DOI:
10.1039/D1SC02662C
(Edge Article)
Chem. Sci., 2021,
12, 10455-10466
Experimental evidence that halogen bonding catalyzes the heterogeneous chlorination of alkenes in submicron liquid droplets†
Received
14th May 2021
, Accepted 9th July 2021
First published on 9th July 2021
Abstract
A key challenge in predicting the multiphase chemistry of aerosols and droplets is connecting reaction probabilities, observed in an experiment, with the kinetics of individual elementary steps that control the chemistry that occurs across a gas/liquid interface. Here we report evidence that oxygenated molecules accelerate the heterogeneous reaction rate of chlorine gas with an alkene (squalene, Sqe) in submicron droplets. The effective reaction probability for Sqe is sensitive to both the aerosol composition and gas phase environment. In binary aerosol mixtures with 2-decyl-1-tetradecanol, linoleic acid and oleic acid, Sqe reacts 12–23× more rapidly than in a pure aerosol. In contrast, the reactivity of Sqe is diminished by 3× when mixed with an alkane. Additionally, small oxygenated molecules in the gas phase (water, ethanol, acetone, and acetic acid) accelerate (up to 10×) the heterogeneous chlorination rate of Sqe. The overall reaction mechanism is not altered by the presence of these aerosol and gas phase additives, suggesting instead that they act as catalysts. Since the largest rate acceleration occurs in the presence of oxygenated molecules, we conclude that halogen bonding enhances reactivity by slowing the desorption kinetics of Cl2 at the interface, in a way that is analogous to decreasing temperature. These results highlight the importance of relatively weak interactions in controlling the speed of multiphase reactions important for atmospheric and indoor environments.
Introduction
The reaction rate of a gas phase molecule with an aerosol or droplet surface is governed by a set of kinetic steps that include adsorption/desorption, solvation/de-solvation, and diffusion.1–5 The relative rates and coupling of these steps can often yield new pathways or catalytic mechanisms,6–13 which are largely absent for a reaction occurring solely within a single phase. Given the central role that aerosols play in air pollution,14,15 climate16–18 and human health,19 developing better insight, using laboratory measurements,20 into the molecular factors that enhance, suppress or even catalyze multiphase chemical reactions is an important challenge. Using a model reaction, the heterogeneous chlorination of an alkene, we examine how “spectator molecules” in the gas and condensed phase decelerate as well as catalyze heterogeneous transformations. These results suggest that weak interactions, such as halogen bonding, can play substantial roles in controlling the speed at which multiphase transformations occur in the atmosphere and on indoor surfaces.21–25
Rudich and co-workers6 observed that classes of chemical reactions that occur slowly in the gas phase (k < 10−15 cm3 molecules−1 s−1), due to high activation barriers, are enhanced at monolayer organic surfaces that mimic tropospheric aerosol; in some cases by many orders of magnitude. For example, the reaction of bromine atoms with saturated aliphatic films occurs 10
000 times faster than predictions based upon analogous reactions in the gas phase. Conversely, gas phase reactions that are already fast6 (k ∼10−12 cm3 molecules−1 s−1), exhibit only minimal surface enhancement.
Some fraction26 of the enhanced reactivity at surfaces vs. the gas phase can be explained, somewhat trivially, by secondary reactions (i.e. promoted by the higher molecular densities at surfaces) or by the asymmetry of interfaces, where unreactive forward scattering trajectories yield additional possibilities for a reaction while in the gas phase they do not. More interestingly, surface enhancement factors are also consistent with well-known changes in the activation energy for an interfacial reaction, which are controlled by an array of interactions, such as molecular orientation, partial solvation, and the formation of unique interfacial complexes.27 Theoretical work has also shown the importance of relatively weak interactions, such as hydrogen bonding, in governing reaction mechanisms at the surface of atmospheric droplets.8–10 A recent study11 revealed the importance of halogen bonded complexes in mediating iodine reactions at droplet surfaces. In most cases, a halogen bond is weaker than a hydrogen-bond with typical bond lengths ∼2.8 Å, which form between an electrophilic molecule containing a halogen atom X (X = I, Br, Cl and F), and a nucleophilic molecule containing atoms, such as N, O, S etc.28–32
The reaction of Cl2 with an alkene is relatively slow, so it is an ideal system to examine how the competing kinetics of chlorine adsorption/desorption, solvation/desolvation influence heterogeneous reaction rates. Here we examine the heterogeneous reaction of Cl2 with squalene (Sqe) – a branched hydrocarbon with six C
C bonds. This reaction has been previously well-characterized by Popolan et al.33 These prior results are leveraged to examine how the heterogeneous reaction rate depends upon temperature and the presence of small spectator molecules in the gas, which are unreactive towards Cl2 (water, ethanol, acetone, and acetic acid). We also examine how doping a fatty acid, an ester, alcohol, or an alkane together with squalene in the aerosol phase alters its reactivity towards Cl2. The chemistry examined in these model systems addresses the growing interest in understanding surface reaction mechanisms of unsaturated fatty acids and Sqe; major constituents of skin lipids34 that are reactive towards halogens commonly released during indoor activities, e.g. bleach cleaning ([Cl2] ∼ 100 ppb).25,35–39
This paper is organized as follows. The apparatus and experimental approach for quantifying heterogeneous chlorination rates, reaction products as well as determining effective uptake coefficients are described in Experimental design. Data is presented in Results and discussion aimed at understanding the changes in Sqe reactivity and product formation kinetics as a function of temperature and in binary mixtures of Sqe with 2-decyl-1-tetradecanol, oleic and linoleic acids, bis(2-ethylhexyl) sebacate and squalane. Additional results are presented examining how the reaction rate of Sqe is altered by the presence of small gas phase molecules such as H2O, acetone, acetic acid, and ethanol. Together these experiments point to the interactions of Cl2 with alkene (C
C), alcohol (–OH), acid (–COOH), and ester (–COOC) functional groups, which, like temperature, appear to modify the desorption kinetics of Cl2 from the aerosol surface.
Experimental design
The heterogeneous reactions are conducted in the flowtube reactor, which has been widely used to explore the chemical kinetics of the heterogeneous reactions between aerosols and gases. Below we summarize the main features of the experiment.
Aerosol generation
Polydisperse aerosols are generated via homogeneous nucleation by passing dry N2 (0.3 SLM) through a Pyrex tube located in a tube furnace. The Pyrex tube is filled with either a neat liquid (e.g. Sqe), or is mixed with an additive, i.e. linoleic acid (LA), oleic acid (OA), 2-decyl-1-tetradecanol (C24OH), squalane (Sqa) or bis(2-ethylhexyl) sebacate (BES). These particular additives were selected because they are liquids at room temperature and should be fully miscible in Sqe. Specifically, 2-decyl-1-tetradecanol, also a liquid at room temperature, is used rather than a normal alcohol to avoid the potential complications of well-known surface freezing effects, which can produce unexpected reaction products as observed for the OH oxidation of n-octacosane.40 The final composition of the aerosol is assumed to identical to the composition of the liquid mixture prior to nucleating the aerosol. These chemicals were purchased from Sigma-Aldrich. As the vapor cools and exits the Pyrex tube, aerosols homogeneously nucleate. The aerosol-laden flow is then passed through an annular activated charcoal denuder to remove any residual gas-phase organics produced in the oven. The furnace temperature is adjusted for each experiment to generate stable aerosol streams. The average aerosol distribution is log-normal with a mass concentration of ∼3000 μg m−3 (2.0 × 106 cm−3) and an average diameter of 150 nm.
Flowtube reactor
Upon exiting from the charcoal denuder, the aerosol flow is mixed with additional gases: Cl2, O2, wet or dry N2 and for selected experiments gas phase additives (acetone, acetic acid, ethanol), to yield a total flow of 1.1 SLM. This flow is then introduced into the room temperature flowtube reactor (140 cm long and 2.5 cm inner diameter) that has, under these flow conditions, an average residence time of ∼37 seconds. For the temperature dependent experiments, a second double-walled flowtube reactor is used. This flow tube is 110 cm long with an inner diameter of 2.2 cm and an average residence time, at 1.1 SLM, of ∼23 seconds. Water is circulated in the outer portion of the flow tube in order to control reaction temperature. The recirculated water is fixed at 5, 25, 35 or 50 °C for the temperature dependent studies. Cl2 is delivered from a standard cylinder (52 ppm balanced with nitrogen) purchased from Praxair, Inc. All experiments are conducted at atmospheric pressure and in the dark to avoid the possible photolysis of Cl2 to produce Cl atoms.
Detectors
The aerosol chemical composition and its size distribution are measured in real time. The aerosol size distribution is measured by a TSI scanning mobility particle sizer (SMPS), consisting of a 3080L Differential Mobility Analyzer (DMA) and 3025 Condensation Particle Counter (CPC). The average size of the aerosol remains unchanged during reaction, which indicates that C–C bond scission pathways to produce volatile reaction products are unlikely.
The chemical composition of the aerosol is measured by a vacuum ultraviolet aerosol mass spectrometer (VUV-AMS),41,42 located at the Chemical Dynamics Beamline 9.0.2, Advanced Light Source (ALS), Lawrence Berkeley National Laboratory, Berkeley, CA, USA. Mass spectra are recorded by first vaporizing the aerosol at ∼125 °C, followed by photoionization using tunable vacuum ultraviolet (VUV) light. Photon energies are selected for each experiment to minimize ion fragmentation. 9.6 eV is used for neat Sqe, while slightly larger energies are used for Sqe/additive mixtures (e.g. 10.2 eV for Sqe/C24OH mixture experiment).
Data analysis
In a typical experiment, a series of mass spectra are recorded as a function of Cl2 exposure ([Cl2] × reaction time) to obtain the kinetic decay of Sqe and the rise of reaction products, as shown in Fig. 1A. A plot of the normalized Sqe signal versus Cl2 exposure is then fit to an exponential function to obtain a second order heterogeneous rate coefficient, kfit.43kfit is then used to compute an effective reaction probability for Sqe (γSqeeff) using, | 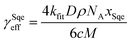 | (1) |
where D, ρ, c, M and NA are the average diameter, the initial particle density, velocity of Cl2 gas, molecular weight of Sqe, and Avogadro's number, respectively. γSqeeff is the fraction of Cl2 collisions with Sqe in the aerosol that yield a reaction.43 For the mixture experiments, the mole fraction of the Sqe (xSqe) is used when computing γSqeeff. This approach allows us to focus on how the inherent reaction rate (i.e. γSqeeff) of Sqe with Cl2 is altered in the presence of spectator molecules doped either together with Sqe in the aerosol phase or introduced as gases into the reactor.
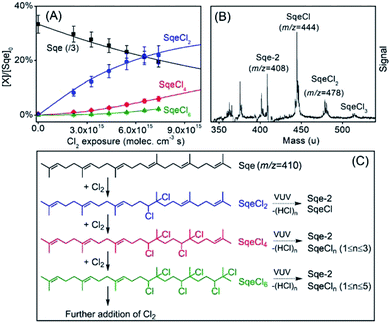 |
| Fig. 1 (A) Normalized concentrations of Sqe and SqeCln as a function of chlorine exposure ([Cl2] × time). The normalized concentration of Sqe is divided by three (i.e. /3) for ease of presentation. Symbols are experiments. Solid lines are predictions by the sequential reaction model described in eqn (2). (B) Difference mass spectra (reacted–unreacted Sqe) showing the SqeCln reaction products. (C) A reaction scheme showing the first three product generations (n), as well as the dissociative photoionization pathways that involve one or more –HCl eliminations. | |
A sequence of chlorinated reaction products (denoted SqeCln) are observed in the mass spectra and provide insight into the reaction mechanism shown in Fig. 1. It is difficult to obtain the absolute concentrations of the reaction products from the ion signal alone, due to the lack of absolute photoionization cross-sections and authentic standards to account for ion fragmentation patterns. Instead, the relative abundance (%) of the products are reported by normalizing the product signals (e.g. product [SqeCln]) to the initial signal of the unreacted Sqe (e.g. [Sqe]0), namely [SqeCln]/[Sqe]0.
Results and discussion
(a) Kinetics and temperature dependence of the heterogeneous reaction of neat Sqe
Fig. 1A shows the normalized decay of Sqe as a function of Cl2 exposure, which when fit to an exponential function yields γSqeeff = 3.5 × 10−5 using eqn (1). This value is slightly smaller than previously reported value by Popolan et al.33 (i.e. 6.6 × 10−5). The reason for this difference is unclear, but likely reflects, as will be shown below, the sensitivity of this reaction to the presence of gas and particle phase species present in the reactor. Fig. 1B shows a difference mass spectrum (reacted–unreacted) showing reaction products; denoted SqeCln. This pattern of reaction products is consistent with the sequential electrophilic addition of Cl2 to each of the 6 C
C bonds (Fig. 1C), after accounting for –(HCl)n elimination produced by dissociative photoionization as shown previously.33,44 For example, the first-generation neutral product SqeCl2 (C30H50Cl2) appears at a m/z corresponding to either SqeCl (C30H49Cl) or Sqe-2 (C30H48) after one or two –HCl eliminations, respectively. This pattern is also consistent with –(HI)n elimination observed during dissociative photoionization of iodo-hydrocarbons.45
The kinetic evolution of each product generation, n (SqeCln), shown in Fig. 1A appears statistical and is consistent with a sequential reaction model analysis46 conducted by Popolan et al.33 as well as other systems exhibiting multi-generational kinetics.43–45 The sequential reaction model applies to particles that are well-mixed on the timescale of the reaction, where the population of reactive species in the aerosol (i.e. Sqe, SqeCln) is a Poisson distribution46 (eqn (2)) that evolves with chlorine exposure ([Cl2]t).
|  | (2) |
[SqeCl
2n] is the concentration of product generation (
n) and [Sqe]
0 is the initial Sqe concentration.
kfit is the second order heterogeneous rate constant, which is assumed, for simplicity, to be the same for all generations (
e.g. Cl
2 + Sqe and Cl
2 + SqeCl
n).
Eqn (2) can reasonably account for the kinetic evolution of the products (SqeCl
2, SqeCl
4 and SqeCl
6) as shown in
Fig. 1A, which indicates that the chemical evolution of Sqe and SqeCl
n can be well represented by the same rate constant
kfit, indicating that

.
The temperature dependence of the Sqe + Cl2 reaction is measured at 5, 25 and 50 °C. As shown in Fig. 2A, the Sqe reaction rate gets faster as the temperature is lowered (e.g. 50 to 5 °C). As expected, γSqeeff also increases with decreasing temperature as shown in Fig. 2B, with the reaction proceeding ∼5× faster at 5 °C compared to 50 °C. Although the reaction rate increases with decreasing temperature, the product formation kinetics remain sequential (i.e.
) and the product mass spectra (Fig. 1B) are nearly identical for all temperatures. This suggests that the overall mechanism does not change with temperature, but rather just the overall rate of chlorination.
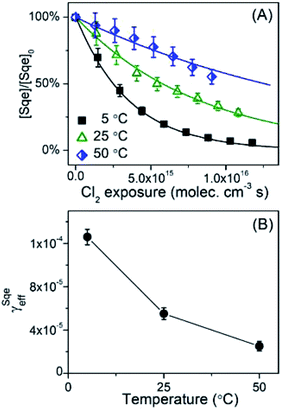 |
| Fig. 2 (A) Normalized decay of Sqe as a function of Cl2 exposure at 5, 25 and 50 °C. Symbols are experimental data; solid lines are predictions from the sequential reaction model. (B) γSqeeff as a function of temperature. Symbols are experimental data, which are connected by solid lines to guide the eye. | |
While this negative temperature dependence is somewhat unusual for chemical reactions occurring in single phase, it does occur for multistep reactions that proceed through a potential well or pre-reactive complex. Trace gas uptake at droplet surfaces often exhibit a negative temperature dependence, since mass accommodation is controlled by multiple elementary steps as shown in Fig. 3.1–5,47–50 Without reaction, there are two coupled equilibria that control the concentration of Cl2 in the aerosol. The first equilibrium describes the partitioning of gas phase Cl2(gas) to form an adsorbed species (i.e. Cl2(ads)), whose steady state concentration is controlled by the rate constants for Cl2 adsorption (kads) to and desorption (kdes) from the interface (steps (i) and (ii) in Fig. 3). The second equilibrium describes the partitioning of Cl2(ads) to the bulk (Cl2(bulk)) and is governed by the solvation (ksol) and de-solvation (ksol-rev) rate constants shown in steps (iii) and (iv) in Fig. 3. The product of these two equilibria is the Henry's law constant (H) for Cl2. Diffusion pathways are not explicitly shown in Fig. 3.
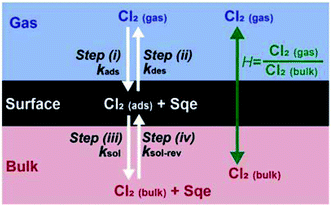 |
| Fig. 3 Schematic of gas uptake at an aerosol surface showing adsorption, desorption, solvation, de-solvation steps (denoted as steps (i) to (iv)), as well as the chemical reactions. The net Henry's law constant (H) is also shown. | |
For the system considered here there are reaction steps in the bulk and at the surface. The reacto-diffusive length (L) of Cl2 in Sqe is,
|  | (3) |
where
D is the diffusion coefficient of Cl
2 and
krxn is the bimolecular rate constant for the chlorination reaction.
L is estimated to be ∼300 nm (
D = 1.38 × 10
−5 cm
2 s
−1,
51krxn = 1.0 × 10
−17 cm
3 molecules
−1 s
−1,
52 [Sqe] = 1.26 × 10
21 molecules cm
−3), which is ∼2× larger than the average aerosol diameter used in the experiments. Additionally, Cl
2 is quite soluble in both hydrocarbons
53,54 (
e.g. decane
55) and in polymers
56,57 with a dimensionless Henry's law (H
cc) constant between 10–20. Thus chlorination is expected to occur within the volume of the particle. However, despite this expectation that chlorination occurs mainly in the bulk (as suggested by
eqn (3)), the temperature dependence of the uptake coefficient, estimated using a resistor model explicitly neglecting surface processes, predicts that the uptake coefficient will actually decrease with temperature as shown in Fig. S1 and described in the ESI.
† This is opposite of the experimental trend and suggests the importance of surface process in governing the observed uptake coefficient.
58
As shown previously for many systems,1–4,47–50 the negative temperature dependence observed for the mass accommodation coefficient arises from the aggregate temperature dependence of the individual surface steps shown in Fig. 3. The negative temperature dependence observed in Fig. 2B is likely of similar origin.4,47,48 From previous studies,59 the adsorption step kads is assumed to have no barrier and expected to be nearly temperature independent with only slight changes arising from the temperature dependence of the Cl2 velocity. The negative dependence48,60 is mainly controlled by surface desorption (kdes, step (ii), Fig. 3) and solvation (ksol, step (iii), Fig. 3), however the exact scaling and magnitude of these individual rates is model dependent. From molecular beam scattering experiments, kdes is expected to decrease with temperature following an Arrhenius relationship: as temperature decreases, desorption time lengthens and kdes becomes smaller.61 The temperature dependence of ksol is more ambiguous in prior studies. In some studies,59,60,62ksol is thought to be weakly temperature dependent, varying much more slowly than desorption due to the smaller activation energy for solvation. The critical cluster nucleation model60 predicts an increase of ksol with decreasing temperature, whereas the Gibb's surface model60 predicts the opposite – a slight decrease. In Molecular Dynamics (MD) simulations63–65 there doesn't appear to be a substantial barrier for solvation, as predicted by the nucleation model. To our knowledge, there remains a substantial uncertainty in how to reconcile these various model predictions (MD, critical cluster nucleation,49 capillary wave66) of trace gas uptake with experimental measurements,1,2,60,67,68 which is needed to produce a self-consistent set of kinetic parameters that vary with temperature.
Despite this uncertainty, the temperature dependence shown in Fig. 2B can be reasonably interpreted as follows. Since the reacto-diffusive length is larger than the particle diameter, the chlorination reaction is expected to occur throughout the particle volume. So as temperature decreases so does kdes, leading to an increase in [Cl2(ads)], which will increase both the chlorination rate at the interface as well as the rate of solvation (i.e. Cl2(ads) → Cl2(bulk)), which in turn increases the chlorination rate in the bulk. Effectively, a decrease in kdes (or ksol) at low temperatures, increases the net Henry's law constant (H) for Cl2, since it is product of the two equilibria shown in Fig. 3.
In order to further explore the origin of the accelerated heterogeneous reaction rates at low temperature, a series of isothermal experiments are conducted to examine how spectator molecules containing oxygenated functional groups modify the chlorination rate of Sqe in a similar way as decreasing the temperature.
(b) Accelerated rates in Sqe/alcohol mixtures
2-Decyl-1-tetradecanol (C24H50O, termed C24OH) is mixed with Sqe to make an aerosol of binary composition. C24OH is saturated and thus unreactive towards Cl2. As shown in Fig. 4A, the decay of Sqe in the binary mixture is faster when compared to the reactivity of neat Sqe. Fig. 4B shows γSqeeff as a function of C24OH mole fraction, which is observed to steeply increase and then plateau at 8 × 10−4 when the aerosol is ∼12–15% C24OH. γSqeeff in the mixture is 23 times larger than for the neat Sqe experiment (3.5 × 10−5). As discussed below we find no evidence that the presence of C24OH alters the product composition.
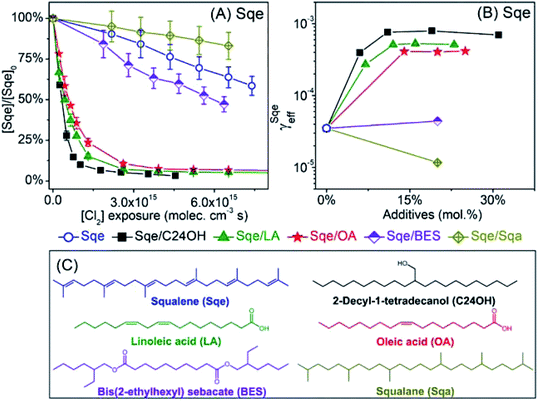 |
| Fig. 4 (A) Normalized decay of Sqe as a function of Cl2 exposure for neat Sqe and Sqe + additive (i.e. C24OH, LA, OA, BES and Sqa) experiments. (B) γSqeeff as a function of additive mole fraction. Symbols are experimental data, which are connected by solid lines to guide the eye. (C) Chemical structures of Sqe and the liquid additives. | |
The plateau shown in Fig. 4B suggests that C24OH partitions to the aerosol surface and tends towards saturation as its bulk mole fraction is increased. The steep increase and saturation of γSqeeff is different from the monotonic increase one would expect for the alternative case where the bulk solubility of Cl2 is substantially larger in C24OH than in Sqe. Previous computational and experimental studies, on reasonably analogous systems, support these observations; namely that oxygenated solutes (e.g. C24OH, ethanol, and other oxygenates) generally partition to the surface of a hydrocarbon solvent. For example, Pecsok and Gump69 found that the surface tension of squalane (saturated analog of Sqe) decreases steeply when mixed with a small amount of ethanol. At ethanol mole fractions of ∼0.02, the squalane/ethanol solution exhibits a surface tension that is nearly identical to that of the pure ethanol liquid. A Molecular Dynamics study of squalane, reported by Wick et al.,70 found that for 1-butanol the gas–surface partitioning coefficient was ∼1.5 × larger than the gas–bulk partitioning coefficient, indicating interfacial enrichment. Another study71 of the partitioning of alkanols in squalane found that surface partitioning increased with alcohol carbon number, with the surface partitioning coefficient of 4-heptanol and 2-heptanol being 10 to 100 times larger than that of ethanol, respectively.69
Fig. 5A shows the reaction products formed in the Sqe/C24OH mixture experiment. The major products are the same series of SqeCln peaks that were observed in the neat Sqe reaction (Fig. 1). This is expected since C24OH lacks reactive sites for electrophilic addition of Cl2. The kinetics, shown in Fig. 5B, although faster, remain sequential (i.e.
) in the presence of C24OH.
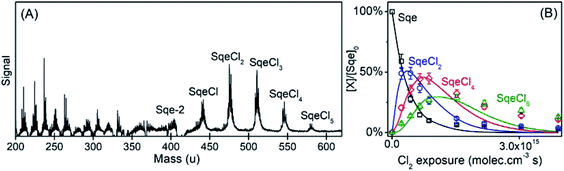 |
| Fig. 5 (A) Difference mass spectra (reacted–unreacted Sqe) showing the reaction products (SqeCln); (B) Decay of Sqe and the formation kinetics of SqeCln in the Sqe/C24OH mixture experiment. Symbols are experimental data; solid lines are generated from the sequential reaction model in eqn (2). | |
In summary, we find evidence for the surface partitioning of C24OH, but no evidence that its presence in the aerosol forms new reaction products, which would suggest an altered reaction mechanism (e.g. chlorohydrin formation39). Rather, the alcohol appears only to accelerate the sequential addition of chlorine to Sqe without directly participating in the mechanism (i.e. a catalyst). Together these observations suggest that the acceleration mechanism involves a slowing of the Cl2 desorption kinetics (in analogous way as decreasing temperature for the neat Sqe reaction) likely through the stronger binding of Cl2 to the –OH moiety of C24OH. This is consistent with the delayed desorption of gas when forming interfacial hydrogen-bonds, as reported by Morris and coworkers.61 Thus, any decrease in kdes, due to halogen-bonding, will enhance both the chlorination rate of Sqe at the interface as well as solvation rate of Cl2(ads), which together would account for the accelerated kinetics.
Fig. 6A shows the temperature dependence of the Sqe decay kinetics in a binary mixture with C24OH. Although the decay of Sqe in the mixture becomes more rapid as temperature is reduced to 5 °C, the temperature dependence of the uptake coefficient is much weaker in the mixture than the pure Sqe aerosol (Fig. 6B). An Arrhenius analysis of the temperature dependence in Fig. 6B yields a phenomenological activation energy of −26.7 kJ mol−1 for pure Sqe and −13.9 kJ mol−1 for the Sqe/C24OH mixture. In the past, the temperature dependence of the mass accommodation coefficient is used to extract information about the energetic barriers for non-reactive and in some cases reactive uptake. However, the interpretation of the energies extracted from the data in Fig. 6B in terms of simple energetic barriers is more ambiguous due to the complex coupling of the equilibria and surface/bulk reaction pathways shown in Fig. 3.
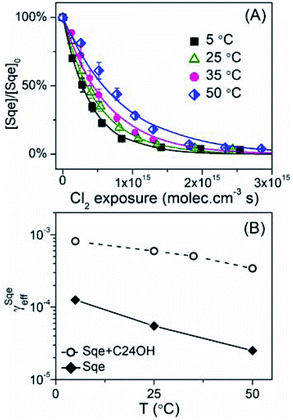 |
| Fig. 6 (A) Normalized decay of Sqe as a function of Cl2 exposure for Sqe/C24OH as a function of temperature (5, 25, 35 and 50 °C). Symbols are experimental data; solid lines are from the sequential reaction model. (B) γSqeeff as a function of temperature for pure Sqe (solid symbols), and the Sqe/C24OH mixture experiments (open symbols). Symbols are experimental data, which are connected by lines to guide the eye. | |
(c) Accelerated rates in Sqe/fatty acid mixtures
Aerosols containing binary mixtures of Sqe and two liquid fatty acids are used to further evaluate the role of oxygenated functional groups (i.e. –COOH) in accelerating the chlorination of squalene. Unlike C24OH, linoleic acid (LA) and oleic acid (OA) have C
C bond(s), and react with Cl2. LA and OA reside together with Sqe in skin lipids.34Fig. 4A shows the decay of Sqe in binary mixtures with either LA or OA. The presence of these fatty acids leads to faster decay kinetics for Sqe than for the neat case. As observed with C24OH, there is a steep increase of γSqeeff with LA and OA mole fraction, reaching a plateau of γSqeeff = 5.3 × 10−4 and 4.17 × 10−4, which is around 16 and 12 times faster than the reaction rate of pure Sqe.
Fig. 7 compares the products formed in the Sqe/LA (or OA) mixture with results obtained using pure LA (or OA). In addition to the series of products originating from Sqe (i.e. SqeCln), there appears new species that arise from the reaction of Cl2 with OA and LA. This is expected since the C
C bond(s) in LA and OA react with Cl2 to produce LACln (detected as LA-2 and LACl) and OACln (detected as OA-2) products as shown in Fig. 7. These LACln and OACln products are the same as those in pure aerosols of LA (or OA) as shown in Fig. 7A and C. The reaction pathways for the formation of these chlorinated products and the mass spectral peak patterns, after accounting for –(HCl)n elimination, are shown in Fig. S2 and S3 in the ESI.† These patterns are consistent with the dissociative photoionization pathways observed for SqeCln and described above.
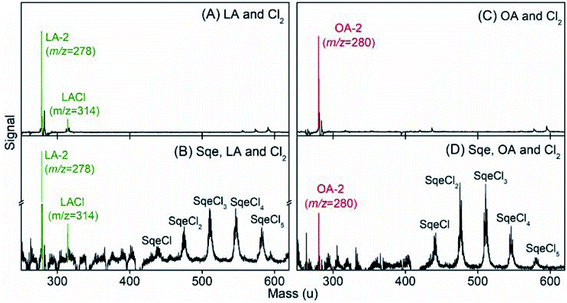 |
| Fig. 7 Difference mass spectra showing reaction products (SqeCln, LACln and OACln) during the heterogeneous reaction of Cl2 in (A) neat LA; (B) the Sqe/LA mixture; (C) neat OA; and (D) the Sqe/OA mixture experiments, respectively. | |
Besides SqeCln, LACln and OACln, no new products, beyond what is observed for pure LA and OA, are detected in the mixed aerosols, which suggest that the presence of these fatty acids does not alter the reaction pathway of Sqe itself. This is consistent with Sqe/C24OH experiment described above. Additional experimental evidence and checks for Sqe/LA system can be found in Fig. S4 and S5 in the ESI.†
It is interesting to note that the acceleration of the Sqe kinetics in the presence of LA (or OA) is only slightly smaller than that observed for C24OH as shown in Fig. 4B. This suggests that the key moiety that enhances the kinetics is the –OH rather than the –C
O group.
(d) Accelerated and decelerated heterogeneous rates of Sqe in the presence of BES and Sqa
To examine the role of the –C
O group on the rate acceleration, a liquid ester, bis(2-ethylhexyl) sebacate (BES), is mixed with Sqe as shown in Fig. 4C. BES has a saturated carbon backbone, which is unreactive towards Cl2. As shown in Fig. 4, the presence of BES slightly accelerates the decay of Sqe, with a γSqeeff that is only 26% larger than is observed for the pure Sqe case. This indicates, assuming that interfacial orientation plays a minor role, that the –C
O portion of BES only slightly increases the reaction rate, suggesting that the largest contribution to reaction acceleration is from the –OH group in the acids. For a final comparison, a saturated alkane, squalane (Sqa), is added to Sqe. Sqa has a similar branched structure as Sqe, but lacks C
C bonds as shown in Fig. 4C. In this binary mixture, γSqeeff decreases by 66% relative to pure Sqe.
As shown above for the Sqe/C24OH experiment, the same set of Sqe reaction products are observed to form sequentially in the LA, OA, BES and Sqa mixtures, albeit their formation kinetics are either accelerated or decelerated when compared to the pure Sqe aerosol. This is illustrated in Fig. 8A where the formation and subsequent consumption kinetics of SqeCl is observed to be most rapid for the Sqe/C24OH mixture, followed by Sqe/LA, Sqe/OA, Sqe/BES, pure Sqe and Sqe/Sqa, respectively. The product formation kinetics mirror the trend in γSqeeff observed in Fig. 4A. This is illustrated in Fig. 8B where the kinetics are recast in terms of Sqe lifetimes (τSqe) instead of Cl2 exposure as shown in Fig. 8A. τSqe is the product of Cl2 exposure (molecules per cm3 × s) and the inverse Sqe decay constant, 1/kfit. Fig. 8B shows that the evolution of SqeCl in pure Sqe aerosol and in the various binary mixtures as a function of τSqe are identical within error. This indicates that the presence of the aerosol additives doesn't change the overall reaction mechanism but rather these additives serve only to modulate the overall rate of sequential addition of Cl2 to Sqe and its chlorination products.
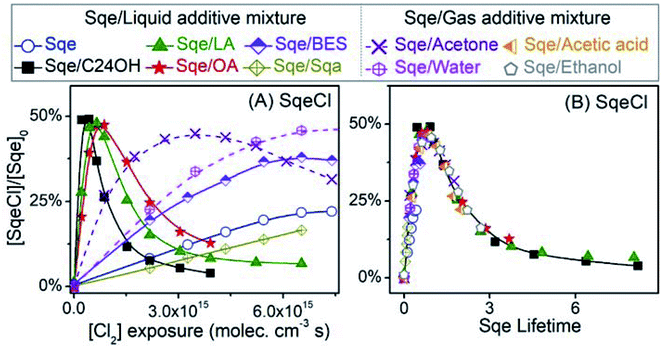 |
| Fig. 8 Formation kinetics of SqeCl in pure Sqe aerosols, Sqe/C24OH, Sqe/LA, Sqe/OA, Sqe/BES and Sqe/Sqa aerosol mixtures, and Sqe aerosols with gas additives (i.e. acetone, water, acetic acid, ethanol): (A) as a function of Cl2 exposure; or (B) as a function of Sqe lifetime (τSqe). Symbols are experimental data, which are connected by lines to guide the eye. | |
To summarize, the reactivity of Sqe can vary by over an order of magnitude and depends upon temperature and molecules co-present with Sqe in the aerosol. We find no evidence that these additives (or temperature) alter the Sqe + Cl2 mechanism through the formation of new products or by deviations from the sequential reaction mechanism. Instead, we find that the magnitude of γSqeeff scales with the molecular structure of the additive, with clear evidence that oxygenated functional groups accelerate the heterogeneous chlorination of Sqe. The observed order of decreasing heterogeneous reactivity by functional group is: –OH > –COOH > –C
O > C
C > C–C. This pattern appears consistent with the expected interaction strength of Cl2 with various functional groups, pointing to the importance of halogen bonding in promoting heterogeneous reactivity in the system. For example, from calculations it is observed that the interaction of Cl2 with –OH groups is more favorable than with C
C bonds.30 It seems likely that the additives (as well as reducing temperature) prolong the lifetime of Cl2 at the aerosol surface, allowing increased flux through those pathways that culminate in a reaction, either directly at the interface or via reaction in the bulk after solvation of Cl2(ads). This conclusion will be further tested by introducing small oxygenated spectator molecules, as additives to the gas phase.
(e) Heterogeneous chlorination of Sqe with gas phase additives
Gas phase additives are introduced to the reactor by passing N2 through a glass bubbler filled with either water, ethanol, acetic acid, or acetone. The vapor concentration is controlled by changing the fraction of the total N2 flow that is diverted through the bubbler. Fig. 9A shows that Sqe decays faster with increasing water vapor concentration ([H2O]). γSqeeff increases monotonically with [H2O], reaching a value at [H2O] = 4.2 × 1017 moleclues cm−3, that is 45% faster than under dry conditions (Fig. 9C). These observations are consistent with a recent study that has confirmed the presence of adsorbed water at the squalene/air interface.72
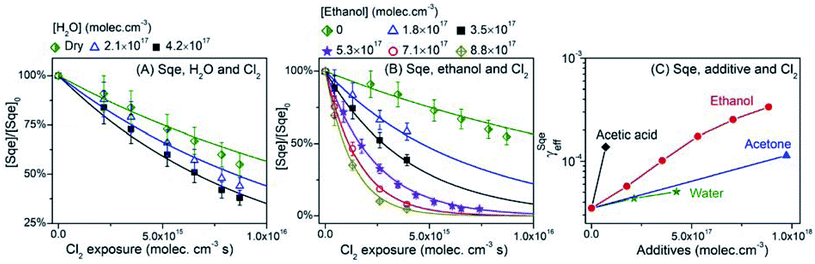 |
| Fig. 9 Normalized decay of Sqe as a function of Cl2 exposure and gas phase additives concentration: (A) H2O and (B) ethanol. (C) γSqeeff as a function of gas phase additive concentration. | |
Sqe decays more rapidly (up to 10×) with increasing concentrations of ethanol vapor as shown in Fig. 9B. This is also observed for acetic acid and acetone vapor. These results are summarized in Fig. 9C where γSqeeff shows a monotonic increase with additive concentration over what is observed for Sqe in the presence of N2 only. In each case γSqeeff does not reach a plateau as was observed for the longer chain alcohol and fatty acid aerosol mixtures shown in Fig. 4B. This likely means, for example, that ethanol does not reach high enough concentrations in our reactor to saturate the aerosol surface. The order of reaction acceleration normalized to gas phase concentration is: acetic acid > ethanol > acetone ≥ water. Direct comparison with the aerosol additive trend in Fig. 4 is somewhat difficult, since the magnitude of reaction acceleration will depend both upon the strength of halogen bonding to Cl2 as well as the partitioning of these small molecules to the aerosol surface, which will roughly scale with their relative vapor pressure.
The first generation reaction product (i.e. SqeCl) produced in the presence of these small gas phase molecules is plotted versus chlorine exposure and Sqe lifetime in Fig. 8. When normalized to Sqe lifetime the product formation kinetics are nearly identical to the binary mixture results. This suggests that in addition to aerosol phase additives, small gas phase molecules co-adsorbed to the aerosol surface can prolong the lifetime of Cl2 providing additional time for the chlorination reaction to occur. These results appear consistent with previous studies of the enhanced uptake of HCl gas onto organic liquids in the presence of co-adsorbed H2O, which is facilitated by the formation of H2O–HCl complexes as reported in the ref. 73.
Conclusions
Experimental evidence is presented that both gas and aerosol phase molecules, which have oxygenated functional groups, catalyze the heterogeneous chlorination of an alkene leading to, in some cases, a 20× enhancement in the reaction rate. This behavior is similar to reducing temperature, which is known to slow the desorption kinetics relative to competing elementary steps that ultimately lead to solvation and reaction. For certain additives (ester and alkane), the heterogeneous reaction rate of Cl2 with Sqe remains unchanged or is even slightly decelerated, which suggest weaker halogen bonding and larger values for kdes for these systems. We find no evidence that either the gas or aerosol phase additives modify the reaction mechanism.
Instead, these additives behave as spectators that either accelerate or decelerate the sequential formation of chlorinated Sqe reaction products. Together these results all point to the significant role that halogen bonding might play in this heterogeneous reaction. We propose that the formation of these relatively weak directional interactions between the oxygenated functional groups and Cl2 slows its desorption rate (i.e. a smaller kdes) from the aerosol surface, thereby enhancing those pathways (solvation and reaction) that increase the rate of electrophilic addition. A full kinetic analysis of this data set will be conducted in a forthcoming publication to examine quantitatively the coupling of reaction, adsorption, solvation, de-solvation, and reaction rates on the observed reactive uptake kinetics.
Finally, it is likely, and has indeed been shown theoretically that weak interactions, such as halogen and hydrogen bonding at aerosol surfaces can play a substantial role in determining heterogeneous reaction rates and mechanisms.8–11 The chlorination reaction studied here is perhaps unique in that it is relatively slow reaction compared to others (e.g. ozonolysis and free radical reactions), thereby allowing the coupling and competition of the elementary steps shown in Fig. 3 to be more easily observed.
It is also interesting to consider the implication of these results for indoor surface chemistry.23,24,38 Sqe, LA and OA are all important constituents of skin lipids, which act as a natural defense against the reactive oxygen and chlorine species. The half-life (τ1/2) of Sqe (in the absence of additives) is ∼56 min under indoor conditions when bleach is used ([Cl2] ∼ 100 ppb).35,37 However, in a mixture with other skin lipids such as LA (or OA), τ1/2 for Sqe decreases substantially to ∼3.6 min at the same [Cl2]. This indicates that skin lipid reactivity is a more complex function of the surface and gas phase environment, in which trace constituents and weak interactions play more important roles that previously imagined.
Data availability
The authors confirm that the data supporting the findings of this work are available within the article and its ESI.
Author contributions
M. Z. and K. R. W. designed the experiment. M. Z. made the experimental measurements and conducted the analysis of the data. M. Z. and K. R. W. wrote the manuscript.
Conflicts of interest
The authors declare no competing financial interest.
Acknowledgements
This work is supported by the Gas Phase Chemical Physics Program in the Chemical Sciences Geosciences and Biosciences Division of the Office of Basic Energy Sciences of the U.S. Department of Energy under Contract No. DE-AC02-05CH11231. This research used the Advanced Light Source, which is a U.S. Department of Energy Scientific User Facility under contract no. DE-AC02-05CH11231. The authors thank Bruce Rude and Ryan Reynolds for assistance at the ALS, Musahid Ahmed and Wenchao Lu for helpful discussion.
References
- P. Davidovits, C. E. Kolb, L. R. Williams, J. T. Jayne and D. R. Worsnop, Mass accommodation and chemical reactions at gas-liquid interfaces, Chem. Rev., 2006, 106, 1323–1354 CrossRef CAS PubMed.
- P. Davidovits, C. E. Kolb, L. R. Williams, J. T. Jayne and D. R. Worsnop, Update 1 of: Mass accommodation and chemical reactions at gas-liquid interfaces, Chem. Rev., 2011, 111, PR76–PR109 CrossRef CAS PubMed.
- G. M. Nathanson, Molecular beam studies of gas-liquid interfaces, Annu. Rev. Phys. Chem., 2004, 55, 231–255 CrossRef CAS PubMed.
- G. M. Nathanson, P. Davidovits, D. R. Worsnop and C. E. Kolb, Dynamics and kinetics at the gas-liquid interface, J. Phys. Chem., 1996, 100, 13007–13020 CrossRef CAS.
- M. Galib and D. T. Limmer, Reactive uptake of N2O5 by atmospheric aerosol is dominated by interfacial processes, Science, 2021, 371, 921–925 CrossRef CAS PubMed.
- T. Moise and Y. Rudich, Uptake of Cl and Br by organic surfaces – a perspective on organic aerosols processing by tropospheric oxidants, Geophys. Res. Lett., 2001, 28, 4083–4086 CrossRef CAS.
- G. Rovelli, M. I. Jacobs, M. D. Willis, R. J. Rapf, A. M. Prophet and K. R. Wilson, A critical analysis of electrospray techniques for the determination of accelerated rates and mechanisms of chemical reactions in droplets, Chem. Sci., 2020, 11, 13026–13043 RSC.
- R. D. Hoehn, M. A. Carignano, S. Kais, C. Zhu, J. Zhong, X. C. Zeng, J. S. Francisco and I. Gladich, Hydrogen bonding and orientation effects on the accommodation of methylamine at the air-water interface, J. Chem. Phys., 2016, 144, 214701 CrossRef PubMed.
- M. Kumar and J. S. Francisco, Reactions of Criegee Intermediates with Non-Water Greenhouse Gases: Implications for Metal Free Chemical Fixation of Carbon Dioxide, J. Phys. Chem. Lett., 2017, 8, 4206–4213 CrossRef CAS PubMed.
- M. Kumar, J. Zhong, X. C. Zeng and J. S. Francisco, Reaction of Criegee intermediate with nitric acid at the air-water interface, J. Am. Chem. Soc., 2018, 140, 4913–4921 CrossRef CAS PubMed.
- M. Kumar, T. Trabelsi, J. C. Gomez Martin, A. Saiz-Lopez and J. S. Francisco, HIOx-IONO2 dynamics at the air-water interface: revealing the existence of a halogen bond at the atmospheric aerosol surface, J. Am. Chem. Soc., 2020, 142, 12467–12477 CrossRef CAS PubMed.
- E. M. Knipping, M. J. Lakin, K. L. Foster, P. Jungwirth, D. J. Tobias, R. B. Gerber, D. Dabdub and B. J. Finlayson-Pitts, Experiments and simulations of ion-enhanced interfacial chemistry on aqueous NaCl aerosols, Science, 2000, 288, 301–306 CrossRef CAS.
- M. Jang, N. M. Czoschke, S. Lee and R. M. Kamens, Heterogeneous atmospheric aerosol production by acid-catalyzed particle-phase reactions, Science, 2002, 298, 814–817 CrossRef CAS PubMed.
- B. J. Finlayson-Pitts and J. N. Pitts Jr, Tropospheric air pollution: ozone, airborne toxics, polycyclic aromatic hydrocarbons, and particles, Science, 1997, 276, 1045–1051 CrossRef CAS PubMed.
- A. Mellouki, T. J. Wallington and J. Chen, Atmospheric chemistry of oxygenated volatile organic compounds: impacts on air quality and climate, Chem. Rev., 2015, 115, 3984–4014 CrossRef CAS.
- R. J. Charlson, S. E. Schwartz, J. M. Hales, R. D. Cess, J. J. A. Coakley, J. E. Hansen and D. J. Hofmann, Climate forcing by anthropogenic aerosols, Science, 1992, 255, 423–430 CrossRef CAS PubMed.
- V. Ramanathan, P. J. Crutzen, J. T. Kiehl and D. Rosenfeld, Aerosols, climate, and the hydrological cycle, Science, 2001, 294, 2119–2124 CrossRef CAS PubMed.
- M. Kanakidou, J. H. Seinfeld, S. N. Pandis, I. Barnes, F. J. Dentener, M. C. Facchini, R. V. Dingenen, B. Ervens, A. Nenes, C. J. Nielsen, E. Swietlicki, J. P. Putaud, Y. Balkanski, S. Fuzzi, J. Horth, G. K. Moortgat, R. Winterhalter, C. E. L. Myhre, K. Tsigaridis, E. Vignati, E. G. Stephanou and J. Wilson, Organic aerosol and global climate modelling: a review, Atmos. Chem. Phys., 2005, 5, 1053–1123 CrossRef CAS.
- U. Poschl, Atmospheric aerosols: composition, transformation, climate and health effects, Angew. Chem., Int. Ed., 2005, 44, 7520–7540 CrossRef PubMed.
- J. B. Burkholder, J. P. D. Abbatt, I. Barnes, J. M. Roberts, M. L. Melamed, M. Ammann, A. K. Bertram, C. D. Cappa, A. G. Carlton, L. J. Carpenter, J. N. Crowley, Y. Dubowski, C. George, D. E. Heard, H. Herrmann, F. N. Keutsch, J. H. Kroll, V. F. McNeill, N. L. Ng, S. A. Nizkorodov, J. J. Orlando, C. J. Percival, B. Picquet-Varrault, Y. Rudich, P. W. Seakins, J. D. Surratt, H. Tanimoto, J. A. Thornton, Z. Tong, G. S. Tyndall, A. Wahner, C. J. Weschler, K. R. Wilson and P. J. Ziemann, The Essential Role for Laboratory Studies in Atmospheric Chemistry, Environ. Sci. Technol., 2017, 51, 2519–2528 CrossRef CAS.
- Y. Liu, A. G. Bé, V. W. Or, M. R. Alves, V. H. Grassian and F. M. Geiger, Challenges and Opportunities in Molecular-Level Indoor Surface Chemistry and Physics, Cell Rep. Phys. Sci., 2020, 1, 100256 CrossRef.
- C. J. Weschler and N. Carslaw, Indoor Chemistry, Environ. Sci. Technol., 2018, 52, 2419–2428 CrossRef CAS PubMed.
- J. P. D. Abbatt and C. Wang, The atmospheric chemistry of indoor environments, Environ. Sci.: Processes Impacts, 2020, 22, 25–48 RSC.
- A. P. Ault, V. H. Grassian, N. Carslaw, D. B. Collins, H. Destaillats, D. J. Donaldson, D. K. Farmer, J. L. Jimenez, V. F. McNeill, G. C. Morrison, R. E. O'Brien, M. Shiraiwa, M. E. Vance, J. R. Wells and W. Xiong, Indoor Surface Chemistry: Developing a Molecular Picture of Reactions on Indoor Interfaces, Chem, 2020, 6, 3203–3218 CAS.
- S. Gligorovski and J. P. D. Abbatt, An indoor chemical cocktail, Science, 2018, 359, 632–633 CrossRef CAS PubMed.
- P. A. J. Bagot, C. Waring, M. L. Costen and K. G. McKendrick, Dynamics of Inelastic Scattering of OH Radicals from Reactive and Inert Liquid Surfaces, J. Phys. Chem. C, 2008, 112, 10868–10877 CrossRef CAS.
- M. F. Ruiz-Lopez, J. S. Francisco, M. T. C. Martins-Costa and J. M. Anglada, Molecular reactions at aqueous interfaces, Nat. Rev. Chem., 2020, 4, 459–475 CrossRef CAS.
- P. Metrangolo and G. Resnati, Halogen Versus Hydrogen, Science, 2008, 321, 918–919 CrossRef CAS.
- G. Cavallo, P. Metrangolo, R. Milani, T. Pilati, A. Priimagi, G. Resnati and G. Terraneo, The Halogen Bond, Chem. Rev., 2016, 116, 2478–2601 CrossRef CAS PubMed.
- S. J. Ang, A. M. Mak and M. W. Wong, Nature of halogen bonding involving pi-systems, nitroxide radicals and carbenes: a highlight of the importance of charge transfer, Phys. Chem. Chem. Phys., 2018, 20, 26463–26478 RSC.
- M. E. Wolf, B. Zhang, J. M. Turney and H. F. Schaefer, A comparison between hydrogen and halogen bonding: the hypohalous acid–water dimers, HOX⋯H2O (X = F, Cl, Br), Phys. Chem. Chem. Phys., 2019, 21, 6160–6170 RSC.
- P. Auffinger, F. A. Hays, E. Westhof and P. S. Ho, Halogen bonds in biological molecules, Proc. Natl. Acad. Sci. U. S. A., 2004, 101, 16789–16794 CrossRef CAS PubMed.
- D. M. Popolan-Vaida, C.-L. Liu, T. Nah, K. R. Wilson and S. R. Leone, Reaction of chlorine molecules with unsaturated submicron organic particles, Z. Phys. Chem., 2015, 229, 1521–1540 CrossRef CAS.
- N. Nicolaides, Skin Lipids: Their Biochemical Uniqueness, Science, 1974, 186, 19–26 CrossRef CAS PubMed.
- C. Wang, D. B. Collins and J. P. D. Abbatt, Indoor illumination of terpenes and bleach emissions leads to particle formation and growth, Environ. Sci. Technol., 2019, 53, 11792–11800 CrossRef CAS PubMed.
- J. P. S. Wong, N. Carslaw, R. Zhao, S. Zhou and J. P. D. Abbatt, Observations and impacts of bleach washing on indoor chlorine chemistry, Indoor Air, 2017, 27, 1082–1090 CrossRef CAS.
- J. M. Mattila, C. Arata, C. Wang, E. F. Katz, A. Abeleira, Y. Zhou, S. Zhou, A. H. Goldstein, J. P. D. Abbatt, P. F. DeCarlo and D. K. Farmer, Dark Chemistry during Bleach Cleaning Enhances Oxidation of Organics and Secondary Organic Aerosol Production Indoors, Environ. Sci. Technol. Lett., 2020, 7, 795–801 CrossRef CAS.
- J. M. Mattila, P. S. J. Lakey, M. Shiraiwa, C. Wang, J. P. D. Abbatt, C. Arata, A. H. Goldstein, L. Ampollini, E. F. Katz, P. F. DeCarlo, S. Zhou, T. F. Kahan, F. J. Cardoso-Saldana, L. H. Ruiz, A. Abeleira, E. K. Boedicker, M. E. Vance and D. K. Farmer, Multiphase chemistry controls inorganic chlorinated and nitrogenated compounds in indoor air during bleach cleaning, Environ. Sci. Technol., 2020, 54, 1730–1739 CrossRef CAS PubMed.
- H. Schwartz-Narbonne, C. Wang, S. Zhou, J. P. D. Abbatt and J. Faust, Heterogeneous Chlorination of Squalene and Oleic Acid, Environ. Sci. Technol., 2019, 53, 1217–1224 CrossRef CAS PubMed.
- C. R. Ruehl, T. Nah, G. Isaacman, D. R. Worton, A. W. H. Chan, K. R. Kolesar, C. D. Cappa, A. H. Goldstein and K. R. Wilson, The Influence of Molecular Structure and Aerosol Phase on the Heterogeneous Oxidation of Normal and Branched Alkanes by OH, J. Phys. Chem. A, 2013, 117, 3990–4000 CrossRef CAS.
- E. Gloaguen, E. R. Mysak, S. R. Leone, M. Ahmed and K. R. Wilson, Investigating the chemical composition of mixed organic–inorganic particles by “soft” vacuum ultraviolet photoionization: The reaction of ozone with anthracene on sodium chloride particles, Int. J. Mass Spectrom., 2006, 258, 74–85 CrossRef CAS.
- K. R. Wilson, M. Jimenez-Cruz, C. Nicolas, L. Belau, S. R. Leone and M. Ahmed, Thermal vaporization of biological nanoparticles: fragment-free vacuum ultraviolet photoionization mass spectra of tryptophan, phenylalanine-glycine-glycine, and β-carotene, J. Phys. Chem. A, 2006, 110, 2106–2113 CrossRef CAS PubMed.
- J. D. Smith, J. H. Kroll, C. D. Cappa, D. L. Che, C. L. Liu, M. Ahmed, S. R. Leone, D. R. Worsnop and K. R. Wilson, The heterogeneous reaction of hydroxyl radicals with sub-micron squalane particles: a model system for understanding the oxidative aging of ambient aerosols, Atmos. Chem. Phys., 2009, 9, 3209–3222 CrossRef CAS.
- C. L. Liu, J. D. Smith, D. L. Che, M. Ahmed, S. R. Leone and K. R. Wilson, The direct observation of secondary radical chain chemistry in the heterogeneous reaction of chlorine atoms with submicron squalane droplets, Phys. Chem. Chem. Phys., 2011, 13, 8993–9007 RSC.
- D. M. Popolan-Vaida, K. R. Wilson and S. R. Leone, Reaction of iodine atoms with submicrometer
squalane and squalene droplets: mechanistic insights into heterogeneous reactions, J. Phys. Chem. A, 2014, 118, 10688–10698 CrossRef CAS PubMed.
- K. R. Wilson, J. D. Smith, S. H. Kessler and J. H. Kroll, The statistical evolution of multiple generations of oxidation products in the photochemical aging of chemically reduced organic aerosol, Phys. Chem. Chem. Phys., 2012, 14, 1468–1479 RSC.
- D. R. Worsnop, M. S. Zahniser, C. E. Kolb, J. A. Gardner, L. R. Watson, J. M. Van Doren, J. T. Jayne and P. Davidovits, Temperature dependence of mass accommodation of SO2 and H2O2 on aqueous surfaces, J. Phys. Chem., 1989, 93, 1159–1172 CrossRef CAS.
- Q. Shi, P. Davidovits, J. T. Jayne, D. R. Worsnop and C. E. Kolb, Uptake of Gas-Phase Ammonia. 1. Uptake by Aqueous Surfaces as a Function of pH, J. Phys. Chem. A, 1999, 103, 8812–8823 CrossRef CAS.
- P. Davidovits, J. T. Jayne, S. X. Duan, D. R. Worsnop, M. S. Zahniser and C. E. Kolb, Uptake of gas molecules by liquids: a model, J. Phys. Chem., 1991, 95, 6337–6340 CrossRef CAS.
- P. Davidovits, J. H. Hu, D. R. Worsnop, M. S. Zahniser and C. E. Kolb, Entry of gas molecules into liquids, Faraday Discuss., 1995, 100, 65–82 RSC.
- A. Tang and O. C. Sandall, Diffusion Coefficient of Chlorine in Water at 25–60 °C, J. Chem. Eng. Data, 1985, 30, 189–191 CrossRef CAS.
- K. Yates and H. W. Leung, Kinetics and Stereochemistry of the Addition of Chlorine to Styrenes, J. Org. Chem., 1980, 45, 1401–1406 CrossRef CAS.
- N. W. Taylor and J. H. Hildebrand, Solubility. VIII. Solubility Relations of Certain Gases, J. Am. Chem. Soc., 1923, 45, 682–694 CrossRef CAS.
- M. Lohse and W. D. Deckwer, Solubility of chlorine in benzene, toluene, ethylbenzene, o-, m-, and p-xylenes, and 2-, 3-, and 4-chlorotoluenes, J. Chem. Eng. Data, 1981, 26, 159–161 CrossRef CAS.
-
IUPAC Solubility Data Series, ed. C. L. Young, Pergamon Press, Oxford, England, 1983, ch. Sulfur Dioxide, Chlorine, Fluorine and Chlorine Oxides, vol. 12, p. 494 Search PubMed.
- S. Wachi, H. Morikawa and H. Inoue, Conversion distribution in diffusion-governed chlorination of poly(vinyl chloride), AIChE J., 1988, 34, 1683–1690 CrossRef CAS.
- R. Paterson, Y. Yampol’skii, P. G. T. Fogg, A. Bokarev, V. Bondar, O. Ilinich and S. Shishatskii, IUPAC-NIST Solubility Data Series 70. Solubility of Gases in Glassy Polymers, J. Phys. Chem. Ref. Data, 1999, 28, 1255–1450 CrossRef CAS.
- L. Lee and K. Wilson, The Reactive-Diffusive Length of OH and Ozone in Model Organic Aerosols, J. Phys. Chem. A, 2016, 120, 6800–6812 CrossRef CAS PubMed.
- L. Chaix, H. v. d. Bergh and M. J. Rossi, Real-Time Kinetic Measurements of the Condensation and Evaporation of D2O Molecules on Ice at 140 K < T < 220 K, J. Phys. Chem. A, 1998, 102, 10300–10309 CrossRef CAS.
- Q. Shi, Y. Q. Li, P. Davidovits, J. T. Jayne, D. R. Worsnop, M. Mozurkewich and C. E. Kolb, Isotope exchange for gas-phase acetic acid and ethanol at aqueous interfaces: a study of surface reactions, J. Phys. Chem. B, 1999, 103, 2417–2430 CrossRef CAS.
- J. R. Lohr, B. S. Day and J. R. Morris, Scattering, accommodation, and trapping of HCl in collisions with a hydroxylated self-assembled monolayer, J. Phys. Chem. B, 2005, 109, 15469–15475 CrossRef CAS PubMed.
- B. Fluckiger and M. J. Rossi, Common Precursor Mechanism for the Heterogeneous Reaction of D2O, HCl, HBr, and HOBr with Water Ice in the Range 170–230 K: Mass Accommodation Coefficients on Ice, J. Phys. Chem. A, 2003, 107, 4103–4115 CrossRef CAS.
- A. Morita and B. C. Garrett, Molecular theory of mass transfer kinetics and dynamics at gas–water interface, Fluid Dyn. Res., 2008, 40, 459–473 CrossRef.
- M. A. Wilson and A. Pohorille, Adsorption and Solvation of Ethanol at the Water Liquid-Vapor Interface: A Molecular Dynamics Study, J. Phys. Chem. B, 1997, 101, 3130–3135 CrossRef CAS PubMed.
- R. S. Taylor and B. C. Garrett, Accommodation of Alcohols by the Liquid/Vapor Interface of Water: Molecular Dynamics Study, J. Phys. Chem. B, 1999, 103, 844–851 CrossRef CAS.
- C. J. H. Knox and L. F. Phillips, Capillary-Wave Model of Gas-Liquid Exchange, J. Phys. Chem. B, 1998, 102, 8469–8472 CrossRef CAS.
- B. C. Garrett, G. K. Schenter and A. Morita, Molecular Simulations of the Transport of Molecules across the Liquid/Vapor Interface of Water, Chem. Rev., 2006, 106, 1355–1374 CrossRef CAS.
- P. Davidovits, D. R. Worsnop, L. R. Williams, C. E. Kolb and M. Gershenzon, Comment on “Mass Accommodation Coefficient of Water: Molecular Dynamics Simulation and Revised Analysis of Droplet Train/Flow Reactor Experiment”, J. Phys. Chem. B, 2005, 109, 14742–14746 CrossRef CAS PubMed.
- R. L. Pecsok and B. H. Gump, Gas-liquid interface and solid support effects of polar solute-nonpolar solvent systems in gas chromatography, J. Phys. Chem., 1967, 71, 2202–2209 CrossRef CAS.
- C. D. Wick, J. I. Siepmann and M. R. Schure, Molecular Simulation of Concurrent Gas–Liquid Interfacial Adsorption and Partitioning in Gas–Liquid Chromatography, Anal. Chem., 2002, 74, 3518–3524 CrossRef CAS PubMed.
- M. Tascon, L. M. Romero, A. Acquaviva, S. Keunchkarian and C. Castells, Determinations of gas–liquid partition coefficients using capillary chromatographic columns. Alkanols in squalane, J. Chromatogr. A, 2013, 1294, 130–136 CrossRef CAS PubMed.
- M. von Domaros, Y. Liu, J. L. Butman, E. Perlt, F. M. Geiger and D. J. Tobias, Molecular Orientation at the Squalene/Air Interface from Sum Frequency Generation Spectroscopy and Atomistic Modeling, J. Phys. Chem. B, 2021, 125, 3932–3941 CrossRef CAS PubMed.
- H. Z. Zhang, Y. Q. Li, P. Davidovits, L. R. Williams, J. T. Jayne, C. E. Kolb and D. R. Worsnop, Uptake of gas-phase species by 1-octanol. 2. uptake of hydrogen halides and acetic acid as a function of relative humidity and temperature, J. Phys. Chem. A, 2003, 107, 6398–6407 CrossRef CAS.
Footnote |
† Electronic supplementary information (ESI) available. See DOI: 10.1039/d1sc02662c |
|
This journal is © The Royal Society of Chemistry 2021 |
Click here to see how this site uses Cookies. View our privacy policy here.