DOI:
10.1039/D1SC01616D
(Edge Article)
Chem. Sci., 2021,
12, 9977-9982
A fluorescent probe for the discrimination of oxidation states of palladium†‡
Received
21st March 2021
, Accepted 24th June 2021
First published on 24th June 2021
Abstract
Palladium-based catalysts are widely used in pharmaceutical industries, which can sometimes cause palladium contamination in pharmaceutical drug manufacture. It is important to separately quantify the different oxidation states of palladium (Pd0 and Pd2+) in pharmaceuticals as they react with scavengers differently. Although palladium sensors have been under intense investigation, oxidation state differentiators are very rare. Here, we report a simple porphyrin–coumarin conjugate, PPIX-L2, that can selectively discriminate between the oxidation states of palladium. The reaction of PPIX-L2 with Pd0 showed a 24-fold fluorescence increase of the coumarin emission, meanwhile, the presence of Pd2+ led to a 98% quenching of the porphyrin emission. Fluorescent responses of PPIX-L2 towards Pd0 and Pd2+ are specific, and its sensitivity towards both palladium species is significantly increased with a detection limit of 75 nM and 382 nM for Pd0 and Pd2+ respectively.
Introduction
Palladium-catalysed reactions have become an indispensable tool in organic synthesis due to their strong ability and high efficiency in building versatile organic compounds.1 This has realized a vast number of applications, not only in the research laboratory, but also in pharmaceutical industries.2,3 In recent years, alternative non-precious metal-based catalysts including copper, nickel, and iron, have been developed.4,5 However, palladium-based catalysts often offer significantly shorter routes towards the desired products due to their relatively higher activity in enabling the conversion of less reactive substrates, or at lower temperatures.6,7 Shorter synthetic routes reduce the formation of side-products and waste, both of which are important points that have to be taken into account by pharmaceutical factories to minimize the plant costs.
However, when using ‘precious metals’ such as palladium for the production of pharmaceuticals, additional points have to be considered e.g. the possibility of contaminating the products with metal. Residual palladium in the body is highly toxic as it can bind to thiol-containing proteins or other biomacromolecules.8–10 Usually, the amount of heavy metals in an active pharmaceutical ingredient has to be controlled to levels below 5–10 ppm.11,12 If higher, specific scavengers are applied to remove the metal, thus substantial efforts are required to analyse residual metals. As Pd0 and Pd2+ bind to scavengers differently,12,13 it is necessary to separately detect and quantify the palladium species in active pharmaceutical ingredients.
Small molecule-based fluorescent probes are versatile tools which are frequently explored as metal sensors.14–16 Although probes with novel structures or improved properties are routinely developed, it remains a very active research area. For example, two fluorescent rotaxane probes have been recently identified in Goldup's group as a selective Zn2+ and Pt2+ sensor.17,18 As for palladium, there are also numerous probes designed for detecting Pd0 (sometimes appeared as Pd2+ with reducing agents such as carbon monoxide and sodium borohydride)19–25 or Pd2+.26–31 However, to the best of our knowledge, very few reports have described the discrimination of Pd0 and Pd2+ within one fluorescent probe.32,33 Koide's group developed an elegant fluorescein-constructed sensor to discriminate between Pd0 and Pd2+ in a reaction-based manner in 2008.32 Incubation of the sensor with Pd0 and Pd2+ caused a fluorescence intensity increase at 526 nm and 535 nm respectively. The sensor is able to detect 10 ppm Pd0, and its detection limit for Pd2+ was calculated as 3.9 μM. Although the maximum emission wavelength caused by Pd0 and Pd2+ is different, specifically 526 nm by Pd0 and 535 nm by Pd2+, the 9 nm difference in the maximum wavelength is insignificant in the broad fluorescein emission, decreasing its sensitivity to discriminate Pd0 and Pd2+ in pharmaceutical drugs. A more recent example describing a non-reaction based colorimetric and anthraquinone-imidazole probe was reported by Kim and co-workers, where both Pd0 and Pd2+ demonstrated a quenching effect on the emission of the probe, with a calculated detection limit of 2.84 μM and 1.65 μM respectively.33 However, the discrimination between Pd0 and Pd2+ relied on the colour change of the probe, which is hard to quantify. Higher concentrations of palladium species are also required for an observable readout (in the micromolar range), making it impractical for analysis of the oxidative states of Pd0 and Pd2+ in active pharmaceutical ingredients, where concentrations are generally much lower.
Here we report an alternative strategy to differentiate between Pd0 and Pd2+ by using a simple porphyrin–coumarin conjugate PPIX-L2 (Fig. 1). Although porphyrins are known to bind metal ions, porphyrin derivatives have not been exploited as a probe for the discrimination of palladium species. The designed conjugate showed a significant increase of coumarin fluorescence at 440 nm and a quenching of porphyrin fluorescence at 631 nm towards Pd0 and Pd2+ respectively. Changes in coumarin and porphyrin fluorescence caused by Pd0 and Pd2+ are independent and have no interference on each other. PPIX-L2 also demonstrated a much lower detection limit towards Pd0 (75 nM) and Pd2+ (382 nM), which are as great as 38-fold and 10-fold lower, respectively, compared to the previously reported differentiators.
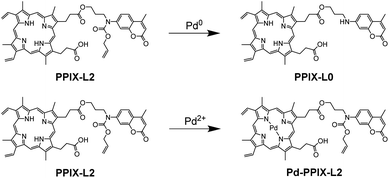 |
| Fig. 1 Proposed detection strategy for Pd0 and Pd2+. | |
Results and discussion
Design and synthesis of PPIX-L2
The synthesis of PPIX-L2 started from the substitution reaction of commercially available 7-amino-4-methylcoumarin and 3-bromo-1-propanol. The hydroxyl group in 3-bromo-1-propanol was first protected with tert-butyldimethylsilane (TBS), as it was found that it reacts with allyl chloroformate preferentially in the second step, due to the steric hindrance presented around the nitrogen atom in compound 1 (Scheme 1). Another route was also attempted towards the synthesis of 1, by reacting 7-amino-4-methylcoumarin with a halogenated alcohol first (n = 2, 3; X = Cl, Br, I), followed by alcohol protection with TBSCl (presented in pink in Scheme 1). Here, it was found that the halogenated alcohol starting material underwent an intramolecular reaction under these conditions, forming an ether bond, and significantly decreasing the reaction yield of the desired product. The length of the carbon chain and halogen reactivities in the TBS-protected alcohol towards 1 were also investigated. The three-carbon chain showed a better reactivity compared to the two-carbon chain as it further decreased the electron donating effect from the oxygen to the halogen, and hence increased its reactivity. Bromide was shown to have a higher reactivity than chloride. Given these observations, (3-bromopropoxy)-tert-butyldimethylsilane was chosen, and the finalized synthetic route was determined shown in the black route of Scheme 1 (characterization data shown in Fig. S6–S16‡).
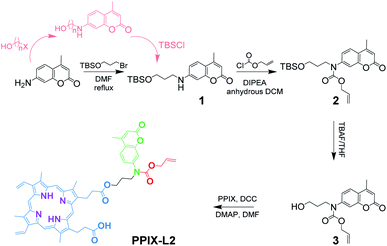 |
| Scheme 1 Synthetic route and chemical structure of PPIX-L2. | |
Distinctive fluorescence responses of PPIX-L2 to Pd0 and Pd2+
To study the behaviour of PPIX-L2 as a responsive sensor for Pd0, a fluorescence titration with Pd(PPh3)4 was conducted. To avoid Pd0 oxidation, N2 was bubbled through the solution during the measurements. The addition of Pd0 to PPIX-L2 in MeOH led to a gradual increase of the coumarin emission centred at 440 nm, as well the coumarin absorbance centred at around 353 nm, based on the well-studied Pd0-catalyzed Tsuji–Trost reaction (λex: 361 nm, Fig. 2a, S1 and S2‡).19–25,34 Increase of the coumarin fluorescence is time-dependent, a bar graph describing changes of fluorescence intensity over time is shown in Fig. 2b. PPIX-L2 was shown to quickly respond to Pd0, displaying a 1.5-fold fluorescence increase immediately after the addition of Pd0. A significant fluorescence increase can be observed in the first three hours, where the fourth hour displayed only a mild increase. A final 24-fold increase of the fluorescence intensity of PPIX-L2 at 440 nm was caused by Pd0. To verify the interaction of Pd0 with PPIX-L2 occurs through the Tsuji–Trost reaction mechanism, a MALDI-TOF mass analysis was performed for PPIX-L2 upon Pd0 addition. The product with expected mass of PPIX-L0 was clearly shown in the mass spectrum, which confirmed the Pd0 sensing mechanism (Fig. S3‡). In contrast, titrations of other investigated metal ions (M), including Pd2+, revealed no change of the fluorescence spectra after 4 h (Fig. 2c).
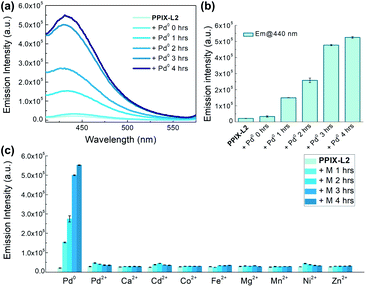 |
| Fig. 2 Fluorescence profile of PPIX-L2 (MeOH, 2 μM, λex = 361 nm) to Pd0. Changes of (a) fluorescence spectra, (b) coumarin fluorescence intensity at 440 nm of PPIX-L2 on Pd0 (10 μM) over time. (c) Metal selectivity. The selectivity of PPIX-L2 to Pd0. [M] = 10 μM. The y-axis is coumarin fluorescence intensity of PPIX-L2 at 440 nm. | |
Having confirmed the responsive increase in coumarin emission of PPIX-L2 towards Pd0, we extended our investigation of PPIX-L2 to Pd2+ (Fig. 3, PdCl2 was used as the source of Pd2+). Addition of Pd2+ resulted in the striking decrease in porphyrin fluorescence of PPIX-L2 and had no effect on the coumarin fluorophore (Fig. 3a). The decrease was determined to be time-dependent (Fig. 3b). The rapid decline of the porphyrin fluorescence up to 81% was seen immediately after the addition of Pd2+, and plateaued at a time point of 1 h, with an almost total quenching of the porphyrin fluorescence presented. A further titration was performed, focusing on changes of porphyrin fluorescence in the first one hour following addition of Pd2+ (Fig. 3c), confirming the fast and efficient detection of Pd2+ by PPIX-L2. Pleasingly, all other metal ions produced no or a much smaller variation of the porphyrin fluorescence of PPIX-L2 (Fig. 3d). As the porphyrin core can act as a cavity for different metal ions, a competition experiment was also performed (Fig. 3e). Pd2+ was shown to be able to displace metal ions from PPIX-L2 in all cases; addition of M followed by Pd2+ to PPIX-L2 led to a rapid and significant decrease of the porphyrin fluorescence. Thus, the porphyrin–coumarin system can serve as a simple and effective system for the discrimination of palladium species.
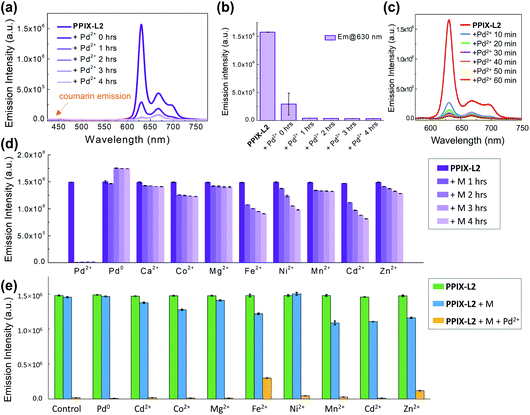 |
| Fig. 3 Fluorescence profile of PPIX-L2 (2 μM, MeOH, λex = 400 nm) to Pd2+. Changes of (a) fluorescence spectra, (b) porphyrin fluorescence intensity at 630 nm of PPIX-L2 on Pd2+ (10 μM) over time. (c) Changes of porphyrin fluorescence spectra of PPIX-L2 within the first one hour of the addition of Pd2+ (10 μM). (d) Metal selectivity and (e) Metal competitively (intensity was measured at 1 h after the addition of M/Pd2+). [M/Pd2+] = 10 μM. The y-axis is fluorescence intensity at 630 nm. | |
Improved detection limit of PPIX-L2 to Pd0 and Pd2+
Having identified PPIX-L2 as a fluorescent differentiator for Pd0 and Pd2+, we then measured its detection limit towards the two palladium species. Correlations between fluorescence of PPIX-L2 and concentrations of Pd0/Pd2+ are demonstrated in Fig. 4. A linear relationship was observed in the concentration range of 0–1 μM at 2 hours after addition of Pd0 and 0–4 μM at 1 hour after addition of Pd2+ (Fig. 4a and b). Using the 3σ method,35 the detection limit towards Pd0 and Pd2+ was determined as 75 nM and 382 nM respectively. Importantly, both values are much lower than the previously reported palladium species differentiators. Furthermore, we investigated the ability of PPIX-L2 to quantify the concentration for Pd0 and Pd2+ in one mixed sample. Therefore, a Pd0 and Pd2+ mixed ion solution ([Pd0] = 0.25 μM, [Pd2+] = 2 μM) was prepared. The emission intensity of PPIX-L2 with the mixed sample was recorded and used for the calculations. The calculated concentration is 0.23 μM and 2.17 μM for Pd0 and Pd2+ respectively (Table S1‡). Both values are having only 8% error to the actual concentration, suggesting PPIX-L2 is able to quantitatively determine the two ions at the same time in one mixed sample solution. Besides, an additional detection limit measurement using the mixed sample solution was also conducted (Fig. S4‡). The detection limit for Pd0 and Pd2+ under this condition was calculated as 97 nM and 390 nM respectively. Although both value increases a little bit compared to that in the single sample, it is still far lower than the previously reported differentiators.
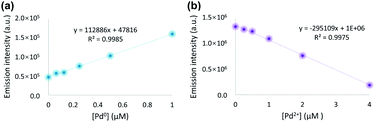 |
| Fig. 4 Fluorescence analysis of PPIX-L2 against various concentrations of Pd0 and Pd2+ in MeOH for the determination of detection limit. (a) Linear correlation between coumarin fluorescence intensity of 5 μM PPIX-L2 at 440 nm and [Pd0]. Fluorescence intensity was recorded at 2 hours after the addition of Pd0 (λex = 361 nm). (b) Linear correlation between porphyrin fluorescence intensity of 2 μM PPIX-L2 at 631 nm and [Pd2+]. Fluorescence intensity was recorded at 1 hour after the addition of Pd2+ (λex = 400 nm). | |
Selectivity mechanism of PPIX-L2 to Pd2+
To study the mechanism by which PPIX-L2 possesses the high selectivity towards Pd2+, a portion-wise titration of Pd2+ with a longer treatment time of 8 h to allow for a complete interaction, was performed and analyzed with the Stern–Volmer equation (Fig. 5).36 The linear relationship between the relative fluorescence intensity of the probe under low concentrations of the quencher (Pd2+), allows an easy graphical determination of Stern–Volmer quenching constant (Ksv), which defines the quenching efficiency. The linear relationship is shown in Fig. 5b, with the determined Ksv as 106 M−1, demonstrating Pd2+ is an effective quencher of the porphyrin fluorescence of PPIX-L2. Double logarithmic of the Stern–Volmer plot further revealed the binding of PPIX-L2 with Pd2+ is extremely strong, with a binding constant (Kb) of 1.41 × 107 M−1 calculated for a 1
:
1 binding stoichiometry. Therefore, the strong binding between Pd2+ and PPIX-L2 is the likely underlying mechanism of the excellent selectivity of PPIX-L2 towards Pd2+ (Fig. 5c). The binding of Pd2+ is likely to occur by coordinating with the four pyrrolic nitrogens in the porphyrin cavity. To corroborate this, an absorption titration with Pd2+ was conducted, given that metal-chelated porphyrin are more symmetrical with the production of a simplified Q band patterns to form only two Q bands (a spectral characteristic of porphyrin coordinated with a metal ion).37 In particular for palladium(II) porphyrins, the two Q bands have been well described to appear at around 525 and 560 nm in the literature.38–40 The absorption titration results are consistent with these descriptions, the four Q bands of PPIX-L2 at 506, 540, 576 and 630 nm gradually diminished with a final appearance of only two Q bands at 519 and 555 nm (Fig. 5d). Furthermore, a MALDI-TOF analysis was performed to confirm the binding of the Pd2+ into the porphyrin cavity of PPIX-L2 to form Pd-PPIX-L2 with expected mass which matches the proposed detecting strategy (Fig. S5‡). These results demonstrate the formation of Pd2+–porphyrin complex, suggesting the fluorescence decrease of PPIX-L2 on Pd2+ is due to the heavy metal effect which leads to the phosphorescence emission.41
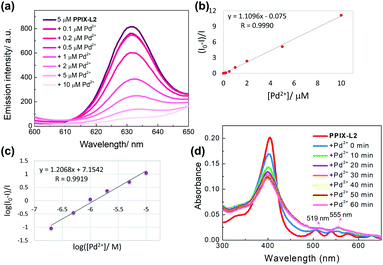 |
| Fig. 5 Study of the interaction details of PPIX-L2 with Pd2+. (a) Fluorescence profile of PPIX-L2 (5 μM) with various concentrations of Pd2+ (treatment time, 8 h). (b) Stern–Volmer analysis and (c) double logarithmic of the Stern–Volmer analysis of the decrease of fluorescence intensity at 631 nm on [Pd2+]. (d) Absorption profile of PPIX-L2 (2 μM) on Pd2+ (10 μM) over time. Measurements were performed in MeOH. | |
Conclusions
In conclusion, we have identified a simple porphyrin–coumarin system PPIX-L2 that can serve as an effective differentiator to discriminate the oxidation states of palladium (Pd0 and Pd2+) through the independent fluorescence changes of coumarin and porphyrin fluorophores, respectively. Importantly, we confirmed that PPIX-L2 can impart not only the independent optical responses towards Pd0 and Pd2+, but also a degree of selectivity. Detection of Pd0 by PPIX-L2 is through the well-studied Tsuji–Trost reaction, therefore, the selectivity mechanism of PPIX-L2 towards Pd2+ was examined in detail. Stern–Volmer analysis of the fluorescence titration results suggested that the strong binding between PPIX-L2 and Pd2+ contributes to the selectivity. Absorption titration experiment and MALDI-TOF mass spectrum further confirms the formation of a Pd2+–porphyrin complex. It is also noteworthy that PPIX-L2 can demonstrate not only different optical responses towards Pd0 and Pd2+, but a much lower detection limit towards these palladium species (75 nM for Pd0, 382 nM for Pd2+) compared to the previously reported palladium differentiators. Crucially, the detection limit of PPIX-L2 towards Pd0 meets the requirement of palladium contamination set by European Agency. From a practical viewpoint, the next step is to further lower the detection limit of PPIX-L2 for Pd2+. Additionally, we are currently investigating the application of PPIX-L2 in pharmaceutical drugs to detect palladium contamination, especially the new chemical entities developed in the pharmaceutical industry. More generally, the results presented here demonstrate a new and simple system that can be exploited as effective palladium differentiators.
Data availability
Further details of the experimental procedures, photophysical measurements, and structural characterization are available in the ESI.
Author contributions
L. J., H. N. M., E. R. H. W., W.-T. W., K.-L. W. and N. J. L. designed the project. L. J. and H. N. M. carried out the synthesis and characterization of all samples. L. J. and H. N. M. performed photophysical measurements. L. J., H. N. M., W.-T. W., K.-L. W. and N. J. L. contributed new reagents and analyzed tools. L. J., H. N. M., W.-T. W., K.-L. W. and N. J. L. analyzed data. L. J., H. N. M., E. R. H. W. and N. J. L. wrote the paper. L. J., H. N. M., E. R. H. W., W.-T. W., K.-L. W. and N. J. L. discussed and commented on the manuscript.
Conflicts of interest
There are no conflicts to declare.
Acknowledgements
N. J. L. gratefully acknowledges the support of a Royal Society Wolfson Research Merit Award. K.-L. W. acknowledges the support from the Dr Mok Man Huang Endowed Professorship in HKBU Chemistry. We thank Dr P.-K. So (PolyU University Research Facility in Life Science) for technical assistance in MALDI-TOF mass spectrum. This work was supported by grants from Hong Kong Research Grant Council (HKBU 12300019).
Notes and references
- A. Biffis, P. Centomo, A. Del Zotto and M. Zecca, Chem. Rev., 2018, 118, 2249–2295 CrossRef CAS
.
- A. F. P. Biajoli, C. S. Schwalm, J. Limberger, T. S. Claudino and A. L. Monteiro, J. Braz. Chem. Soc., 2014, 25, 2186–2214 CAS
.
-
A. O. King and N. Yasuda, in Organometallics in Progress Chemistry, Springer, Berlin, Heidelberg, 2017, pp. 205–245 Search PubMed
.
- A. A. Gewirth, J. A. Varnell and A. M. Diascro, Chem. Rev., 2018, 118, 2313–2339 CrossRef CAS PubMed
.
- G. A. Filonenko, R. Van Putten, E. J. M. Hensen and E. A. Pidko, Chem. Soc. Rev., 2018, 47, 1459–1483 RSC
.
- A. C. Frisch and M. Beller, Angew. Chem., Int. Ed., 2005, 44, 674–688 CrossRef CAS PubMed
.
- Q. Xiao, S. Sarina, A. Bo, J. Jia, H. Liu, D. P. Arnold, Y. Huang, H. Wu and H. Zhu, ACS Catal., 2014, 4, 1725–1734 CrossRef CAS
.
- T. Z. Liu, S. D. Lee and R. S. Bhatnagar, Toxicol. Lett., 1979, 4, 469–473 CrossRef CAS
.
-
C. Melber and I. Mangelsdorf, in Palladium Emissions in the Environment: Analytical Methods, Environmental Assessment and Health Effects, Springer Berlin Heidelberg, 2006, pp. 575–596 Search PubMed
.
-
J. Wataha, in Encyclopedia of Metalloproteins, Springer, New York, 2013, pp. 1628–1635 Search PubMed
.
- J. S. Carey, D. Laffan, C. Thomson and M. T. Williams, Org. Biomol. Chem., 2006, 4, 2337–2347 RSC
.
- C. E. Garrett and K. Prasad, Adv. Synth. Catal., 2004, 346, 889–900 CrossRef CAS
.
- H. Ren, C. A. Strulson, G. Humphrey, R. Xiang, G. Li, D. R. Gauthier and K. M. Maloney, Green Chem., 2017, 19, 4002–4006 RSC
.
- G. J. Stasiuk, F. Minuzzi, M. Sae-Heng, C. Rivas, H.-P. Juretschke, L. Piemonti, P. R. Allegrini, D. Laurent, A. R. Duckworth, A. Beeby, G. A. Rutter and N. J. Long, Chem.–Eur. J., 2015, 21, 5023–5033 CrossRef CAS PubMed
.
- E. R. H. Walter, J. A. G. Williams and D. Parker, Chem.–Eur. J., 2018, 24, 7724–7733 CrossRef CAS PubMed
.
- T. Hirayama, M. Inden, H. Tsuboi, M. Niwa, Y. Uchida, Y. Naka, I. Hozumi and H. Nagasawa, Chem. Sci., 2019, 10, 1514–1521 RSC
.
- M. Denis, J. Pancholi, K. Jobe, M. Watkinson and S. M. Goldup, Angew. Chem., Int. Ed., 2018, 57, 5310–5314 CrossRef CAS
.
- Z. Zhang, G. J. Tizzard, J. A. G. Williams and S. M. Goldup, Chem. Sci., 2020, 11, 1839–1847 RSC
.
- F. Song, A. L. Garner and K. Koide, J. Am. Chem. Soc., 2007, 129, 12354–12355 CrossRef CAS PubMed
.
- A. L. Garner and K. Koide, Chem. Commun., 2009, 86–88 RSC
.
- J. Jiang, H. Jiang, W. Liu, X. Tang, X. Zhou, W. Liu and R. Liu, Org. Lett., 2011, 13, 4922–4925 CrossRef CAS PubMed
.
- S. Pal, M. Mukherjee, B. Sen, S. K. Mandal, S. Lohar, P. Chattopadhyay and K. Dhara, Chem. Commun., 2015, 51, 4410–4413 RSC
.
- W. Feng, D. Liu, S. Feng and G. Feng, Anal. Chem., 2016, 88, 10648–10653 CrossRef CAS
.
- Q. Wang, Q. Chen, C. Li, Q. Lai, F. Zou, F. Liang, G. Jiang and J. Wang, Microchem. J., 2020, 153, 1–5 Search PubMed
.
- L. Lukomski, I. Pohorilets and K. Koide, Org. Process Res. Dev., 2020, 24, 85–95 CrossRef CAS
.
- M. Santra, S. K. Ko, I. Shin and K. H. Ahn, Chem. Commun., 2010, 46, 3964–3966 RSC
.
- M. Wang, Y. Yuan, H. Wang and Z. Qin, Analyst, 2016, 141, 832–835 RSC
.
- A. Kumar Bhanja, S. Mishra, K. Das Saha and C. Sinha, Dalton Trans., 2017, 46, 9245 RSC
.
- A. A. Soares-Paulino, L. Giroldo, N. A. Pradie, J. S. Reis, D. F. Back, A. A. C. Braga, H. A. Stefani, C. Lodeiro and A. A. Dos Santos, Dyes Pigm., 2020, 179, 108355 CrossRef CAS
.
- X. Chen, Q. Ma, Z. Wang, Z. Xie, Y. Song, Y. Ma, Z. Yang and X. Zhao, Asian J. Chem., 2020, 15, 4104–4112 CrossRef CAS
.
- H. Li, J. Fan and X. Peng, Chem. Soc. Rev., 2013, 42, 7943 RSC
.
- A. L. Garner and K. Koide, J. Am. Chem. Soc., 2008, 130, 16472–16473 CrossRef CAS PubMed
.
- L. Rasheed, M. Yousuf, I. S. Youn, G. Shi and K. S. Kim, RSC Adv., 2016, 6, 60546–60549 RSC
.
- G. Hata, K. Takahashi and A. Miyake, J. Chem. Soc., Chem. Commun., 1970, 1392–1393 RSC
.
- Title 40 – Protection of Environment: Code of Federal Regulations.
- M. H. Gehlen, J. Photochem. Photobiol., C, 2020, 42, 100338 CrossRef CAS
.
-
R. Giovannetti, The Use of Spectrophotometry UV-Vis for the Study of Porphyrins, 2012 Search PubMed
.
- Q. X. Wan and Y. Liu, Catal. Lett., 2009, 128, 487–492 CrossRef CAS
.
- S. Drouet, C. O. Paul-Roth, V. Fattori, M. Cocchi and J. A. G. Williams, New J. Chem., 2011, 35, 438–444 RSC
.
- Z. Valicsek and O. Horváth, Microchem. J., 2013, 107, 47–62 CrossRef CAS
.
- L. Zang, H. Zhao, J. Hua, W. Cao, F. Qin, J. Yao, Y. Tian, Y. Zheng and Z. Zhang, J. Mater. Chem. C, 2016, 4, 9581–9587 RSC
.
Footnotes |
† Dedication: For Professor Wolfgang Keim, an outstanding and inspirational scientist, on his 70th birthday. |
‡ Electronic supplementary information (ESI) available: Details of synthesis, chemical structure characterization and photophysical measurement. See DOI: 10.1039/d1sc01616d |
§ Authors with equal contribution. |
|
This journal is © The Royal Society of Chemistry 2021 |