DOI:
10.1039/D1SC01987B
(Edge Article)
Chem. Sci., 2021,
12, 9946-9951
Cyclic monoterpenes trapped in a polyaromatic capsule: unusual selectivity, isomerization, and volatility suppression†
Received
9th April 2021
, Accepted 17th May 2021
First published on 18th May 2021
Abstract
Cyclic monoterpenes (CMTs) are intractable natural products with high volatility and strong odors so that there has been no molecular receptor capable of selectively and tightly trapping CMTs in both solution and the solid state. We herein report that a polyaromatic capsule acts as a functional nanoflask for CMTs with the following five features: (i) the capsule can selectively bind menthone from mixtures with other saturated CMTs in water. In contrast, (ii) treatment of the capsule with mixtures of menthone and π-conjugated CMTs gives rise to ternary host–guest complexes with high pair-selectivity. Notably, (iii) the encapsulated menthone displays unusual isomerization from a typical chair conformer to otherwise unstable conformers upon heating. (iv) The selective binding of volatilized CMTs is demonstrated by the capsule even in the solid state at atmospheric pressure. Furthermore, (v) the volatilities of CMTs are significantly suppressed at elevated temperatures by the capsule upon encapsulation in solution as well as in the solid state.
Introduction
Cyclic monoterpenes (CMTs) are simple yet important natural products, selectively biosynthesized from an acyclic monoterpene derivative in plants (Fig. 1a).1 The bioproducts are widely used as common fragrances, essences, and perfumes, due to their characteristic odors, and as key starting materials for drug synthesis.2,3 The relatively small structures (i.e., ∼0.7 nm and ∼200 Da) and the absence of strongly interactive substituents make CMTs highly volatile. Thus, these intractable features cause great difficulty in their molecular-level recognition and microscale separation from CMT mixtures, besides the existence of plenty of derivatives with only tiny structural differences.4 There have been many reports on molecular receptors (e.g., covalent tubular and bowl-shaped compounds,5 hydrogen-bonding and coordination capsules,6 and π-stacking capsules7) capable of binding CMTs such as camphor (CMP), menthol (MTL), and p-cymene (CMN; Fig. 1a). However, the majority of the previous receptors possess open and/or flexible cavities surrounded by aliphatic and/or small aromatic frameworks5–8 so that the selective recognition and high volatility suppression of CMTs have been hardly accomplished by the artificial receptors so far. What type of molecular receptor is the most suitable for these bioproducts? We herein focused on polyaromatic capsule 1 (Fig. 1b and c) to strategically utilize multiple CH–π and hydrogen-bonding interactions with CMTs in the closed cavity (Fig. 1d).
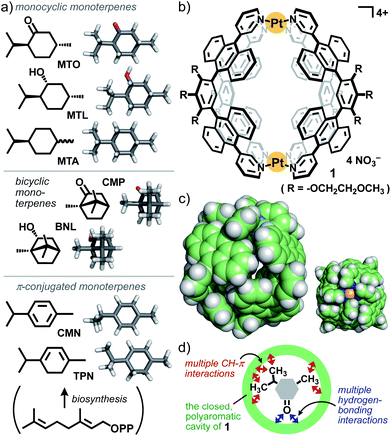 |
| Fig. 1 (a) Cyclic monoterpenes (CMTs; OPP = pyrophosphate) and (b) polyaromatic capsule 1 used in this work as volatile biosubstrates and a receptor, respectively. (c) Crystal structure of 1 (top and side views; the hydrophilic side chains are replaced by hydrogen atoms for clarity). (d) Strategy for the selective recognition of CMTs by 1. | |
Capsule 1, with a simple M2L4 composition, provides a spherical cavity with relatively high rigidity and a diameter of ∼1 nm (Fig. 1b),9 in contrast to biological cavities with high flexibility and complex shapes. In water, the polyaromatic cavity of 1 binds various biomolecules, e.g., relatively large androgens (∼1.4 nm in length) and medium-sized xanthines and disaccharides (∼1.0 nm in length), with a high selectivity through efficient host–guest interactions.10 Here we disclose that, unlike the previous receptors,5–8 capsule 1 acts as a functional nanoflask toward relatively small CMTs with the following novel characteristics. (i) The capsule binds menthone (MTO; Fig. 1a) with high efficiency and selectivity from mixtures with other saturated CMTs (e.g., MTL and CMP) in water at room temperature. The host–guest structure is fully confirmed by NMR, MS, ITC, and X-ray crystallographic analyses. On the other hand, (ii) MTO is pair-selectively bound by 1 with π-conjugated CMTs (i.e., CMN and TPN) under the same conditions. In the confined cavity, (iii) unusual thermal isomerization of MTO from a typical diequatorial chair conformer to otherwise unstable diaxial chair and twist-boat conformers can be observed in a quantitative and reversible fashion. The cavity-directed, conformational stabilization of the resultant isomers is supported by theoretical calculations. (iv) The selective binding of volatilized MTO is also accomplished by solid 1 at ambient temperature and pressure. Finally, we reveal that (v) capsule 1 significantly suppresses the volatilities of CMTs upon encapsulation in solution as well as in the solid state. Combined with the crystal structures, the present behaviour demonstrates the presence of highly efficient CH–π and hydrogen-bonding interactions between the polyaromatic host cavity and the CMT guests.
Results and discussion
Selective trap of CMTs by the capsule in water
Capsule 1 bound various CMTs to give the corresponding 1
:
1 host–guest complexes in a quantitative fashion and displayed a distinct binding preference. When a mixture of (−)-menthone (MTO), p-menthane (MTA), and (−)-menthol (MTL; 10 equiv. each) was suspended in a D2O solution (0.5 mL) of 1 (0.26 μmol) at room temperature for 1 h, host–guest complexes 1·MTO, 1·MTA, and 1·MTL were formed in 90, 10, and 0% yields, respectively (Fig. 2a, left).11 In the 1H NMR spectrum, the three methyl and six methylene signals of encapsulated MTO were observed in the range of −2.65 to −0.71 ppm. These guest signals are outstandingly shifted upfield, due to the efficient shielding effect (Δδmax = −4.09 ppm) of the polyaromatic host (Fig. 2b and c). Selective formation of 1·MTO was further confirmed by the ESI-TOF MS spectrum, showing a series of prominent peaks derived from the [1·MTO–n·NO3−]n+ species (n = 4 to 2; Fig. S4†). A similar binding study clarified that 1 binds MTA stronger than MTL (in a 67
:
33 ratio; Fig. S17†).11 Therefore, the present competitive experiments elucidated the binding preference in the order of MTO ≫ MTA > MTL. The binding constant (Ka) of 1 toward MTO was estimated to be 6.6 × 105 M−1 by isothermal titration calorimetry (ITC) analysis (Fig. S20 and Table S1†).11 The obtained thermodynamics indicate that the guest binding is an enthalpically favorable process (ΔH = −84.5 kJ mol−1, TΔS = −51.5 kJ mol−1 (25 °C)), through multiple host–guest interactions.
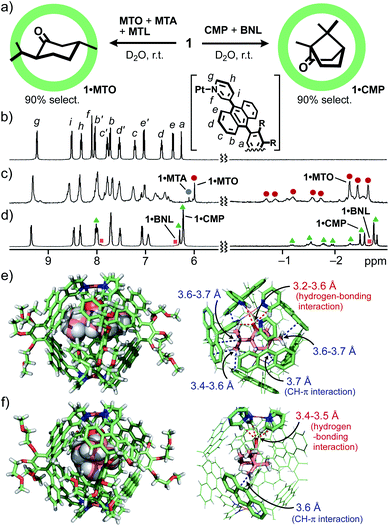 |
| Fig. 2 (a) Selective binding of MTO from a mixture of MTO, MTA, and MTL (left side) and CMP from a mixture of CMP and BNL (right side) by 1. 1H NMR spectra (500 MHz, D2O, r.t.) of (b) 1 and host–guest complexes obtained from mixtures of (c) 1, MTO, MTA, and MTL, and (d) 1, CMP, and BNL after 1 h at r.t. X-ray crystal structures of (e) 1·MTO and (f) 1·CMP, and their host–guest interactions. | |
Whereas bicyclic monoterpenes such as (−)-camphor (CMP) and (−)-borneol (BNL) were also quantitatively bound by 1 in water (Fig. S9–S13†), their binding affinities were lower than those of the monocyclic ones. Treatment of a mixture of CMP and BNL (10 equiv. each) with 1 in D2O gave rise to 1·CMP and 1·BNL in a 9
:
1 ratio (Fig. 2a, right and 2d).12 Since 1 bound MTL more efficiently (65% selectivity) than CMP from a 1
:
1 MTL/CMP mixture (Fig. S19†),11 the present competitive studies established the binding affinities of 1 for the tested CMTs to be MTO ≫ MTA > MTL > CMP ≫ BNL. This order stems not from the guest solubility in water (Fig. S23†) but from the identity of the guest shape and substituents. High recognition ability of 1 toward MTO most probably results from its planar structure (0.9 nm in the longest length) with three methyl groups and one carbonyl group (Fig. 1d). Eventually, the X-ray crystallographic analysis of 1·MTO indicated that the functional groups of MTO, adopting a typical diequatorial chair conformer, efficiently interact with the polyaromatic host cavity through seven CH–π and four CH⋯O
C hydrogen-bonding interactions (Fig. 2e).10c,13 The number of the observed host–guest interactions within 1·MTO is larger than that within 1·CMP (i.e., one CH–π and four CH⋯O
C interactions; Fig. 2f), which is consistent with the ITC data in solution (ΔH = −84.5 and −14.9 kJ mol−1, respectively).11,12 The spherical structure of CMP (∼0.6 nm in diameter) is too small to efficiently contact with the spherical cavity (∼1.0 nm) of 1.
Pairwise encapsulation of CMTs by the capsule
Pairwise encapsulation of CMTs exceptionally took place, when 1 was combined with MTO and π-conjugated CMTs, such as p-cymene (CMN) and α-terpinene (TPN), in water.14 Although there is no electrostatic interaction between MTO and CMN, ternary host–guest complex 1·(MTO·CMN) was generated exclusively from a 1
:
1 mixture of MTO and CMN (10 equiv. each), being stirred in a D2O solution of 1 (0.52 mM) at room temperature for 1 h (Fig. 3a, left). The 1H NMR spectrum of the product displayed new signals derived from the host and the two guests (Fig. 3c), which are completely different from those of 1·MTO and 1·(CMN)2, prepared separately (Fig. 3b and d, respectively). The formation of the ternary complex was indicated by ESI-TOF MS analysis, where the MS peaks corresponding to [1·(MTO·CMN)–n·NO3−]n+ (n = 4 to 2) species were observed at m/z = 976.6, 1322.4, and 2014.6 (Fig. 3e).14 The 1
:
1 ratio of guests MTO and CMN within 1 was further confirmed by 1H NMR analysis after extraction of the encapsulated guests with CDCl3. Notably, the selective formation of 1·(MTO·CMN) (>98%) was found even using a 1
:
5
:
50 mixture of 1, MTO, and CMN in water at room temperature (Fig. S28†).11
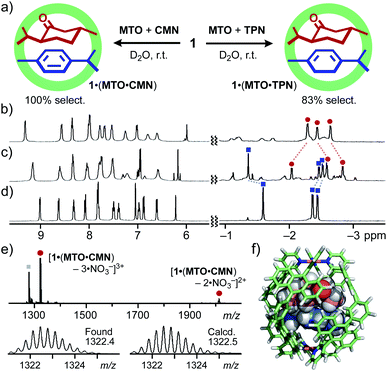 |
| Fig. 3 (a) Selective formation of ternary host–guest complexes 1·(MTO·CMN) and 1·(MTO·TPN). 1H NMR spectra (500 MHz, D2O, r.t.) of (b) 1·MTO, (c) 1·(MTO·CMN), and (d) 1·(CMN)2. (e) ESI-TOF MS spectrum (H2O) of 1·(MTO·CMN) (gray square: 1·MTO) and the expanded and simulated signals of [1·(MTO·CMN)–3NO3−]3+. (f) Optimized structure of 1·(MTO·CMN) (R = –H). | |
The observed pair-selectivity is ascribed to structure-based complementary guest uptake. The optimized structure of 1·(MTO·CMN) suggested that the size and shape of a stacked heterodimer of planar MTO (diequatorial chair form) and CMN nicely fit those of the polyaromatic cavity of 1 (Fig. 3f).15 The π-conjugated framework of CMN is essential for the pair-selective encapsulation with MTO so that 1·(MTO·TPN) also formed in high selectivity (83%) by the treatment of 1 with MTO and TPN, bearing a cyclohexadiene ring, under the same conditions (Fig. 3a, right). In contrast, the formation of a ternary complex was undetected from a mixture of 1, MTO, and MTA under various conditions (Fig. S15†).
Thermal isomerization of menthone within the capsule
In the confined cavity of 1, a unique conformation change of MTO was observed from a typical chair form to otherwise labile forms by thermal stimuli. In the 1H NMR spectra of 1·MTO in D2O, unexpectedly, the aromatic host signals and the aliphatic guest signals were fully shifted upon heating at 100 °C for 8 h, without the appearance of empty host signals (Fig. 4a and b, left). Particularly, the downfield shift of the inner protons (Ha) of 1 and its splitting into three signals suggested the generation of three new species derived from MTO in the cavity.16 The IR analysis of 1·MTO showed the C
O stretching band of the encapsulated MTO being also shifted by 10 cm−1 through the heating (Fig. S36†). On the other hand, the ESI-TOF MS peaks of the host–guest complex were unchanged before and after the treatment (Fig. 4a and b, right). The new host and guest NMR signals gradually went back to the initial positions at 30 °C (τ1/2 = 22 h; Fig. S37d†).11,17 The signal shifts could be repeated at least three times without the decomposition of the host–guest complex (Fig. S37c†). These findings indicate the thermal isomerization of MTO in the cavity of 1 in water.
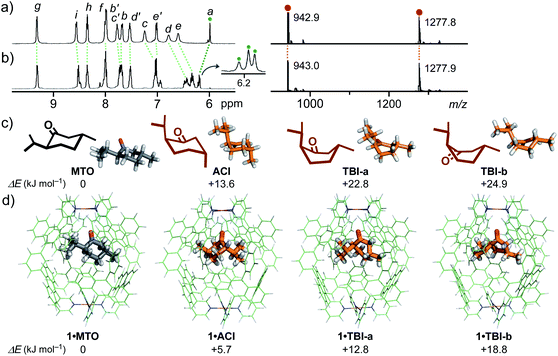 |
| Fig. 4
1H NMR spectra (500 MHz, D2O, r.t., left) and ESI-TOF MS spectra (H2O, right) of 1·MTO (a) before and (b) after heating at 100 °C for 8 h. (c) Optimized structures of MTO, ACI, TBI-a, and TBI-b (c) without 1 and (d) within 1, and their relative energies based on MTO or 1·MTO (the peripheral substituents are replaced with hydrogen atoms for clarity). | |
Theoretical calculation studies suggested the formation of diaxial-type chair isomer ACI and twist-boat isomers TBI-a (major) and TBI-b (minor) upon heating and their large conformational stabilization within 1 (Fig. 4c).11,18 Whereas the energy of ACI is 13.6 kJ mol−1 higher than that of MTO (diequatorial-type chair form) without 1 (Fig. 4c), the energy difference (ΔE) between 1·ACI and 1·MTO decreased to be 5.7 kJ mol−1 (Fig. 4d). Accordingly, the diaxial chair conformer was stabilized by 7.9 kJ mol−1 upon encapsulation, as compared with the diequatorial chair conformer. Conformers TBI-a and TBI-b are also stabilized by 10.0 and 6.0 kJ mol−1 within 1, respectively. In the optimized structures, roughly bowl-shaped ACI and TBI are suitably accommodated in the spherical cavity of 1 (Fig. 4d), displaying multiple host–guest CH–π and hydrogen-bonding interactions (Fig. S40b†). To the best of our knowledge, there has been no report on the spectroscopic observation of the diaxial to diequatorial19 and chair to twist-boat isomerizations20 of CMTs as well as other cyclohexane derivatives in solution.
Selective trap of volatilized CMTs by the capsule solid
Inspired by the solution-state binding ability of 1 toward CMTs, we next examined the binding of volatilized CMTs using solid 1.21,22 To our surprise, a solid of 1 could efficiently and selectively bind MTO from a mixture with volatilized CMTs at room temperature and atmospheric pressure. Each of MTO, MTA, and MTL (100 equiv.) was placed in a closed glass vessel (50 mL) including 1 (0.26 μmol) without direct host–guest contact (Fig. 5a). After standing for 14 h, the resultant solid was exposed to reduced pressure (480 Pa, 1 h) to remove CMTs outside the solid. The guest binding and its selectivity were elucidated by 1H NMR analysis in D2O. The NMR spectrum indicated that 1 binds the guests quantitatively to yield host–guest complexes 1·MTO, 1·MTL, and 1·MTA in 70, 30, and 0%, respectively (Fig. 5b). Solid 1 also bound volatilized MTO and CMN pair-selectively to give 1·(MTO·CMN) in 95% yield after standing for 2 d (Fig. 5c).
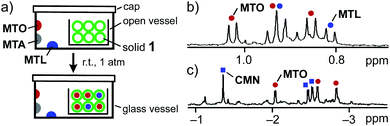 |
| Fig. 5 (a) Selective binding of volatilized MTO from a mixture of CMTs by solid 1. (b) 1H NMR spectrum (400 MHz, CDCl3, r.t.) of guests extracted from solid 1 after treatment with MTO, MTA, and MTL. (c) 1H NMR spectrum (500 MHz, D2O, r.t.) of host–guest complexes obtained from a mixture of solid 1 and volatilized MTO and CMN. | |
It is noteworthy that each of the tested CMTs was quantitatively encapsulated by 1 in water, as described above, whereas solid 1 displayed largely different affinity toward each of the volatilized CMTs, e.g., quantitative binding to MTO yet weak binding to CMP (4%) under the same conditions (Fig. S45†). The rigid and sterically demanding features of CMP are major reasons for the selectivity.
Volatility suppression of CMTs by the capsule
Notably, the volatility of CMTs was largely suppressed upon encapsulation by 1 in both solution and the solid state. When a mixture of 1·CMP and CMP (∼12 equiv.) in D2O was heated at 80 °C, the majority of free CMP (96%) was volatilized after 25 h. On the other hand, CMP within 1 remained fully intact under the same conditions, as confirmed by time-dependent 1H NMR and ESI-TOF MS analyses (Fig. 6a and b).11 No peaks derived from empty 1 were detected in the MS spectrum. On the basis of the NMR studies, the kinetic analysis of the guest volatilization revealed that the half-lives (τ1/2) of free and encapsulated CMP are approximately 5 and >690 h, respectively (Fig. 6c), indicating an encapsulation-induced >140 times suppression of the thermal volatility. In a similar way, the volatilization of MTL was suppressed by a factor of >70 upon encapsulation by 1 (Fig. S47†).11
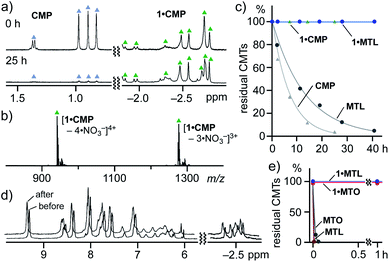 |
| Fig. 6 (a) 1H NMR spectra (500 MHz, D2O, r.t.) of free CMP and 1·CMP before/after heating at 80 °C for 25 h in water, and (b) ESI-TOF MS spectrum (H2O) of the resultant solution after heating. (c) Time-course profile of the volatilization (%) of CMP and MTL within/without 1 in water at 80 °C. (d) 1H NMR spectra (500 MHz, D2O, r.t.) of 1·MTO before/after heating at 80 °C under vacuum for 1 h in the solid state. (e) Time-course profile of the volatilization (%) of MTO and MTL within/without 1 in the solid state at 80 °C under vacuum. | |
Moreover, encapsulated CMTs (i.e., MTL and MTO) in the cavity of solid 1 hardly volatilized even under high vacuum at elevated temperature (i.e., 480 Pa and 80 °C) for 1 h, as confirmed by 1H NMR analysis (Fig. 6d and e). In contrast, free MTL and MTO volatilized instantly (<4 min) under the same conditions.23 Thermal gravimetric (TG) analysis of solid 1·MTO showed 4.4% weight loss in the range of 88–200 °C, assignable to the release of the encapsulated MTO (Fig. S49†). The present results demonstrated that 1 acts as an excellent molecular storage for volatile CMTs in solution as well as in the solid state.24
Conclusions
We have revealed the new functions of a polyaromatic capsule acting as a nanoflask for cyclic monoterpenes (CMTs) both in solution and in the solid state. Although the recognition of CMTs is highly challenging due to their simple structure and high volatility, the capsule preferentially bound menthone from a mixture of CMTs in water and in the gas phase under ambient conditions. Unusual pair-selective encapsulations of two different CMTs (e.g., menthone and p-cymene) were also accomplished by the capsule. Moreover, the capsule prompted the thermal isomerization of menthone and largely suppressed the volatilities of CMTs in the confined cavity. This level of control over CMTs has not been accomplished with previous molecular receptors5–8 and solid materials.25 We hope that the present work will open the door to new host–guest chemistry of polyaromatic nanoflasks26 toward not only natural but also synthetic volatile compounds.
Author contributions
R. S., Y. T., K. N., S. T., and M. Y. designed the work, carried out research, analyzed data, and wrote the paper. Y. S. contributed to crystallographic analysis. M. Y. is the principal investigator. All authors discussed the results and commented on the manuscript.
Conflicts of interest
There are no conflicts to declare.
Acknowledgements
This work was supported by JSPS KAKENHI (Grant No. JP18H01990/JP19H04566) and “Support for Tokyo Tech Advanced Researchers (STAR)”. The computations were performed using computers in Research Center for Computational Science, Okazaki, Japan.
Notes and references
-
C. Sell in Handbook of Essential Oils Science, Technology, and Applications, ed K. H. C. Başer and G. Buchbauer, CRC Press, 2009, pp. 131–135 Search PubMed.
- P. K. Ajikumar, K. Tyo, S. Carlsen, O. Mucha, T. H. Phon and G. Stephanopoulos, Mol. Pharm., 2008, 167–190 Search PubMed.
- A. G. Guimarães, J. S. S. Quintans and L. J. Quintans-Júnior, Phytother Res., 2013, 27, 1–15 Search PubMed.
- Z. Jiang, C. Kempinski and J. Chappell, Curr. Protoc. Plant Biol., 2016, 1, 345–358 Search PubMed.
-
(a) C. Donze and A. W. Coleman, J. Inclusion Phenom. Mol. Recognit. Chem., 1996, 16, 1–15 Search PubMed;
(b) G. Ramon, A. W. Coleman and L. R. Nassimbeni, Cryst. Growth Des., 2006, 6, 132–136 Search PubMed;
(c) M. A. Romero, N. Basílio, A. J. Moro, M. Domingues, J. A. González-Delgado, J. F. Arteaga and U. Pischel, Chem.–Eur. J., 2017, 23, 13105–13111 Search PubMed.
-
(a) Y. Tokunaga and J. Rebek Jr, J. Am. Chem. Soc., 1998, 120, 66–69 Search PubMed;
(b) J. L. Bolliger, T. K. Ronson, M. Ogawa and J. R. Nitschke, J. Am. Chem. Soc., 2014, 136, 14545–14553 Search PubMed.
- A. Suzuki, K. Kondo, Y. Sei, M. Akita and M. Yoshizawa, Chem. Commun., 2016, 52, 3151–3154 Search PubMed.
- Recent reviews on coordination cages and capsules:
(a) T. R. Cook and P. J. Stang, Chem. Rev., 2015, 115, 7001–7045 Search PubMed;
(b) C. J. Brown, F. D. Toste, R. G. Bergman and K. N. Raymond, Chem. Rev., 2015, 115, 3012–3035 Search PubMed;
(c) L.-J. Chen, H.-B. Yang and M. Shionoya, Chem. Soc. Rev., 2017, 46, 2555–2576 Search PubMed;
(d) I. Sinha and P. S. Mukherjee, Inorg. Chem., 2018, 57, 4205–4221 Search PubMed;
(e) F. J. Rizzuto, L. K. S. von Krbek and J. R. Nitschke, Nat. Rev. Chem., 2019, 3, 204–222 Search PubMed;
(f) H. Sepehrpour, W. Fu, Y. Sun and P. J. Stang, J. Am. Chem. Soc., 2019, 141, 14005–14020 Search PubMed;
(g) A. B. Grommet, M. Feller and R. Klajn, Nat. Nanotechnol., 2020, 15, 256–271 Search PubMed;
(h) E. G. Percástegui, T. K. Ronson and J. R. Nitschke, Chem. Rev., 2020, 120, 13480–13544 Search PubMed;
(i) K. Ariga and M. Shionoya, Bull. Chem. Soc. Jpn., 2021, 94, 839–859 Search PubMed.
-
(a) N. Kishi, Z. Li, K. Yoza, M. Akita and M. Yoshizawa, J. Am. Chem. Soc., 2011, 133, 11438–11441 Search PubMed;
(b) M. Yoshizawa and L. Catti, Acc. Chem. Res., 2019, 52, 2392–2404 Search PubMed.
-
(a) M. Yamashina, S. Matsuno, Y. Sei, M. Akita and M. Yoshizawa, Chem.–Eur. J., 2016, 22, 14147–14150 Search PubMed;
(b) M. Yamashina, M. Akita, T. Hasegawa, S. Hayashi and M. Yoshizawa, Sci. Adv., 2017, 3, e1701126 Search PubMed;
(c) S. Kusaba, M. Yamashina, M. Akita, T. Kikuchi and M. Yoshizawa, Angew. Chem., Int. Ed., 2018, 57, 3706–3710 Search PubMed;
(d) M. Yamashina, T. Tsutsui, Y. Sei, M. Akita and M. Yoshizawa, Sci. Adv., 2019, 5, eaav3179 Search PubMed;
(e) K. Niki, T. Tsutsui, M. Yamashina, M. Akita and M. Yoshizawa, Angew. Chem., Int. Ed., 2020, 59, 10489–10492 Search PubMed;
(f) H. Dobashi, L. Catti, Y. Tanaka, M. Akita and M. Yoshizawa, Angew. Chem., Int. Ed., 2020, 59, 11881–11885 Search PubMed;
(g) T. Tsutsui, L. Catti, K. Yoza and M. Yoshizawa, Chem. Sci., 2020, 11, 8145–8150 Search PubMed.
- See the ESI.† Pale-yellow single crystals were obtained by slow concentration of the H2O solutions of 1·MTO and 1·CMP at room temperature for ∼40 d.
- The ITC analysis of 1·CMP also revealed its thermodynamic parameters (i.e., ΔH = −14.9 kJ mol−1, TΔS = 15.8 kJ mol−1 (25 °C), and Ka = 2.4 × 105 M−1; Fig. S21 and Table S1†).11.
-
(a) D. P. August, C. S. Nichol and P. J. Lusby, Angew. Chem., Int. Ed., 2016, 128, 15246–15250 Search PubMed;
(b) V. Martí-Centelles, A. L. Lawrence and P. J. Lusby, J. Am. Chem. Soc., 2018, 140, 2862–2868 Search PubMed;
(c) T. A. Young, V. Martí-Centelles, J. Wang, F. Duarte and P. J. Lusby, J. Am. Chem. Soc., 2020, 142, 1300–1310 Search PubMed.
- Pair-selective encapsulation of biomolecules by a synthetic host is very rare: P. Mateus, N. Chandramouli, C. D. Mackereth, B. Kauffmann, Y. Ferrand and I. Huc, Angew. Chem., Int. Ed., 2020, 59, 5797–5805 Search PubMed.
- Ternary complex 1·(MTO·CMN) is not stable enough under the MS condition and thus MS peaks derived from 1·MTO were observed at m/z = 942.8 and 1278.0 (Fig. 3e and S29†). Theoretical studies of the host–guest complexes indicated that the formation of 1·(MTO·CMN) is more favorable (>25 kJ mol−1) than that of 1·MTO or 1·(CMN)2 in the gas phase (Fig. S31†).
- The 1H NMR, 1H–1H COSY, and HSQC analyses of 1·MTO after the thermal treatment indicated the formation of three isomers of MTO, besides the diequatorial chair form (Fig. S35a–c†).
- The activation energy (ΔG‡) of the thermal isomerization of MTO within 1 was estimated to be 92.8 kJ mol−1 at 303 K, by the Eyring plots (Fig. S37†).
- The conformational isomers of MTO (20 structures) were initially generated through automatic conformational analysis using Conflex 8, Rev. C program and subsequently their optimized structures and energies were obtained by DFT calculations (CAM-B3LYP/6-31G(d) level of theory, gas phase; Fig. S40a†). Furthermore, the optimized structures and energies were obtained from the host–guest complexes, using 1 (R = –OCH3) and the most stable four conformers of MTO, by DFT calculations (CAM-B3LYP/LanL2DZ (for Pt), 6-31G(d) (for C, H, N, O) level of theory, gas phase for optimization and PCM(H2O) for estimation of energies; Fig. S40b†).
- Stabilization of the axial conformer of chlorocyclohexane in the crystalline state: S. Hirano, S. Toyota, M. Kato and F. Toda, Chem. Commun., 2005, 3646–3648 Search PubMed.
-
(a) M. Squillacote, R. S. Sheridan, O. L. Chapman and F. A. L. Anet, J. Am. Chem. Soc., 1975, 97, 3244–3246 Search PubMed;
(b) G. Gill, D. M. Pawar and E. A. Noe, J. Org. Chem., 2005, 70, 10726–10731 Search PubMed;
(c) A. Krin, C. Pérez, P. Pinacho, M. M. Quesada-Moreno, J. J. López-González, J. R. Avilés-Moreno, S. Blanco, J. C. López and M. Schnell, Chem.–Eur. J., 2018, 24, 721–729 Search PubMed.
- Gas molecules bound by coordination cages in solution:
(a) I. A. Riddell, M. M. J. Smulders, J. K. Clegg and J. R. Nitschke, Chem. Commun., 2011, 47, 457–459 Search PubMed;
(b) J. Roukala, J. Zhu, C. Giri, K. Rissanen, P. Lantto and V.-V. Telkki, J. Am. Chem. Soc., 2015, 137, 2464–2467 Search PubMed;
(c) C. Browne, W. J. Ramsay, T. K. Ronson, J. Medley-Hallam and J. R. Nitschke, Angew. Chem., Int. Ed., 2015, 54, 11122–11127 Search PubMed;
(d) L. Ma, C. J. E. Haynes, A. B. Grommet, A. Walczak, C. C. Parkins, C. M. Doherty, L. Longley, A. Tron, A. R. Stefankiewicz, T. D. Bennett and J. R. Nitschke, Nat. Chem., 2020, 12, 270–275 Search PubMed.
-
(a) D. Preston, K. F. White, J. E. M. Lewis, R. A. S. Vasdev, B. F. Abrahams and J. D. Crowley, Chem.–Eur. J., 2017, 23, 10559–10567 Search PubMed;
(b) J. S. Wright, A. J. Metherell, W. M. Cullen, J. R. Piper, R. Dawson and M. D. Ward, Chem. Commun., 2017, 53, 4398–4401 Search PubMed.
- Free MTO volatilized over 25 min at atmospheric temperature and pressure (Fig. S48e†).
-
(a) β-Cyclodextrin and its derivative show weak volatile suppression effects in water (e.g., ∼50% loss of CMP at 60 °C for 100 min and 60% loss of MTL at 40 °C for 5 h);
(b) M. Tanaka, H. Matsuda, H. Sumiyoshi, H. Arima, F. Hirayama, K. Uesaka and S. Tsuchiya, Chem. Pharm. Bull., 1996, 44, 416–420 Search PubMed;
(c) N. Ajisaka, K. Hara, K. Mikuni, K. Hara and H. Hashimoto, Biosci., Biotechnol., Biochem., 2000, 64, 731–734 Search PubMed.
-
(a) T. Gruber, C. Fischer, W. Seichter, P. Bombicz and E. Weber, CrystEngComm, 2011, 11, 1422–1431 Search PubMed;
(b) J. Zhang, M. Yu, P. Yuan, G. Lu and C. Yu, J. Inclusion Phenom. Macrocyclic Chem., 2011, 71, 593–602 Search PubMed.
- Reviews on polyaromatic capsules, cages, and tubes:
(a) M. Yoshizawa and M. Yamashina, Chem. Lett., 2017, 46, 163–171 Search PubMed;
(b) K. Yazaki, L. Catti and M. Yoshizawa, Chem. Commun., 2018, 54, 3195–3206 Search PubMed.
Footnote |
† Electronic supplementary information (ESI) available. CCDC 2058897 and 2059097. For ESI and crystallographic data in CIF or other electronic format see DOI: 10.1039/d1sc01987b |
|
This journal is © The Royal Society of Chemistry 2021 |