc (15 mol%), r.t., 16 h. TBAI = tetrabutylammonium iodide, TBAPF6 = tetrabutylammonium hexafluorophosphate. DCE = 1,2-dichloroethane, THF = tetrahydrofuran. | |
We commenced our studies by probing various reaction conditions for the envisioned copper-catalyzed cage C–H thiolation of o-carborane in an operationally simple undivided cell setup equipped with a GF (graphite felt) anode and a Pt cathode (Fig. 1b and Table S1†). After extensive experimentation, we observed that the thiolation of substrate 1 proceeded efficiently with catalytic amounts of CuOAc and 2-phenylpyridine, albeit in the presence of 2 equivalents LiOtBu as the base, and 2 equivalents n-Bu4NI as the electrolyte at room temperature under a constant current of 2 mA (entry 1). The yield was reduced when other copper sources or additives were used (entries 2–5). Surprisingly, n-Bu4NPF6 as the electrolyte failed to facilitate the carborane modification, indicating that n-Bu4NI operates not only as electrolyte, but also as a redox mediator (entry 6). Altering the stoichiometry of the electrolyte or using KI did not improve the performance (entries 7–8). Product formation was not observed, when the reaction was conducted with DCE as the solvent, while CH3CN resulted in a drop of the catalytic performance (entries 9–10). Control experiments confirmed the essential role of the electricity and the catalyst (entries 11–12), while a sequential procedure was found to be beneficial (entries 13–15).
With the optimized reaction conditions in hand, we explored the versatility of the cage C–H thiolation of o-carborane 1a with different thiols 2 (Scheme 1). Electron-rich as well as electron-deficient substituents on the arenes were found to be amenable to the electrocatalyzed C–H activation, providing the corresponding thiolation products 3aa–3ao in good to excellent yields. Thereby, a variety of synthetically useful functional groups, such as fluoro (3ae, 3am), chloro (3af, 3ak, 3an) and bromo (3ag, 3al), were fully tolerated, which should prove instrumental for further late-stage manipulations. Various disubstituted aromatic and heterocyclic thiols afforded the corresponding cage C–S modified products 3ap–3as. Notably, aliphatic thiols efficiently underwent the electrochemical transformation to provide the corresponding cage alkylthiolated products 3at–3au. Notably, the halogen-containing thiols (2e–2f, 2k–2n and 2q) reacted selectively with o-carboranes to deliver the desired products without halide coupling byproducts being observed. The connectivity of the products 3aa, 3am and 3ao was unambiguously verified by X-ray single crystal diffraction analysis.22
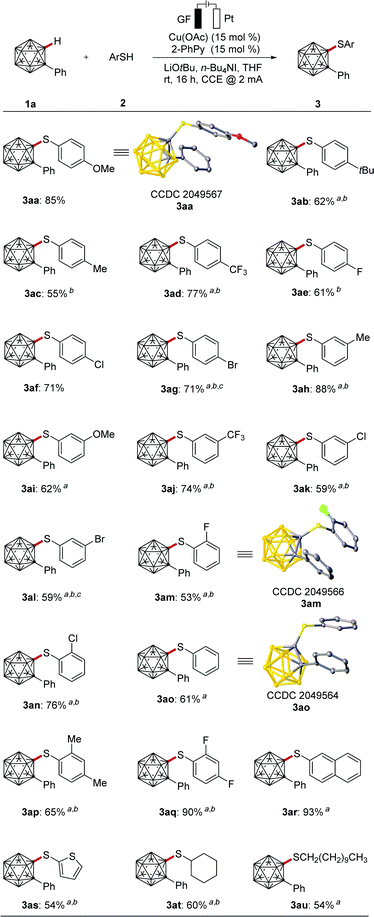 |
| Scheme 1 Electrochemical C–H thiolation of o-carborane 1a. (a) Procedure B. (b) KI (1 equiv.). (c) Cul as the catalyst. | |
Encouraged by the efficiency of the cupraelectro-oxidative cage C–H thiolation, we became intrigued to explore the chalcogenantion of differently-decorated o-carboranes 1 (Scheme 2). Electronically diverse carboranes 1 served as competent coupling partners, giving the corresponding thiolation products 4bo–4do with high levels of efficacy in position-selective manner. The strategy was not restricted to phenyl-substituted o-carboranes. Indeed, substrates bearing benzyl and even alkyl groups also performed well to deliver the desired products 4eo–4ga. It is noteworthy that the C–H activation approach was also compatible with selenols to give the o-carboranes 4av–4fv. The molecular structures of the carborane 4br and 4av were unambiguously verified by single-crystal X-ray diffraction.22
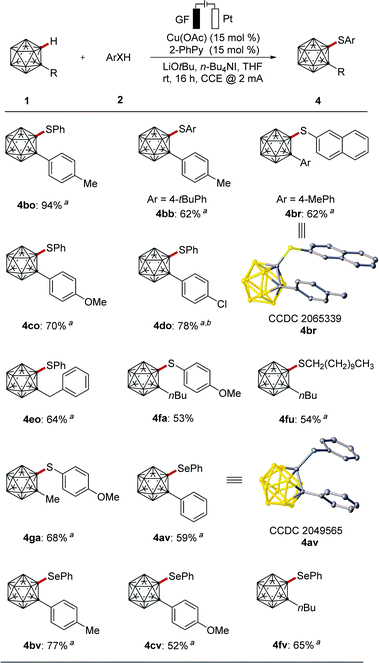 |
| Scheme 2 Electrochemical cage C–H chalcogenation of o-carboranes. (a) Procedure B. (b) KI (1 equiv.). | |
Scaffold functionalization of the thus obtained carborane 3ag provided the alkynylated derivative 5a and amine 5b (Scheme 3), giving access to carborane-based host materials of relevant to phosphorescent organic light-emitting diodes.20
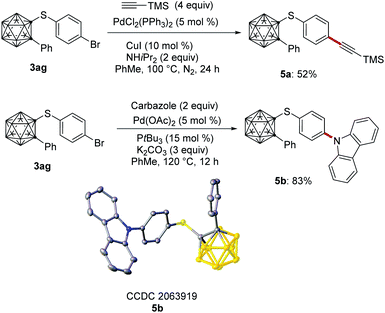 |
| Scheme 3 Late-stage diversification. | |
Next, we became attracted to delineating the mode of the cupraelectro-catalyzed cage C–H chalcogenation. To this end, control experiments were performed (Scheme 4a). First, electrocatalysis in the presence of TEMPO or Ph2C
CH2 gave the desired product 3aa. EPR studies of thiol 2a, LiOtBu and THF under the electrochemical conditions showed a small radical signal, which might be attributed to a thiol radical.21 Second, the cupraelectrocatalysis occurred efficiently in the dark. Third, detailed cyclovoltammetric analysis of the thiol and iodide mediator (Scheme 4b and ESI†)21 revealed an irreversible oxidation of the thiol anion at Ep = −0.62 V vs. Ag/Ag+ and two oxidation events for the iodide, including an irreversible oxidation at Ep = 0.12 V vs. Ag/Ag+ and a reversible oxidation at Ep = 0.44 V vs. Ag/Ag+, which is in good agreement with the literature reported iodide oxidation potentials,18c,d and is suggestive of the preferential oxidation of the iodide as a redox mediator. In this context, the use of n-Bu4NI as a redox mediator to achieve copper-catalyzed electrochemical arene C–H aminations had been documented.18d Furthermore, we calculated the redox potential of complex C by means of DFT calculations at the PW6B95-D4/def2-TZVP + SMD(MeCN)//TPSS-D3BJ/def2-SVP level of theory.21 These studies revealed a calculated oxidation half-wave potential for complex C is Eo,calc1/2 = −0.08 V vs. SCE. Hence, iodide is a competent redox mediator to achieve the transformation from complex C to complex D. Analysis of non-covalent interactions21 in complex C (Fig. 2) show the presence of a weak stabilization interaction between the chalcogen's anisole group and the 2-phenylpyridine. In contrast, in complex D these interactions were found more relevant between the o-carborane phenyl group and the chalcogen aromatic motif.
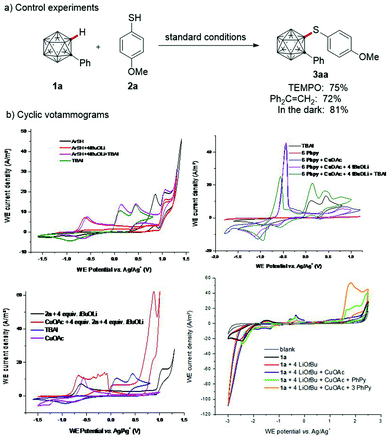 |
| Scheme 4 Control experiments and cyclic voltammograms. | |
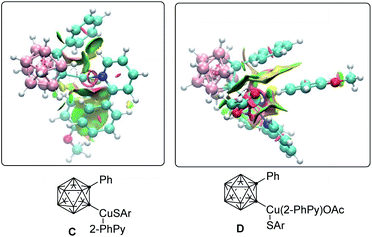 |
| Fig. 2 Non-covalent interaction plots for the complexes C and D. Strong attractive interactions are shown in blue, weak attractive interactions are given in green, while red corresponds to repulsive interactions. Ar = 4-MeOC6H4. | |
On the basis of the aforementioned findings,18 a plausible reaction mechanism is proposed in Scheme 5, which commences with an anodic single electron-transfer (SET) oxidation of the thiol anion E to form the sulfur-centered radical F. Subsequently, the copper(I) species A reacts with the sulfur radical F to deliver copper(II) complex B, which next reacts with o-carborane 1 in the presence of LiOtBu to generate a copper(II)-o-carborane complex C. Thereafter, the complex C is oxidized by the anodically generated redox mediator I2 to furnish the copper(III) species D,18d which subsequently undergoes reductive elimination, affording the final product and regenerating the catalytically active complex A. Alternatively, the direct oxidation of copper(II) complex C by electricity to generate copper(III) species D can not be excluded at this stage.18a,b
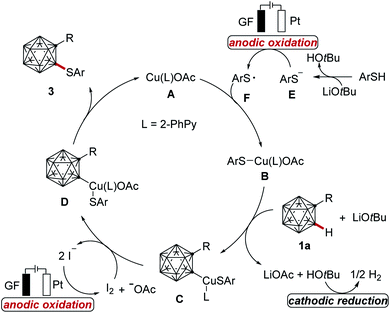 |
| Scheme 5 Proposed reaction mechanism. | |
In conclusion, a sustainable electrocatalytic C–H chalcogenation of o-carboranes with thiols and selenols was realized at room temperature by earth abundant copper catalysis. The C–H activation was characterized by mild reaction conditions and high functional group tolerance, leading to the facile assembly of various o-carboranes. Thereby, a transformative platform for the design of cage C–S and C–Se o-carboranes was established that avoids chemical oxidants by environmentally-sound electricity in the absence of directing groups. A plausible mechanism of paired electrolysis was established by detailed mechanistic studies.
Data availability
All experimental data, procedures for data analysis and pertinent data sets are provided in the ESI.†
Author contributions
L. Y. and L. A. conceived the project. L. Y. and B. B. J. performed the experiments. A. S. performed CV studies. B. Y. performed DFT calculations. A. C. S. performed EPR studies. L. Y. and L. A. wrote the manuscript. All of the authors discussed the results and contributed to the preparation of the final manuscript.
Conflicts of interest
The authors declare no conflict of interest.
Acknowledgements
Generous support by the CSC (fellowship to L. Y. and B. Y.), the DAAD (fellowship to B. B. J.) and the DFG (Gottfried-Wilhelm-Leibniz prize to L. A.) is gratefully acknowledged. We also thank Dr Christopher Golz (University Göttingen) for great support with the X-ray diffraction analysis.
References
-
(a)
R. N. Grimes, Carboranes, Academic Press, Amsterdam, 3rd edn, 2016 Search PubMed;
(b) J. Poater, M. Solà, C. Viñas and F. Teixidor, Angew. Chem., Int. Ed., 2014, 53, 12191–12195 CrossRef CAS PubMed;
(c)
N. S. Hosmane, Boron Science: New Technologies and Applications, Taylor & Francis Books/CRC Press, Boca Raton, FL, 2012 Search PubMed.
-
(a) S. Mukherjee and P. Thilagar, Chem. Commun., 2016, 52, 1070–1093 RSC;
(b) R. Núñez, M. Tarrés, A. Ferrer-Ugalde, F. F. de Biani and F. Teixidor, Chem. Rev., 2016, 116, 14307–14378 CrossRef PubMed;
(c) X. Li, H. Yan and Q. Zhao, Chem.–Eur. J., 2016, 22, 1888–1898 CrossRef CAS PubMed.
-
(a) E. Q. Qian, A. I. Wixtrom, J. C. Axtell, A. Saebi, P. Rehak, Y. Han, E. H. Moully, D. Mosallaei, S. Chow, M. Messina, J.-Y. Wang, A. T. Royappa, A. L. Rheingold, H. D. Maynard, P. Kral and A. M. Spokoyny, Nat. Chem., 2017, 9, 333–340 CrossRef CAS PubMed;
(b) A. Saha, E. Oleshkevich, C. Viñas and F. Teixidor, Adv. Mater., 2017, 29, 1704238–1704245 CrossRef PubMed;
(c) A. C. Serino, M. E. Anderson, L. M. A. Saleh, R. M. Dziedzic, H. Mills, L. K. Heidenreich, A. M. Spokoyny and P. S. Weiss, ACS Appl. Mater. Interfaces, 2017, 9, 34592–34596 CrossRef CAS PubMed;
(d) J. J. Schwartz, A. M. Mendoza, N. Wattanatorn, Y. Zhao, V. T. Nguyen, A. M. Spokoyny, C. A. Mirkin, T. Baše and P. S. Weiss, J. Am. Chem. Soc., 2016, 138, 5957–5967 CrossRef CAS PubMed;
(e) R. N. Grimes, Dalton Trans., 2015, 44, 5939–5956 RSC;
(f) D. Brusselle, P. Bauduin, L. Girard, A. Zaulet, C. Viñas, F. Teixidor, I. Ly and O. Diat, Angew. Chem., Int. Ed., 2013, 52, 12114–12118 CrossRef CAS PubMed;
(g) A. M. Cioran, A. D. Musteti, F. Teixidor, Ž. Krpetić, I. A. Prior, Q. He, C. J. Kiely, M. Brust and C. Viñas, J. Am. Chem. Soc., 2012, 134, 212–221 CrossRef CAS PubMed;
(h) P. Bauduin, S. Prevost, P. Farràs, F. Teixidor, O. Diat and T. Zemb, Angew. Chem., Int. Ed., 2011, 50, 5298–5300 CrossRef CAS PubMed;
(i) B. P. Dash, R. Satapathy, E. R. Gaillard, J. A. Maguire and N. S. Hosmane, J. Am. Chem. Soc., 2010, 132, 6578–6587 CrossRef CAS PubMed;
(j) C. J. Villagómez, T. Sasaki, J. M. Tour and L. Grill, J. Am. Chem. Soc., 2010, 132, 16848–16854 CrossRef PubMed.
-
(a) S. P. Fisher, A. W. Tomich, J. Guo and V. Lavallo, Chem. Commun., 2019, 55, 1684–1701 RSC;
(b) Y.-P. Zhou, S. Raoufmoghaddam, T. Szilvási and M. Driess, Angew. Chem., Int. Ed., 2016, 55, 12868–12872 CrossRef CAS PubMed;
(c) M. Hailmann, N. Wolf, R. Renner, T. C. Schäfer, B. Hupp, A. Steffen and M. Finze, Angew. Chem., Int. Ed., 2016, 55, 10507–10511 CrossRef CAS PubMed;
(d) R. D. Adams, J. Kiprotich, D. V. Peryshkov and Y. O. Wong, Chem.–Eur. J., 2016, 22, 6501–6504 CrossRef CAS PubMed;
(e) A. El-Hellani and V. Lavallo, Angew. Chem., Int. Ed., 2014, 53, 4489–4493 CrossRef CAS PubMed;
(f) M. Joost, A. Zeineddine, L. Estévez, S. Mallet-Ladeira, K. Miqueu, A. Amgoune and D. Bourissou, J. Am. Chem. Soc., 2014, 136, 14654–14657 CrossRef CAS PubMed;
(g) Z.-J. Yao and G.-X. Jin, Coord. Chem. Rev., 2013, 257, 2522–2535 CrossRef CAS;
(h) Z. Qiu, S. Ren and Z. Xie, Acc. Chem. Res., 2011, 44, 299–309 CrossRef CAS PubMed.
-
(a) S. P. Fisher, A. W. Tomich, S. O. Lovera, J. F. Kleinsasser, J. Guo, M. J. Asay, H. M. Nelson and V. Lavallo, Chem. Rev., 2019, 119, 8262–8290 CrossRef CAS PubMed;
(b) A. F. Armstrong and J. F. Valliant, Dalton Trans., 2007, 4240–4251 RSC.
-
(a) X. Zhang and H. Yan, Coord. Chem. Rev., 2019, 378, 466–482 CrossRef CAS;
(b) Y. Quan and Z. Xie, Chem. Soc. Rev., 2019, 48, 3660–3673 RSC;
(c) Y. Quan, C. Tang and Z. Xie, Dalton Trans., 2019, 48, 7494–7498 RSC;
(d) R. M. Dziedzic and A. M. Spokoyny, Chem. Commun., 2019, 55, 430–442 RSC;
(e) R. M. Dziedzic, J. C. Axtell, A. L. Rheingold and A. M. Spokoyny, Org. Process Res. Dev., 2019, 23, 1638–1645 CrossRef CAS PubMed;
(f) Y. Quan, Z. Qiu and Z. Xie, Chem.–Eur. J., 2018, 24, 2795–2805 CrossRef CAS PubMed;
(g) W.-B. Yu, P.-F. Cui, W.-X. Gao and G.-X. Jin, Coord. Chem. Rev., 2017, 350, 300–319 CrossRef CAS.
- Selected examples:
(a) H. Ni, Z. Lu and Z. Xie, J. Am. Chem. Soc., 2020, 142, 18661–18667 CrossRef CAS PubMed;
(b) Y. Chen, Y. Quan and Z. Xie, Chem. Commun., 2020, 56, 7001–7004 RSC;
(c) Y. Ge, J. Zhang, Z. Qiu and Z. Xie, Angew. Chem., Int. Ed., 2020, 59, 4851–4855 CrossRef CAS PubMed;
(d) Y. K. Au, H. Lyu, Y. Quan and Z. Xie, J. Am. Chem. Soc., 2020, 142, 6940–6945 CrossRef CAS PubMed;
(e) Y. Chen, Y. Quan and Z. Xie, Chem. Commun., 2020, 56, 12997–13000 RSC;
(f) H. Lyu, J. Zhang, J. Yang, Y. Quan and Z. Xie, J. Am. Chem. Soc., 2019, 141, 4219–4224 CrossRef CAS;
(g) Y. K. Au, H. Lyu, Y. Quan and Z. Xie, J. Am. Chem. Soc., 2019, 141, 12855–12862 CrossRef CAS PubMed;
(h) R. Cheng, B. Li, J. Wu, J. Zhang, Z. Qiu, W. Tang, S.-L. You, Y. Tang and Z. Xie, J. Am. Chem. Soc., 2018, 140, 4508–4511 CrossRef CAS PubMed;
(i) R. Cheng, Z. Qiu and Z. Xie, Nat. Commun., 2017, 8, 14827 CrossRef CAS PubMed.
- Selected examples:
(a) H. A. Mills, J. L. Martin, A. L. Rheingold and A. M. Spokoyny, J. Am. Chem. Soc., 2020, 142, 4586–4591 CrossRef CAS PubMed;
(b) J. M. Stauber, E. A. Qian, Y. Han, A. L. Rheingold, P. Král, D. Fujita and A. M. Spokoyny, J. Am. Chem. Soc., 2020, 142, 327–334 CrossRef CAS PubMed;
(c) J. C. Axtell, K. O. Kirlikovali, P. I. Djurovich, D. Jung, V. T. Nguyen, B. Munekiyo, A. T. Royappa, A. L. Rheingold and A. M. Spokoyny, J. Am. Chem. Soc., 2016, 138, 15758–15765 CrossRef CAS PubMed;
(d) R. M. Dziedzic, L. M. Saleh, J. C. Axtell, J. L. Martin, S. L. Stevens, A. T. Royappa, A. L. Rheingold and A. M. Spokoyny, J. Am. Chem. Soc., 2016, 138, 9081–9084 CrossRef CAS PubMed.
-
(a) I. Bennour, F. Teixidor, Z. Kelemen and C. Vinas, Molecules, 2020, 25, 2814 CrossRef CAS PubMed;
(b) A. B. Buades, Z. Kelemen, V. S. Arderiu, A. Zaulet, C. Vinas and F. Teixidor, Dalton Trans., 2020, 49, 3525–3531 RSC;
(c) A. B. Buades, V. S. Arderiu, D. Olid-Britos, C. ViÇas, R. Sillanp, M. Haukka, X. Fontrodona, M. Paradinas, C. Ocal and F. Teixidor, J. Am. Chem. Soc., 2018, 140, 2957–2970 CrossRef CAS PubMed.
- Selected examples:
(a) H. Yan, M. Chen, D. Zhao, J. Xu, C. Li and C. Lu, Angew. Chem., Int. Ed., 2021, 60, 7917–7923 Search PubMed;
(b) L. Yang, B. Bongsuiru Jei, A. Scheremetjew, R. Kuniyil and L. Ackermann, Angew. Chem., Int. Ed., 2021, 60, 1482–1487 CrossRef CAS PubMed;
(c) Y.-F. Liang, L. Yang, B. B. Jei, R. Kuniyil and L. Ackermann, Chem. Sci., 2020, 11, 10764–10769 RSC;
(d) Y. Baek, K. Cheong, G. H. Ko, G. U. Han, S. H. Han, D. Kim, K. Lee and P. H. Lee, J. Am. Chem. Soc., 2020, 142, 9890–9895 CrossRef CAS PubMed;
(e) Z.-Y. Zhang, X. Zhang, J. Yuan, C.-D. Yue, S. Meng, J. Chen, G.-A. Yu and C.-M. Che, Chem.–Eur. J., 2020, 26, 5037–5050 CrossRef CAS PubMed;
(f) Y. Baek, S. Kim, J.-Y. Son, K. Lee, D. Kim and P. H. Lee, ACS Catal., 2019, 9, 10418–10425 CrossRef CAS;
(g) T.-T. Xu, K. Cao, C.-Y. Zhang, J. Wu, L.-F. Ding and J. Yang, Org. Lett., 2019, 21, 9276–9279 CrossRef CAS PubMed;
(h) Z. Yang, W. Zhao, W. Liu, X. Wei, M. Chen, X. Zhang, X. Zhang, Y. Liang, C. Lu and H. Yan, Angew. Chem., Int. Ed., 2019, 58, 11886–11892 CrossRef CAS PubMed;
(i) X. Zhang and H. Yan, Chem. Sci., 2018, 9, 3964–3969 RSC;
(j) X. Zhang, H. Zheng, J. Li, F. Xu, J. Zhao and H. Yan, J. Am. Chem. Soc., 2017, 139, 14511–14517 CrossRef CAS PubMed;
(k) K. Cao, T.-T. Xu, J. Wu, L. Jiang and J. Yang, Chem. Commun., 2016, 52, 11446–11449 RSC.
-
(a) A. Gangjee, Y. B. Zeng, T. Talreja, J. J. McGuire, R. L. Kisliuk and S. F. Queener, J. Med. Chem., 2007, 50, 3046–3053 CrossRef CAS PubMed;
(b) Z. Y. Sun, E. Botros, A. D. Su, Y. Kim, E. J. Wang, N. Z. Baturay and C. H. Kwon, J. Med. Chem., 2000, 43, 4160–4168 CrossRef CAS PubMed.
-
(a) V. Hirschbeck, P. H. Gehrtz and I. Fleischer, Chem.–Eur. J., 2018, 24, 7092–7107 CrossRef CAS PubMed;
(b) I. P. Beletskaya and V. P. Ananikov, Chem. Rev., 2011, 111, 1596–1636 CrossRef CAS PubMed;
(c) M. Mellah, A. Voituriez and E. Schulz, Chem. Rev., 2007, 107, 5133–5209 CrossRef CAS PubMed.
- S. Rej, Y. Ano and N. Chatani, Chem. Rev., 2020, 120, 1788–1887 CrossRef CAS PubMed.
-
(a) M. A. Waddington, X. Zheng, J. M. Stauber, E. Hakim Moully, H. R. Montgomery, L. M. A. Saleh, P. Král and A. M. Spokoyny, J. Am. Chem. Soc., 2021, 143, 8661–8668 CrossRef CAS PubMed;
(b) L. M. A. Saleh, R. M. Dziedzic, S. I. Khan and A. M. Spokoyny, Chem.–Eur. J., 2016, 22, 8466–8470 CrossRef CAS PubMed.
- Selected examples:
(a) F. Lin, J.-L. Yu, Y. Shen, S.-Q. Zhang, B. Spingler, J. Liu, X. Hong and S. Duttwyler, J. Am. Chem. Soc., 2018, 140, 13798–13807 CrossRef CAS PubMed;
(b) T.-T. Xu, K. Cao, C.-Y. Zhang, J. Wu, L. Jiang and J. Yang, Chem. Commun., 2018, 54, 13603–13606 RSC;
(c) R. M. Dziedzic, J. L. Martin, J. C. Axtell, L. M. A. Saleh, T.-C. Ong, Y.-F. Yang, M. S. Messina, A. L. Rheingold, K. N. Houk and A. M. Spokoyny, J. Am. Chem. Soc., 2017, 139, 7729–7732 CrossRef CAS PubMed;
(d) H. Lyu, Y. Quan and Z. Xie, Angew. Chem., Int. Ed., 2016, 55, 11840–11844 CrossRef CAS PubMed;
(e) B. J. Eleazer, M. D. Smith, A. A. Popov and D. V. Peryshkov, J. Am. Chem. Soc., 2016, 138, 10531–10538 CrossRef CAS PubMed;
(f) Y. Quan and Z. Xie, J. Am. Chem. Soc., 2014, 136, 15513–15516 CrossRef CAS PubMed.
- Selected examples:
(a) J. Wu, K. Cao, C. Y. Zhang, T. T. Xu, X. Y. Wen, B. Li and J. Yang, Inorg. Chem., 2020, 59, 17340–17346 CrossRef CAS PubMed;
(b) C. Zhang, Q. Wang, S. Tian, J. Zhang, J. Li, L. Zhou and J. Lu, Org. Biomol. Chem., 2020, 18, 4723–4727 RSC;
(c) C.-X. Cui, J. Zhang, Z. Qiu and Z. Xie, Dalton Trans., 2020, 49, 1380–1383 RSC;
(d) Y. Shen, K. Zhang, X. Liang, R. Dontha and S. Duttwyler, Chem. Sci., 2019, 10, 4177–4184 RSC;
(e) Y. Chen, Y. K. Au, Y. Quan and Z. Xie, Sci. China: Chem., 2018, 62, 74–79 CrossRef.
-
(a) P. Gandeepan, L. H. Finger, T. H. Meyer and L. Ackermann, Chem.
Soc. Rev., 2020, 49, 4254–4272 RSC;
(b) M. Elsherbini and T. Wirth, Acc. Chem. Res., 2019, 52, 3287–3296 CrossRef CAS;
(c) P. Xiong and H.-C. Xu, Acc. Chem. Res., 2019, 52, 3339–3350 CrossRef CAS;
(d) Y. Yuan and A. Lei, Acc. Chem. Res., 2019, 52, 3309–3324 CrossRef CAS PubMed;
(e) M. Ghosh, V. S. Shinde and M. Rueping, Beilstein J. Org. Chem., 2019, 15, 2710–2746 CrossRef CAS PubMed;
(f) S. R. Waldvogel, S. Lips, M. Selt, B. Riehl and C. J. Kampf, Chem. Rev., 2018, 118, 6706–6765 CrossRef CAS PubMed;
(g) J. E. Nutting, M. Rafiee and S. S. Stahl, Chem. Rev., 2018, 118, 4834–4885 CrossRef CAS;
(h) G. S. Sauer and S. Lin, ACS Catal., 2018, 8, 5175–5187 CrossRef CAS;
(i) R. Feng, J. A. Smith and K. D. Moeller, Acc. Chem. Res., 2017, 50, 2346–2352 CrossRef CAS PubMed;
(j) R. Francke and R. D. Little, Chem. Soc. Rev., 2014, 43, 2492–2521 RSC;
(k) A. Jutand, Chem. Rev., 2008, 108, 2300–2347 CrossRef CAS PubMed.
-
(a) C. Tian, U. Dhawa, A. Scheremetjew and L. Ackermann, ACS Catal., 2019, 9, 7690–7696 CrossRef CAS;
(b) S. Kathiravan, S. Suriyanarayanan and I. A. Nicholls, Org. Lett., 2019, 21, 1968–1972 CrossRef CAS PubMed;
(c) F. Wang and S. S. Stahl, Angew. Chem., Int. Ed., 2019, 58, 6385–6390 CrossRef CAS PubMed;
(d) Q. L. Yang, X. Y. Wang, J. Y. Lu, L. P. Zhang, P. Fang and T. S. Mei, J. Am. Chem. Soc., 2018, 140, 11487–11494 CrossRef CAS PubMed.
-
(a) L. Ackermann, Acc. Chem. Res., 2020, 53, 84–104 CrossRef CAS;
(b) T. H. Meyer, L. H. Finger, P. Gandeepan and L. Ackermann, Trends Chem., 2019, 1, 63–76 CrossRef CAS;
(c) Q.-L. Yang, P. Fang and T.-S. Mei, Chin. J. Chem., 2018, 36, 338–352 CrossRef CAS;
(d) C. Ma, P. Fang and T.-S. Mei, ACS Catal., 2018, 8, 7179–7189 CrossRef CAS;
(e) N. Sauermann, T. H. Meyer, Y. Qiu and L. Ackermann, ACS Catal., 2018, 8, 7086–7103 CrossRef CAS.
-
(a) K. R. Wee, W. S. Han, D. W. Cho, S. Kwon, C. Pac and S. O. Kang, Angew. Chem., Int. Ed., 2012, 51, 2677–2680 CrossRef CAS;
(b) K. R. Wee, Y. J. Cho, S. Jeong, S. Kwon, J. D. Lee, I. H. Suh and S. O. Kang, J. Am. Chem. Soc., 2012, 134, 17982–17990 CrossRef CAS PubMed.
- For detailed information, see the ESI.†.
- Deposition numbers 2049567 (3aa), 2049566 (3am), 2049564 (3ao), 2065339 (4br), 2049565 (4av) and 2063919 (5b) contain the supplementary crystallographic data for this paper..
|
This journal is © The Royal Society of Chemistry 2021 |